ABSTRACT
Corn fibre xylan (CX) shows high resistance to enzymatic hydrolysis due to its densely decorated side chains. To find enzymes capable of hydrolyzing CX, we isolated a bacterial strain (named H2C) from soil, by enrichment culture using non-starch polysaccharides of corn as the sole carbon source. Analysis based on the 16S rRNA sequence placed strain H2C within genus Paenibacillus. Enzymes were purified from supernatant of culture broth of strain H2C based on solubilizing activities toward CX. Four enzymes, Xyn5A, Xyn10B, Xyn11A, and Xyn30A, were successfully identified, which belong to glycoside hydrolase (GH) families, 5, 10, 11, and 30, respectively. Phylogenetic analysis classified Xyn5A in subfamily 35 of GH family 5, a subfamily of unknown function. Their activities toward beechwood xylan and/or wheat arabinoxylan indicated that these enzymes are β-1,4-xylanases. They showed high solubilizing activities toward a feed material, corn dried distiller’s grains with solubles, compared to five previously characterized xylanases.
Abbreviations : CX: corn fibre xylan; DDGS: corn dried distiller’s grains with solubles
Graphical abstract
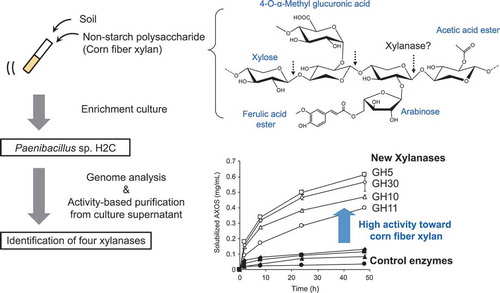
Strain H2C was isolated by enrichment culture using non-starch polysaccharides of corn. The identified enzymes from strain H2C showed high activities toward corn fibre xylan.
The use of “corn distillers dried grains with solubles” (DDGS) in animal feed has recently received extensive attention because of its good nutritional profile including protein, fat, vitamins, and minerals [Citation1,Citation2]. However, previous research suggested that the nutrient availability of DDGS in monogastric animals is limited [Citation3] due to the high concentration of non-starch polysaccharides (NSPs, also known as indigestible fibre), which comprise 25–33% of DDGS [Citation4]. In recent years, much effort has been made to improve the nutritive value of feedstuffs by using exogenous enzymes referred to as NSP-degrading enzymes [Citation5]. These enzymes are added in the feed blending process or sprayed onto pelleted feed, and degrade NSPs passing through the digestive organs of animals after feed intake. Because the primary component of the NSP of DDGS is corn fibre xylan (CX) [Citation4], which shows resistance to enzymatic hydrolysis due to its complex structure, research into enzymes capable of efficient degradation of CX has been a significant challenge in the field of animal feed.
Xylan consists of D-xylose units joined by β-linkages and substituted with side residues. The degree and structures of xylan main chain substitutions are variable and depend on the plant species. In contrast to less-branched xylans like oat spelt xylan and beechwood xylan (BWX), the main chain of CX from the outer layer of seeds is highly substituted with arabinose residues, 4-O- methylglucuronic acid (MeGlcA) residues and acetyl groups. Structural analysis suggested that >70% of the xylose backbone residues of CX have side chains [Citation6–Citation8]. Furthermore, some arabinose residues are esterified with ferulic acids and decorated with xyloses and galactopyranoses [Citation9]. These decorations inhibit recognition of the xylan backbone by xylanase. In addition, feruloylated arabinose residues contribute to xylan cross-linking to form strong heterogeneous intermolecular complexes, further affecting enzymatic degradation [Citation10,Citation11]. In previous studies, CX showed resistance to commercial enzymes compared to the less-branched xylans from oat spelt and beechwood [Citation12,Citation13].
Recent studies have shown some examples of enzymatic hydrolysis of CX. A glycoside hydrolase family 5 (GH5) xylanase (HtGH5) from Hungateiclostridium thermocellum (previously Clostridium thermocellum) was active toward corn bran xylan, and its substrate recognition mechanism was characterized [Citation14]. In addition, a novel endo-acting CX-specific xylanase classified in GH98 was discovered in Bacteroides ovatus. The substrate specificity of the enzyme suggested that some of the CX decorations were the specificity determinants of the enzyme [Citation15]. These studies used alkaline-extracted CX. Alkaline extraction saponifies all ester linkages and thus eliminates acetate and feruloyl groups [Citation16], enabling enzymes to reach to the xylan backbone. However, enzymes for livestock feed need to hydrolyze CX without alkaline extraction, which cannot be used for feed raw material.
Some studies showed enzymatic solubilization of CX without alkaline extraction. In enzymatic degradation of CX isolated by hot water extraction, a mixture of enzymes consisting of α-L-arabinofuranosidases, a xylanase, and a β-xylosidase liberated more xylan when combined with an acetylxylan esterase and/or a feruloyl esterase [Citation17]. Another study showed solubilizing activities of four microbial xylanases toward corn and wheat DDGS. While the amounts of arabino-xylooligosaccharide (AXOS) solubilized from wheat DDGS were similar, those from corn DDGS were different among the enzymes [Citation18]. However, despite the growing interest in feed enzymes for CX degradation, as far as we know, there have been few reports on isolation of enzymes with high hydrolyzing activity toward CX. In this paper, we isolated a bacterial strain, Paenibacillus sp. H2C, by enrichment culture using corn NSP as the sole carbon source and identified four xylanases involved in CX degradation.
Materials and methods
Chemicals
Cellfer (Nihon Shokuhin Kako, Tokyo, Japan) was used as the carbon source for isolation of strain H2C and assay for enzyme purification. According to the manufacturer, this material is produced by a mechanical process from corn hull, and the neutral detergent fibre content of the product is >85%. DDGS was used as corn NSP for enzyme characterization. The pentose and uronic acid contents in Cellfer and the DDGS measured as described below are listed in . BWX (MeGlcA/Xylose molar ratio = 0.11) and insoluble wheat arabinoxylan (WAX, Arabinose/Xylose molar ratio = 0.71) were purchased from Megazyme (Wicklow, Ireland) and oat spelt xylan was purchased from Sigma-Aldrich (St. Louis, MO). GH10 xylanase from Aeromonas punctata [Citation19] and GH11 xylanase from Trichoderma longibrachiatum [Citation20] were purchased from Megazyme.
Table 1. Sugar composition (wt%) of substrate derived from corn.
Isolation and identification of strain H2C
More than 50 soil samples were collected and suspended in distilled water. Each supernatant of the soil suspension was inoculated into test tubes containing 2 mL enrichment medium (1 g/L NH4NO3, 1 g/L K2HPO4, 1.3 g/L NaH2PO4•2H2O, 200 mg/L MgSO4•7H2O, 10 mg/L FeSO4•7H2O, 10 mg/L MnSO4•7H2O, 10 mg/L ZnSO4•7H2O, 10 mg/L CaCl2•2H2O, and 2 g/L Cellfer, pH 6.7). The culture was incubated in a water bath shaker at 160 rpm at room temperature. After 2 weeks, the culture broth from test tubes with reduced turbidity of Cellfer was applied to agar plates containing enrichment medium and grown at room temperature for 1 week. Grown colonies were suspended and diluted in distilled water, and then inoculated onto agar plates containing enrichment medium with 0.5% oat spelt xylan instead of Cellfer. A strain with clear zones around the colonies was selected for further analysis.
Phenotypic characterization was performed according to the method for bacterial identification reported by Barrow & Feltham [Citation21]. Strain H2C was aerobically grown at 37°C for 24 h in Luria-Bertani (LB) medium for phenotypic characterization. A 16S rRNA gene sequence was obtained from the genome sequence. EzTaxon [Citation22] was used as the database for 16S rRNA sequences of type strains.
Genome sequencing, assembly, and prediction
Strain H2C was grown at 30°C in 50 mL of LB medium for 2 days. Genomic DNA was extracted using a Genomic-tip 100/G (Qiagen, Hilden, Germany). A Nextera DNA Sample Prep Kit (Illumina, Little Chesterford, UK) was used following the manufacturer’s instructions to prepare the genomic library. We used the MiSeq v2 system (Illumina) to generate 22.8 million paired-end reads with a read length of 150 bp (average sequencing depth: 35.5). Subsequently, we obtained 109 contigs (total contig length: 6,988,404 bp) with Velvet [Citation23]. From the sequences, 6,128 protein-coding genes were predicted with the GenLook program using default conditions [Citation24].
Production of enzymes from strain H2C
One milliliter of culture broth of strain H2C was inoculated into Sakaguchi flasks each containing 100 mL of LB medium with 0.2% (w/v) Cellfer, and cultured at 30°C and 120 rpm for 48 h. The whole broth was centrifuged at 29,100 × g for 10 min, and the resulting supernatant was filtered through a 0.22-μm polyethersulfone membrane (Merck Millipore, Billerica, MA) in sterile conditions. The culture filtrate containing NSP-degrading enzymes was stored at 4°C.
Identification of enzymes by column chromatography
Enzyme purification was carried out using an ÄKTA Avant chromatography system (GE Healthcare, Uppsala, Sweden) at 4°C. Solid (NH4)2SO4 was added to culture supernatant to produce to a final concentration of 1.0 M. Following centrifugation, the supernatant was applied to hydrophobic interaction chromatography using a HiLoad 16/10 Phenyl Sepharose column (GE Healthcare), eluted with 10 column volumes (CV) of a 1.0 to 0 M (NH4)2SO4 gradient in 50 mM Tris-HCl buffer, pH 7.0 at a flow rate of 5 mL/min. Two activity peaks were observed in the chromatogram ()), and the fractions from each peak were pooled and desalted by ultrafiltration using Amicon centrifugal filter units (10 kDa cutoff; Merck Millipore).
Figure 1. Purification of corn fibre xylan solubilizing enzymes from culture broth of strain H2C.
(a) Relative activity of each fraction obtained by hydrophobic interaction chromatography. The activities were determined by quantification of solubilized AXOS from Cellfer after reaction. The value of the highest activity was defined as 100%. The fractions pooled and subjected to subsequent purification steps are indicated as fr.1 and fr. 2. (b) SDS-PAGE of purified fractions. Lane M: molecular mass standards; lanes 1A, 1B, and 2A: purified fractions. The SDS-PAGE gels were stained with SYPRO Ruby protein gel stain (Thermo Fisher Scientific).
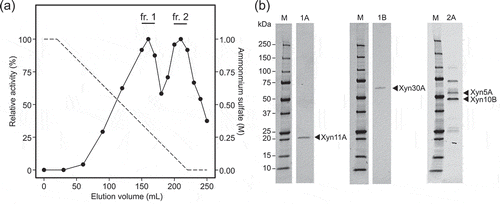
Fraction 1, which included Xyn11A and Xyn30A, was applied to cation-exchange chromatography using a HiTrap SP HP, 5 mL column (GE Healthcare), which was eluted with 10 CV of a 0 to 1.0 M NaCl gradient in 50 mM 2-(N-morpholino)ethanesulfonic acid buffer, pH 6.0 at a flow rate of 5 mL/min. The activity eluted from HiTrap SP was detected both in bound and flow-through fractions. The bound fraction, corresponding to Xyn11A, was collected and named fraction 1A. The flow-through fraction was desalted, and then applied to anion-exchange chromatography using a HiTrap Q HP 5-mL column (GE Healthcare), eluted with 10 CV of a 0 to 1.0 M NaCl gradient in 20 mM Tris-HCl buffer, pH 8.0 at a flow rate of 5 mL/min. The activity eluted from the HiTrap Q column was detected in the flow-through fraction, which was then concentrated and subjected to gel filtration using a HiLoad 16/600 Superdex 200pg column (GE Healthcare, 1.6 × 30 cm) with 100 mM NaCl in 20 mM Tris-HCl buffer, pH 7.0 at a flow rate of 1.0 mL/min. The active fraction, corresponding to Xyn30A, was collected and named fraction 1B.
Fraction 2, which included Xyn5A and Xyn10B, was applied to anion-exchange chromatography using a RESOURCE Q, 6 mL column (GE Healthcare), eluted with 10 CV of a 0 to 1.0 M NaCl gradient in 20 mM Tris-HCl buffer, pH 8.0 at a flow rate of 6 mL/min. The activity was detected in the flow-through fraction, which was then concentrated and stored as fraction 2A.
Fractions 1A, 1B, and 2A were applied to SDS-PAGE [Citation25] and electroblotted onto polyvinylidene difluoride membranes using an iBlot Dry Blotting system (Thermo Fisher Scientific, Waltham, MA). The membrane was stained with Coomassie Brilliant Blue R-250, and the visualized protein bands were cut out. N-terminal amino acid sequencing of each protein band was performed by automated repetitive Edman degradation on a model 494 Procise Protein Sequencer (Applied Biosystems, Foster City, CA). Full-length protein sequences were obtained by comparison of the N-terminal sequences and genome sequence.
Structure and phylogenetic analysis of xylanases
InterPro [Citation26] and dbCAN, a database for carbohydrate-active enzymes [Citation27] were used for determination of the module composition of identified proteins. For phylogenetic analysis, the amino acid sequences of GH5 modules from biochemically characterized GH5_21 proteins and all GH5_35 proteins in the Carbohydrate-Active Enzymes database (CAZy; http://www.cazy.org) database were aligned using MUSCLE [Citation28]. A phylogenetic tree was constructed by the maximum-likelihood method based on the Whelan and Goldman model algorithm [Citation29] in MEGA7 [Citation30]. A discrete Gamma distribution was used to model evolutionary rate differences among sites (five categories). A total of 100 bootstrap replicates were performed.
Enzyme assay for purification steps
For enzyme purification, solubilizing activities of the fractions from strain H2C were determined as described in the literature [Citation13] with some modifications. First, 500 mg of Cellfer was suspended in 16 mL of 100 mM sodium acetate buffer at pH 5.0 and 0.4 mL were dispensed into microtubes. Subsequently, 0.1 mL of material to be assayed was added to each tube, and thus the final concentration of substrate was 2.5% (w/v). Blanks were prepared using 0.1 mL of buffer. All samples were incubated at 40°C for 2 h with continuous mixing by a microtube rotator (MTR103; AS ONE, Osaka, Japan) and then plunged into a heat block (DTU-1B; AS ONE) at 100°C for 5 min to stop the reaction. The samples were then cooled on ice and the supernatants were recovered after centrifugation at 13,800 × g for 5 min at 4°C. AXOS in the supernatants was quantified as total pentose sugars colorimetrically by reaction with phloroglucinol upon heating in acetic–hydrochloric acid as described in a previous study [Citation13], except that the volume of the supernatant and reagent were 0.1 mL and 0.5 mL, respectively.
Preparation of recombinant enzymes
The genes encoding mature forms of Xyn5A, Xyn10B, Xyn11A, and Xyn30A from strain H2C, as well as a GH11 xylanase (accession number: CAB13776.1) from Bacillus subtilis 168 [Citation31], were amplified by PCR using genomic DNA as the template. The amplified products were cloned into the NdeI/XhoI sites of vector pET21b (Merck Millipore) except for Xyn5A, which was cloned into the BamHI/HindIII sites of pET-Duet1 (Merck Millipore), using an In-Fusion HD Cloning Kit (Clontech, Palo alto, CA). Plasmids were propagated in Escherichia coli JM109 (Takara Bio, Shiga, Japan). All the primers used in PCRs are listed in . Expression plasmids for the N-terminus of GH5 xylanase from H. thermocellum (accession number: ABN53395.1, residues 21–885, lacking the DOK module) [Citation32] and the full length of GH98 xylanase from B. ovatus (BACOVA_03433) [Citation15] were constructed using the same vector and the restriction sites described in the original study. Their coding sequence was initially generated by gene synthesis (Eurofins Genomics, Tokyo, Japan) and cloned into the NheI/XhoI or BamHI/HindIII sites of pET21a (Merck Millipore). All constructs contain C-terminal His6-tag derived from the cloning vector except for Xyn5A, which contains N-terminal His6-tag. All expression plasmids were transformed into E. coli BL21 (DE3) (Invitrogen). The recombinant strains were grown in 100 mL of Overnight Express Instant TB Medium (Merck Millipore) supplemented with 100 µg/L ampicillin at 30°C for 18 h in 500-mL Sakaguchi flasks, except for the strains expressing Xyn10B, Xyn11A, and GH98 xylanase from B. ovatus which were grown at 18°C for 40 h. Cells were harvested by centrifugation, lysed with xTractor buffer (Clontech), and loaded onto HisTALON cobalt columns (Clontech). The column was washed with 20 mM Tris-HCl buffer (pH 7.6) containing 300 mM NaCl and 10 mM imidazole, and eluted using the same buffer containing 150 mM imidazole. Eluted fractions were pooled, and buffer was exchanged to 50 mM Britton-Robinson (BR) buffer at pH 6.0 using Amicon centrifugal filter units (Merck Millipore). Protein purity was assessed by SDS–PAGE and protein concentration was estimated by the Lowry method [Citation33] using bovine serum albumin as a standard.
Table 2. Primers used for cloning.
Determination of specific activity toward BWX and WAX
The specific activities toward BWX and WAX were determined based on molar concentrations of newly formed reducing ends by enzymatic reaction. First, enzymatic hydrolysis was performed in a 0.1-mL final volume containing 1.5% (w/v) BWX or WAX and diluted enzyme solution, in 50 mM BR buffer at pH 6.0. All reactions were carried out at 37°C for 10 min, except for H. thermocellum GH5, which was assayed at 60°C. At the end of the reaction, 0.1 mL of 0.1 M NaOH was added to the reaction mixture to terminate the reaction, and the supernatant was recovered after centrifugation at 13,800 × g for 10 min. The amount of reducing sugar liberated was determined using the 3,5-dinitrosalicylic acid method and xylose as the standard [Citation34]. One unit of hydrolyzing activity was defined as the amount of the enzyme that formed reducing sugar ends equivalent to 1 µmol of xylose per min in the assay conditions.
Enzymatic hydrolysis of DDGS
Enzyme activities toward CX were assessed as the amount of solubilized AXOSs from DDGS after hydrolysis reactions. DDGS was ground through a 0.50-mm sieve and washed in warm distilled water to remove soluble components. The enzymatic hydrolysis was performed in a 0.5-mL final volume containing 2.5% (w/v) DDGS and 1 µg/mL of enzyme, in 50 mM BR buffer at pH 6.0. Samples were incubated at 37°C for 2, 8, 24, and 48 h with continuous mixing by a rotator, except for H. thermocellum GH5 which was incubated at 60°C. Samples were plunged into a heat block at 100°C for 2 min to stop the reaction. The samples were then cooled on ice and the soluble fraction recovered using a 0.22-μm Ultrafree-MC low-binding Durapore centrifugal filter (Merck Millipore). After hydrolysis of AXOS in the soluble fraction, the concentrations of pentose (arabinose and xylose) were measured as described below.
Assessment of combinatorial effect on DDGS
For assessment of combinatorial effects of xylanases, 10 µg/mL of a single enzyme or a mixture of 5 µg/mL each of two xylanases, for a total of 10 µg/mL of enzyme, was incubated with 2.5% (w/v) DDGS. After incubation at 37°C and pH 6.0 for 5 h, the soluble fraction was recovered and the concentration of AXOS was analyzed as described below. The protein concentration in the soluble fraction was estimated by the Lowry method. For the comparison of means of released AXOS and protein, one-way analysis of variance was applied followed by a Tukey honestly significant difference test with overall significance level P = 0.05, using R statistical software (The R Foundation for Statistical Computing, version 3.4.1).
Analysis of sugar concentration
Cellfer, ground DDGS, or the soluble fraction from enzymatic reaction solution were hydrolyzed to monosaccharides in 0.4 M sulfuric acid for 1 h at 125°C in an autoclave and analyzed by high-performance anion exchange chromatography–pulsed amperometric detection (ICS-5000+ system; Thermo Fisher Scientific) equipped with a CarboPac SA10–4 μm (2 × 250 mm) column and a CarboPac SA10–4 μm (2 × 50 mm) column. The analysis was performed at a column temperature of 40°C with an injection volume of 5 μL, a flow rate of 0.38 mL/min, and the mobile phase consisted of 1 mM KOH. The degradation products were identified and quantified by comparing to xylose and arabinose standards. The amount of MeGlcA in the substrate after acid hydrolysis was estimated by a colorimetric method for uronic acids [Citation35] using glucuronic acid as the standard.
Accession numbers
The nucleotide sequences of 16S rRNA and xylanase genes of strain H2C have been deposited in DDBJ/EMBL/GenBank with following accession numbers: 16S rRNA, LC464697; Xyn5A, LC464693; Xyn10B, LC464694; Xyn11A, LC464695; and Xyn30A, LC464696. Strain H2C has been deposited with the National Institute of Technology and Evaluation, Patent Microorganisms Depositary (Chiba, Japan) as BP-02241.
Results
Isolation and identification of Paenibacillus sp. H2C
Strain H2C showed growth in medium containing Cellfer, commercially available NSPs from corn hull, as the sole carbon source. The 16S rRNA sequence of strain H2C is 1,474 bp long, and its most closely related type strains are Paenibacillus ginsengiterrae DCY89T (99.0% identity of the 16S rRNA sequence) [Citation36] and P. cellulosilyticus PALXIL08T (98.4% identity) [Citation37]. Strain H2C cells are Gram-positive, aerobic, rod-shaped, motile, and spore-forming, typical characteristics of the genus Paenibacillus. On the basis of the phylogenetic, chemotaxonomic and phenotypic data, we classified strain H2C as a species of the genus Paenibacillus [Citation38].
Isolation of corn arabinoxylan-degrading enzymes
Genome analysis of Paenibacillus sp. H2C identified >100 putative genes coding for glycoside hydrolases, including nine and one gene(s) encoding glycoside hydrolase family 10 and 11 enzymes, respectively, the families to which xylanases mainly belong (data not shown). Thus, to identify the specific enzymes involved in corn NSP degradation, we purified enzymes from culture supernatant based on AXOS release activity from Cellfer, measured by colorimetric assay using phloroglucinol. The activities were divided into two fractions (fractions 1 and 2) in the first chromatography step ()), and following steps divided fraction 1 into two subfractions (fractions 1A and 1B) and further purified fraction 2 into fraction 2A. Fractions 1A and 1B each gave a single band in SDS-PAGE, at 20 and 55 kDa respectively ()). Fraction 2A contained partially-purified proteins and gave three major bands on SDS-PAGE, at 45, 55, and 70 kDa respectively. The N-terminal sequences of the five bands were determined and found in the amino acid sequences predicted from the strain H2C genome (). The estimated sizes obtained on SDS-PAGE corresponded to the theoretical molecular masses of the respective proteins based on amino acid sequences. Sequence comparison to proteins in the databases, InterPro and dbCAN, revealed that the four enzymes contain catalytic modules belonging to glycoside hydrolase families 5, 10, 11, and 30 (GH5, GH10, GH11, and GH30) respectively. Therefore, we designated the enzymes Xyn5A, Xyn10B, Xyn11A, and Xyn30A, respectively. Because the 70-kDa band in fraction 2A did not show similarity to glycoside hydrolases, we did not investigate it further.
Table 3. Identification of proteins in active fractions.
Structures of identified enzymes
All four enzymes have N-terminal signal peptides, and Xyn5A, Xyn10B, and Xyn30A are predicted to be modular enzymes composed of one GH catalytic module and one carbohydrate-binding module (CBM) ().
Figure 2. Module organizations of Paenibacillus sp. H2C xylanases Xyn5A, Xyn10B, Xyn11A, and Xyn30A.
The size of each enzyme and module is scaled according to the polypeptide chain length. GH, glycoside hydrolase family; CBM, carbohydrate binding module family. a.a., amino acids.
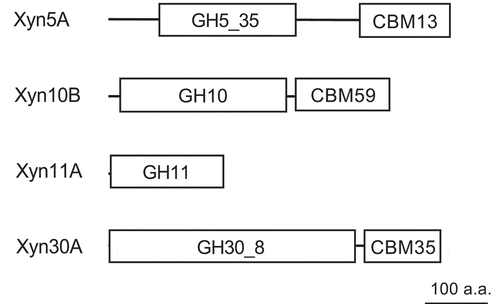
Xyn5A did not show significant similarity with any characterized enzyme. Family GH5 has been subdivided into 51 subfamilies based on sequence similarity [Citation39], and currently contains 12,613 members according to the CAZy. Xyn5A shows similarity to proteins belonging to subfamily 35 of family GH5 (GH5_35) () and shows the highest similarity to GH5_35 putative endo-glucanase from Paenibacillus sp. BIHB4019 (83.5% identity). Therefore, we classified Xyn5A as a member of GH5_35. The C-terminal region of Xyn5A shows sequence similarity to a ricin-type beta-trefoil lectin domain-like domain (RicinB_lectin_2, pfam14200). CAZy lists this domain as CBM family 13 (CBM13). CBM13 is reported to have high affinity for xylan, but not polymers of other β-1,4-linked sugars [Citation40].
Figure 3. Phylogenetic tree of GH5_35 and GH5_21 enzymes based on maximum likelihood with 100 bootstrap replications.
Xyn5A from Paenibacillus sp. H2C is marked with a star. Bootstrap values <50 are not shown.
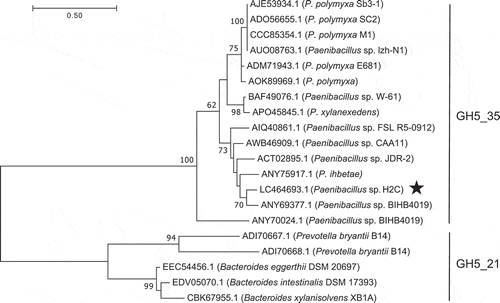
The GH10 catalytic module of Xyn10B shows similarity to the biochemically characterized XynA from Xanthomonas axonopodis pv. citri [Citation41] (44.0% identity). Xyn10B has a CBM59. The CBM59 of Xyn10B shows similarity to CBM59 of mannanase from Bacillus sp. N16–5 [Citation42] and Bacillus sp. JAMB-602 [Citation43] (41.8% and 40.6% identities, respectively). Previous study demonstrated that the CBM59 of ManF-X10, a GH10 xylanase from an environmental genomic DNA library, shows binding affinity to mannan, xylan, and cellulose [Citation44].
Xyn30A has a CBM35. The modular structure of the enzyme, composed of a GH30 catalytic module and CBM35, is identical to that of the biochemically-characterized GH30 xylanase Xyn30D from Paenibacillus barcinonensis [Citation45,Citation46] (79.1% identity). The previous study shows binding affinity of Xyn30D to insoluble oat spelt xylan. However, the deletion of the CBM35 does not affect the specific activity of Xyn30D toward assayed xylans [Citation45].
Xyn11A is a single-module protein and is similar to the biochemically characterized XynA from B. subtilis [Citation47] (90% identity).
Activities toward polysaccharides
Specific activities of recombinant Xyn5A, Xyn10B, Xyn11A, and Xyn30A are shown in . The activities toward BWX and insoluble WAX were assayed by measuring the amount of reducing sugar released from xylan using 3,5-dinitrosalicylic acid reagent. Activities of Xyn5A and Xyn30A were detectable only toward WAX and BWX, respectively, while Xyn10B and Xyn11A were active toward both substrates. For comparison, HtGH5 and B. ovatus GH98 xylanase, in which the activities on alkali-extracted CX were previously reported [Citation14,Citation15], were measured. Aeromonas punctata GH10 xylanase [Citation19], Trichoderma longibrachiatum GH11 xylanase [Citation20], and Bacillus subtilis GH11 xylanase [Citation31] were also measured because their optimum conditions for activity (around neutral pH and 40°C) were similar to the intestinal condition of monogastric animals. There was no remarkable difference between the enzymes of strain H2C and the five control enzymes in the activities toward BWX and WAX.
Table 4. Degradation activities toward BWX, WAX, and DDGS.
To evaluate the enzyme activities in conditions simulating passing time in the intestine of monogastric animals, we compared the amount of pentosans released from DDGS by each enzyme for 2 to 48 h (). In contrast to the results against BWX and WAX, the enzymes of strain H2C showed higher activities toward DDGS than the control enzymes. Especially, Xyn5A, Xyn10B, and Xyn30A had high activities, solubilizing 5.1–6.8 times more AXOS after incubation for 24 h than the GH11 xylanase from B. subtills, which solubilized the highest amount of AXOS among the control enzymes ().
Figure 4. Time course of hydrolysis of corn dried distiller’s grains with solubles (DDGS) by 1 µg/mL of enzymes.
Purified enzymes were incubated with 2.5% DDGS in 50 mM Britton-Robinson buffer, pH 6.0. Concentrations of AXOS in soluble fraction was measured. Error bars represent SDs for duplicate reactions, each measured once.
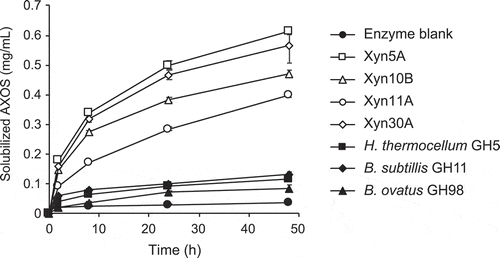
The ratio of arabinose to xylose in released AXOS (the A:X ratio) was also estimated (). The A:X ratio was higher for Xyn5A and Xyn30A than for Xyn10B, indicating that the branching frequency was different in the AXOS released by each enzyme.
Table 5. Arabinose to xylose ratio of AXOS solubilized from DDGS.
Improvement of the degradation of CX by using mixtures of enzymes
The combinatorial effects of recombinant Xyn5A, Xyn10B, and Xyn30A on degradation of DDGS were investigated (). In addition to released AXOS, the amount of released protein, which is related to improvement of nutrient availability of feed, was also measured. In this experiment, the total concentration of enzyme in the assay solution was equivalent in each condition (10 µg/mL). The mixture of Xyn5A and Xyn10B released higher amount of AXOS than each enzyme alone, indicating that the solubilizing efficiency of DDGS per enzyme weight was improved in the enzyme mixture. Similarly, the combination of Xyn10B and Xyn30A liberated more AXOS than each enzyme alone. This combinatorial effect was also observed for released protein. Although Xyn11A showed low activity toward DDGS compared to other enzymes from strain H2C (), it showed the combinatorial effect with Xyn30A in the assay at lower enzyme concentration (1 µg/mL) (data not shown). Since the pentose content in insoluble fraction of DDGS measured after hydrolysis was 11.7% (), the CX concentration in the assay solution was 2.9 mg/mL as pentose equivalent. The concentration of AXOS solubilized by incubation with Xyn5A and Xyn10B was 0.51 mg/mL, which corresponds to 18% of the pentose concentration in DDGS.
Figure 5. Combinatorial effects of xylanases on release of AXOS (a) and proteins (b) from corn dried distiller’s grains with solubles (DDGS).
Ten µg/mL of a single enzyme or a mixture of 5 µg/mL each of two xylanases, for a total of 10 µg/mL of enzyme, was incubated with 2.5% (w/v) DDGS in 50 mM Britton-Robinson buffer, pH 6.0 for 5 h. The concentration of solubilized AXOS and protein were calculated by subtracting the value of the enzyme blank. Error bars represent SDs for triplicate reactions, each measured once. Bars with identical letters indicate no significant difference from one another by the Tukey test at the 5% probability level.
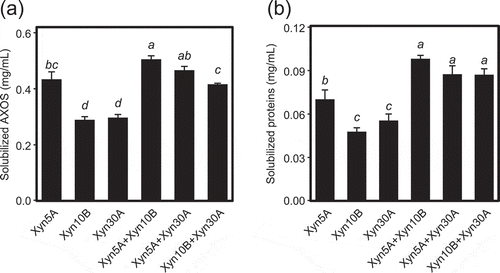
Discussion
CX is a typical example of xylan resistant to the action of xylanases because of its densely substituted main chain. In this study, we found a bacterial strain capable of growing on corn NSP and identified enzymes involved in CX degradation. The enzymes showed high solubilizing activities toward CX without alkaline extraction compared to previously characterized enzymes, although their hydrolytic activities toward WAX and BWX were similar to those of other enzymes ().
Since strain H2C has many glycoside hydrolases, genomic information was not sufficient to identify the enzymes capable of hydrolyzing CX. Therefore, to identify enzymes involved in CX degradation, we purified enzymes from culture supernatant based on CX-solubilizing activity. This approach led to the isolation of Xyn5A, which showed the highest activity toward DDGS among the isolated enzymes.
The enzymes isolated here showed different substrate preferences toward BWX and WAX (). Xyn5A showed high activity toward WAX, xylan containing arabinose side chain. The GH5 subfamily 21 (GH5_21) xylanase from Prevotella buryantii and Bacteroides strains showed hydrolytic activities toward arabinoxylan and xylohexaose [Citation48]. The GH5 subfamily 34 (GH5_34) xylanase from H. thermocellum targets arabinose-branched regions. In contrast, Xyn30A showed high activity toward BWX, xylan containing MeGlcA side chain. GH30 xylanases were reported to target MeGlcA branched regions of xylans [Citation49]. The substrate preference of Xyn5A and Xyn30A were consistent with previous research, suggesting that they also require the side chain in their catalytic site for substrate recognition. Previous research showed substrate recognition in GH10 and GH11 xylanases are inhibited by side chains of specific positions [Citation50]. GH10 requires two unsubstituted consecutive xylose residues to attack the main chain of glucuronoxylan, and one unsubstituted xylose residue to attack that of arabinoxylan. GH11 requires three unsubstituted consecutive xylose residues to hydrolyze both xylans. The substrate specificities of Xyn10B and Xyn11A, which could hydrolyze both WAX and BWX, were consistent with those of reported GH10 and GH11 xylanases [Citation50], suggesting that the substrate recognition of Xyn10B and Xyn11A are also inhibited by the side chains in their catalytic sites.
Mixtures of different enzymes liberated more AXOS and protein than single enzymes (). Based on the recognition mechanisms deduced above, Xyn5A and Xyn30A are suggested to hydrolyze highly branched structures and Xyn10B to hydrolyze less branched structures. Differences in the A:X ratio of AXOS () released by assayed enzymes may reflect their mechanisms of substrate recognition. The mechanism of the improvement of solubilization efficiency by enzyme combination might be that each enzyme preferentially hydrolyzes different positions of glycosidic linkages in the xylan main chain of CX, correspondingly increasing the ratio of hydrolyzable positions. Agger et al. reported enzymatic hydrolysis of pretreated corn bran (190°C, 10 min) using a mixture of α-L-arabinofuranosidases, an endo-xylanase, and a β-xylosidase [Citation17]. They showed that the addition of acetylxylan esterase to the enzyme mixture almost doubled the xylose release from the insoluble substrate fraction. Our results revealed that combinations of xylanases with different substrate recognition mechanisms were also effective in increasing the efficiency of hydrolysis of CX.
The beneficial effects of NSP-degrading enzymes to corn-based diet are attributable to two mechanisms 1) releasing the encapsulated nutrients by disruption of the cell wall structure and 2) prebiotic effects by the released oligosaccharides [Citation5,Citation51]. This study demonstrated that the addition of the identified enzymes improved the protein release and solubilized 18% of AXOS contained in DDGS, suggesting that they have the potential to be applied to feed enzymes for corn-based diets.
Xyn5A showed higher activity toward DDGS than HtGH5. Labourel et al. reported the hydrolysis activity of HtGH5 toward CX and the crystal structure of the enzyme [Citation14]. The crystal structure in complex with a pentasaccharide suggested that the hydroxyls of xylose residues distal to the active site are solvent exposed, suggesting how the enzyme accesses CX with highly decorated side residues. However, the activity of HtGH5 toward DDGS was lower than that of the xylanases from strain H2C. It is still unclear whether the high DDGS-degrading activity of Xyn5A compared to HtGH5 is due to the GH5_35 module or CBM13. GH5_35 contains 14 members, all of which are from Paenibacillus spp., but they have not been characterized. This study newly identified the activity of GH5_35 as xylanase. Among the subfamilies of GH5, GH5_21 and GH5_34 have been described as xylanases acting on arabinoxylan [Citation39]. Because GH5_21 and GH5_34 are not closely related, their acquisition of xylanase activity is likely to be the result of convergent evolution. Based on phylogenetic analysis of GH5 enzymes, the GH5_35 enzymes are most closely related to the xylanases in GH5_21. The GH5_21 enzyme from Bacteroides xylanisolvens XBA1 displays activity toward alkaline-extracted CX [Citation15], suggesting that the GH5_35 module of Xyn5A may also have activity toward CX.
Xyn5A, Xyn10B, and Xyn30A are modular enzymes, consisting of a catalytic module and a CBM. CBMs in polysaccharide degrading enzymes are defined as protein modules recognizing sugars. Because most of CX in DDGS is insoluble, the enzymes have to bind to the surface of the substrate and react at interfaces between water and CX. Therefore, the CBMs of the three enzymes may contribute to efficient degradation of DDGS by promoting the association of the enzymes with the substrate. In contrast, although Xyn11A has a single-module structure, it showed higher activity toward DDGS than GH11 xylanase from Bacillus subtilis (), which has 90% sequence identity to Xyn11A. This finding suggests that differences in the structures of the catalytic modules contribute to the high degradation activity of Xyn11A toward DDGS. Future work should focus on the contribution of each catalytic module and CBM to CX degradation to understand the enzyme properties required for the efficient degradation of CX.
In conclusion, we isolated a novel strain by enrichment culture using corn NSP as the sole carbon source and identified four enzymes capable of degrading CX by activity-based purification. The enzymes showed high activities toward xylan in DDGS and combinatorial effects in release of AXOS and protein. These high activities make the enzymes attractive candidates for applications as feed enzymes.
Author contribution
All authors designed the experiments and discussed the data. YH, KS, RS, and UB performed the experiments. YH wrote the draft of manuscript. RY and SN reviewed and edited the manuscript.
Acknowledgments
We are grateful to K. Nakagawa for providing DDGS Sample and K. Maki, Y. Akadomari, M. Mizuguchi, and K. Takei for their technical assistance. We thank James Allen, DPhil, from Edanz Group (www.edanzediting.com/ac) for editing a draft of this manuscript. This research received no specific grant from any funding agency in the public, commercial, or not-for-profit sectors.
Disclosure statement
YH, YM, RY, and SN are co-inventors on a pending patent on the enzymes described in this study.
References
- Widyaratne GP, Zijlstra RT. Nutritional value of wheat and corn distiller’s dried grain with solubles: digestibility and digestible contents of energy, amino acids and phosphorus, nutrient excretion and growth performance of grower-finisher pigs. Can J Anim Sci. 2007;87:103–114.
- Stein HH, Shurson GC. Boad-invited review: the use and application of distillers dried grains with solubles in swine diets. J Anim Sci. 2009;87:1292–1303.
- Cowieson AJ, Hruby M, Pierson EEM. Evolving enzyme technology: impact on commercial poultry nutrition. Nutr Res Rev. 2006;19:90–103.
- Pedersen MB, Dalsgaard S, Knudsen KEB, et al. Compositional profile and variation of distillers dried grains with solubles from various origins with focus on non-starch polysaccharides. Anim Feed Sci Technol. 2014;197:130–141.
- Slominski B. Recent advances in research on enzymes for poultry diets. Poult Sci. 2011;90:2013–2023.
- Nghiem NP, Montanti J, Johnston DB, et al. Fractionation of corn fiber treated by soaking in aqueous ammonia (SAA) for isolation of hemicellulose B and production of C5 sugars by enzyme hydrolysis. Appl Biochem Biotechnol. 2011;164:1390–1404.
- Saha BC. Hemicellulose bioconversion. J Ind Microbiol Biotechnol. 2003;30:279–291.
- Saulnier L, Vigouroux J, Thibault J-F. Isolation and partial characterization of feruloylated oligosaccharides from maize bran. Carbohydr Res. 1995;272:241–253.
- Saulnier L, Marot C, Chanliaud E, et al. Cell wall polysaccharide interactions in maize bran. Carbohydr Polym. 1995;26:279–287.
- Saulnier L, Thibault JF. Ferulic acid and diferulic acids as components of sugar-beet pectins and maize bran heteroxylans. J Sci Food Agric. 1999;79:396–402.
- Bunzel M, Ralph J, Brüning P, et al. Structural identification of dehydrotriferulic and dehydrotetraferulic acids isolated from insoluble maize bran fiber. J Agric Food Chem. 2006;54:6409–6418.
- Hespell RB, O’bryan PJ, Moniruzzaman M, et al. Hydrolysis by commercial enzyme mixtures of AFEX-treated corn fiber and isolated xylans. Appl Biochem Biotechnol. 1997;62:87–97.
- Rose DJ, Inglett GE. A method for the determination of soluble arabinoxylan released from insoluble substrates by xylanases. Food Anal Methods. 2011;4:66–72.
- Labourel A, Crouch LI, JLA B, et al. The mechanism by which arabinoxylanases can recognize highly decorated xylans. J Biol Chem. 2016;291:22149–22159.
- Rogowski A, Briggs JA, Mortimer JC, Tryfona T, Terrapon N, Lowe EC, Baslé A, Morland C, Day AM, Zheng H, Rogers TE, Thompson P, Hawkins AR, Yadav MP, Henrissat B, Martens EC, Dupree P, Gilbert HJ, Bolam DN. Glycan complexity dictates microbial resource allocation in the large intestine. Nat Commun. 2015;6:7481.
- Biely P, Singh S, Puchart V. Towards enzymatic breakdown of complex plant xylan structures: state of the art. Biotechnol Adv. 2016;34:1260–1274.
- Agger J, Viksø-Nielsen A, Meyer AS. Enzymatic xylose release from pretreated corn bran arabinoxylan: differential effects of deacetylation and deferuloylation on insoluble and soluble substrate fractions. J Agric Food Chem. 2010;58:6141–6148.
- Pedersen MB, Dalsgaard S, Arent S, et al. Xylanase and protease increase solubilization of non-starch polysaccharides and nutrient release of corn- and wheat distillers dried grains with solubles. Biochem Eng J. 2015;98:99–106.
- Usui K, Suzuki T, Akisaka T, et al. A cytoplasmic xylanase (XynX) of Aeromonas caviae ME-1 is released from the cytoplasm to the periplasm by osmotic downshock. J Biosci Bioeng. 2003;95:488–495.
- Mangan D, Cornaggia C, Liadova A, et al. Novel substrates for the automated and manual assay of endo-1,4-β-xylanase. Carbohydr Res. 2017;445:14–22.
- Barrow GI, Feltham RKA. Cowan and Steel’s manual for the identification of medical bacteria. 3rd ed. Cambridge: Cambridge University Press; 1993.
- Chun J, Lee J-H, Jung Y, et al. EzTaxon: a web-based tool for the identification of prokaryotes based on 16S ribosomal RNA gene sequences. Int J Syst Evol Microbiol. 2007;57:2259–2261.
- Zerbino DR, Velvet: BE. Algorithms for de novo short read assembly using de Bruijn graphs. Genome Res. 2008;18:821–829.
- Nishi T, Ikemura T, Kanaya S. GeneLook: a novel ab initio gene identification system suitable for automated annotation of prokaryotic sequences. Gene. 2005;346:115–125.
- Laemmli UK. Cleavage of structural proteins during the assembly of the head of bacteriophage T4. Nature. 1970;227:680–685.
- Mitchell A, Chang HY, Daugherty L, et al. The Interpro protein families database: the classification resource after 15 years. Nucleic Acids Res. 2015;43:D213–D221.
- Yin Y, Mao X, Yang J, et al. A web resource for automated carbohydrate-active enzyme annotation. Nucleic Acids Res. 2012;40:445–451.
- Edgar RC. MUSCLE: multiple sequence alignment with high accuracy and high throughput. Nucleic Acids Res. 2004;32:1792–1797.
- Whelan S, Goldman N. A general empirical model of protein evolution derived from multiple protein families using a maximum-likelihood approach. Mol Biol Evol. 2001;18:691–699.
- Kumar S, Stecher G, Tamura K. MEGA7: molecular evolutionary genetics analysis version 7.0 for bigger datasets. Mol Biol Evol. 2016;33:1870–1874.
- Paice MG, Bourbonnais R, Desrochers M, et al. A xylanase gene from Bacillus subtilis: nucleotide sequence and comparison with B. gene. Arch Microbiol. 1986;144:201–206.
- Correia MAS, Mazumder K, JLA B, et al. Structure and function of an arabinoxylan-specific xylanase. J Biol Chem. 2011;286:22510–22520.
- Lowry OH, Rosebrough NJ, Farr AL, et al. Protein measurement with the Folin phenol reagent. J Biol Chem. 1951;193:265–275.
- Miller GL. Use of dinitrosalicylic acid reagent for determination of reducing sugar. Anal Chem. 1959;31:426–428.
- Blumenkrantz N, Asboe-Hansen G. New method for quantitative determination of uronic acids. Anal Biochem. 1973;54:484–489.
- Huq MA, Kim YJ, Hoang VA, et al. Paenibacillus ginsengiterrae sp. nov., a ginsenoside-hydrolyzing bacteria isolated from soil of ginseng field. Arch Microbiol. 2015;197:389–396.
- Rivas R, Garciá-Fraile P, Mateos PF, et al. Paenibacillus cellulosilyticus sp. nov., a cellulolytic and xylanolytic bacterium isolated from the bract phyllosphere of Phoenix dactylifera. Int J Syst Evol Microbiol. 2006;56:2777–2781.
- Shida O, Takagi H, Kadowaki K, et al. Transfer of Bacillus alginolyticus, Bacillus chondroitinus, Bacillus curdlanolyticus, Bacillus glucanolyticus, Bacillus kobensis, and Bacillus thiaminolyticus to the genus Paenibacillus and emended description of the genus Paenibacillus. Int J Syst Bacteriol. 1997;47:289–298.
- Aspeborg H, Coutinho PM, Wang Y, et al. Evolution, substrate specificity and subfamily classification of glycoside hydrolase family 5 (GH5). BMC Evol Biol. 2012;12:186.
- Boraston AB, Tomme P, Amandoron EA, et al. A novel mechanism of xylan binding by a lectin-like module from Streptomyces lividans xylanase 10A. Biochem J. 2000;350:933–941.
- Santos CR, Hoffmam ZB, de Matos Martins VP, et al. Molecular mechanisms associated with xylan degradation by Xanthomonas plant pathogens. J Biol Chem. 2014;289:32186–32200.
- Ma Y, Xue Y, Dou Y, et al. Characterization and gene cloning of a novel β-mannanase from alkaliphilic Bacillus sp. Extremophiles. 2004;8:447–454.
- Takeda N, Hirasawa K, Uchimura K, et al. Alkaline Mannanase from a Novel Species of Alkaliphilic Bacillus. J Appl Glycosci. 2004;51:229–236.
- Li R, Kibblewhite R, Orts WJ, et al. Molecular cloning and characterization of multidomain xylanase from manure library. World J Microbiol Biotechnol. 2009;25:2071–2078.
- Valenzuela SV, Diaz P, Javier Pastor FI. Modular glucuronoxylan-specific xylanase with a family CBM35 carbohydrate-binding module. Appl Environ Microbiol. 2012;78:3923–3931.
- Sainz-Polo MA, Valenzuela SV, González B, et al. Structural analysis of glucuronoxylan-specific Xyn30D and its attached CBM35 domain gives insights into the role of modularity in specificity. J Biol Chem. 2014;289:31088–31101.
- Vandermarliere E, Bourgois TM, Rombouts S, et al. Crystallographic analysis shows substrate binding at the −3 to +1 active-site subsites and at the surface of glycoside hydrolase family 11 endo-1,4-β-xylanases. Biochem J. 2008;410:71–79.
- Dodd D, Moon YH, Swaminathan K, et al. Transcriptomic analyses of xylan degradation by Prevotella bryantii and insights into energy acquisition by xylanolytic bacteroidetes. J Biol Chem. 2010;285:30261–30273.
- Ä U, Vršanská M, KBR MK, et al. Structural basis for substrate recognition by Erwinia chrysanthemi GH30 glucuronoxylanase. Febs J. 2011;278:2105–2116.
- Pollet A, Delcour JA, Courtin CM. Structural determinants of the substrate specificities of xylanases from different glycoside hydrolase families. Crit Rev Biotechnol. 2010;30:176–191.
- Khadem A, Lourenço M, Delezie E, et al. Does release of encapsulated nutrients have an important role in the efficacy of xylanase in broilers? Poult. Sci. 2016;95:1066–1076.