ABSTRACT
Inulin-type fructans are known to exert different effects on the fermentation profile depending on the average and range of the degree of polymerization (DP). Here, swine fecal cultures were used to investigate the prebiotic properties of native chicory inulin (NIN), extracted from the chicory root, and synthetic inulin (SIN), which has a narrower DP distribution than NIN. Both NIN and SIN showed prebiotic effects, but NIN exhibited a significant decrease in pH and increase in the production of propionate and butyrate compared to SIN. There were also differences in the production of succinate and lactate, the precursors of propionate and butyrate, and the relative abundance of associated genes. Furthermore, NIN induced the growth of certain species of Bifidobacterium and Lactobacillus more strongly than SIN. These results suggest that NIN and SIN exhibit different prebiotic properties due to differences in DP, and that NIN might be more beneficial to host health.
GRAPHICAL ABSTRACT
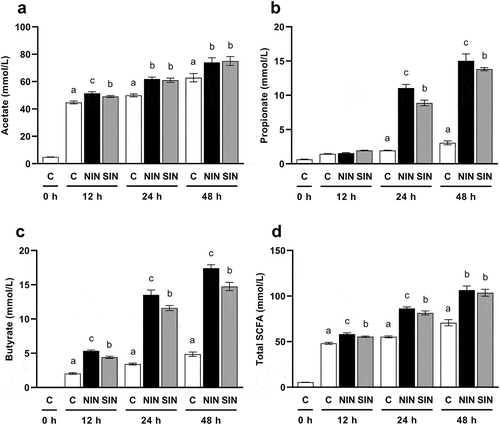
The superior prebiotic properties of native chicory inulin (NIN) compared to those of synthetic inulin (SIN), as demonstrated by propionate and butyrate production.
The colon is colonized by a complex microbial community, referred to as the gut microbiota, which is responsible for promoting normal intestinal function and protecting the host from infection [Citation1]. Disturbances of the gut microbiota, known as dysbiosis, are associated with many diseases, such as obesity, diabetes, and gastrointestinal disorders [Citation2]. Along with alterations in the composition of the microbiota, changes in the metabolic activity have also been observed. Many studies have suggested that the metabolic activity of the gut microbiota is as or more important than the composition [Citation3]. For instance, short-chain fatty acids (SCFA) such as acetate, propionate, and butyrate produced from the fermentation of carbohydrates are considered beneficial, as they are a source of energy for the colon epithelium and inhibit the growth of potential pathogens by lowering the luminal pH. There are growing interests in the involvement of propionate and butyrate in host health [Citation4,Citation5]. Propionate exerts a hypolipidemic effect, improves insulin sensitivity, relieves high blood pressure, and promotes satiety [Citation6,Citation7]. Butyrate improves intestinal barrier function and has anti-inflammatory, anti-cancer, and anti-oxidant activities [Citation6,Citation7]. Metabolites such as ammonia, indole, and branched-chain fatty acids (BCFA), derived from protein fermentation, are also produced by the gut microbiota. Unlike SCFA, ammonia and indole have been suggested to have an undesirable impact on host health [Citation8].
Prebiotics, which are food ingredients, shape the microbial composition and alter the production of SCFA in the gut, resulting in improved host health [Citation9,Citation10]. Prebiotics, including some dietary fibers, are resistant to gastrointestinal digestion and absorption, making them available in the colon for fermentation by specific microorganisms relevant to host health. This effect is generally recognized as an increase in the populations and/or activity of Bifidobacteria and Lactobacilli. A number of prebiotics have been isolated from various plant sources and have been manufactured industrially, each known to have different fermentability.
The best studied prebiotics are inulin-type fructans (ITF), including inulin, oligofructose (OF), and fructooligosaccharides (FOS) [Citation11]. ITF are linear polymers found in various plants, including chicory roots, and are composed of D-fructose with β-(2-1) fructosyl-fructose linkage and terminal α-glucose [Citation12,Citation13]. Food industries have commercialized the use of ITF for food application, as they improve the taste and texture of foods in addition to their function as a prebiotic. It is known that the degree of polymerization (DP) of fructans affects both prebiotic and physicochemical properties, including solubility and viscosity [Citation12,Citation14]. Native inulin (NIN) extracted from the chicory root has a DP of 3 to 60 units, while OF, its partial enzymatic hydrolysis product, and FOS generated by enzymatic transfer of fructosyl groups from sucrose, have a DP of 2–10 [Citation11]. Short-chain carbohydrates such as OF and FOS are fermented faster than inulin [Citation14]. Recently, synthetic inulin (SIN), a linear fructose polymer like NIN, has been industrially produced from sucrose by an enzyme from Bacillus sp. 217 C-1. Wada et al. [Citation15] reported that the average DP of SIN was 16–18, and the range of DP was less than 30 and narrower than that of NIN. SIN stimulates the growth of several species of Bifidobacterium and Lactobacillus, suggesting that SIN can behave as a prebiotic. Although NIN and SIN are both used as functional food ingredients and dietary supplements, it is not clear whether NIN and SIN have similar fermentation profiles and modulating effects on gut bacterial communities.
This study aimed to compare fermentation profiles of NIN and SIN by the swine gut microbiota in vitro. We also investigated the potential of NIN and SIN to influence the growth of specific bacterial groups.
Materials and methods
Materials
NIN Frutafit® IQ (range of DP 2 to 60, average DP 8 to 13) extracted from chicory root was provided by Sensus (Roosendaal, The Netherlands). Commercially available SIN (range of DP 5 to 30, average DP ~16) was purchased from Fuji Nihon Seito Corporation (Shizuoka, Japan). The DP of each inulin was determined based on manufacturer’s product information or published peer-reviewed papers [Citation15–Citation17].
Determination of molecular weight of NIN and SIN by gel permeation chromatography (GPC)
Aqueous solution (1.0%) of NIN or SIN was passed through a filter with a pore size of 0.45 μm (Millex-LH; Merck Millipore, Cork, Ireland). The molecular weight of NIN and SIN was determined using a Prominence GPC system (Shimadzu, Kyoto, Japan) equipped with a refractive index detector (RID-10A; Shimadzu) and TSKgel G2500PWXL (300 mmL. × 7.8 mm I.D., Tosoh, Tokyo, Japan). The mobile phase was water at a flow rate 0.5 mL/min, with a column temperature of 80°C. The detector temperature was maintained at 40°C. The molecular weight was calibrated with reference to pullulans standards (STANDARD P-82; Showa Denko, Tokyo, Japan).
In vitro fecal fermentation
Feces were collected from three swine fed an antibiotic-free diet and mixed in equal amounts. The fecal sample was homogenized with three volumes of 0.9% saline solution and filtered to remove large particles in an anaerobic chamber (BACTRON300; Sheldon Manufacturing, OR, USA) containing 90% N2, 5% H2, and 5% CO2, resulting in a fecal slurry. Fermentation was performed using 1.7% (w/v) fecal slurry in fermentation media based on the study conducted by Yu et al. [Citation18], with some minor modifications. NIN and SIN were added at a final concentration of 1.25% (w/v), while blank cultures (no substrate) were used as controls. The initial pH was adjusted to 6.9, followed by incubation in the anaerobic chamber at 37°C. Culture samples collected at 0, 12, 24, and 48 h were centrifuged at 10,000 rpm for 5 min. The pH value of each supernatant was measured with a LAQUAtwin pH meter (Horiba, Kyoto, Japan), and the supernatant and pellet were frozen separately at −80°C.
Analysis of organic acids by high performance liquid chromatography (HPLC)
A mixture of 0.45 mL of the culture supernatant and 1.0 mL of 0.5 N perchloric acid was passed through a filter with a pore size of 0.45 μm (Millex-LH; Merck Millipore). The concentration of SCFA (acetate, propionate, and butyrate), lactate, succinate, and BCFA (iso-butyrate and iso-valerate) in the samples was determined using a Prominence HPLC system (Shimadzu) using a post-column reaction with a conductivity detector (CDD-10A VP; Shimadzu). The separation of organic acids was achieved using a Shim-pack SCR-102H HPLC column (300 mmL. × 8 mm I.D., Shimadzu), and 5 mM p-toluene sulfonic acid as the mobile phase. A mixture solution of 5 mM p-toluene sulfonic acid, 20 mM Bis-Tris, and 0.1 mM EDTA was used as the reaction solution. The flow rate was 0.8 mL/min, and the oven temperature was 40°C. The detector cell temperature was maintained at 43°C.
Determination of ammonia and indole
Ammonia concentration was determined using an Ammonia-Test Wako kit (Fujifilm Wako Pure Chemical Corporation, Osaka, Japan) according to the manufacturer’s instructions. The indole concentration was determined using p-dimethyl aminobenzaldehyde reagent as described previously [Citation19].
DNA extraction and quantitative real-time polymerase chain reaction (PCR) analysis
Bacterial genomic DNA was extracted from 48 h samples using a NucleoSpin DNA Stool Kit (Macherey-Nagel, Düren, Germany) according to the manufacturer’s instructions. The concentration of extracted DNA was measured using a Tecan Spark (Tecan, Zurich, Switzerland) and stored at −80°C until use. Amplification and detection of purified bacterial DNA were carried out using a CFX384 Touch Real-Time PCR Detection System (Bio-Rad, Hercules, CA, USA) with KOD SYBR qPCR Mix (Toyobo, Tokyo, Japan) or Fast SYBR Green Master Mix (Thermo Fisher Scientific, Waltham, MA, USA). shows the primer sequences used for all PCR analyses. Propionate and butyrate are produced by pathways mainly via methylmalonyl-CoA decarboxylase and butyryl-CoA:acetate-CoA transferase (BCoAT), respectively [Citation4,Citation20,Citation21]. The primers for mmdA, encoding methylmalonyl-CoA decarboxylase, and BCoAT were used to quantify propionate and butyrate producers. As Bacteroides and Prevotella, the major producers of propionate, are known to have different mmdA sequences, we used specific mmdA primers for these two genera [Citation22]. Amplification using the KOD SYBR qPCR Mix was performed at 98°C for 2 min, followed by 40 cycles at 98°C for 10 s, the optimum annealing temperature () for 30 s and 68°C for 60 s. Amplification using the Fast SYBR Green Master Mix was performed at 95°C for 10 min, followed by 39 cycles at 95°C for 15 s, and 60°C for 60 s. The cycle threshold (Ct) of amplified products was detected using the manufacturer’s software. Relative abundances of targeted bacteria and bacterial genes were determined by the Ct method using the Ct values of primers to total bacteria as the reference. The reference sample (control) has the 2 – (Ct target – Ct total bacteria) value of 1. A melt curve analysis was performed to ensure primer specificity.
Table 1. Primers used for quantitative real-time PCR analysis.
Statistics
All data are expressed as mean ± standard deviation (SD). To investigate the effects of NIN and SIN on pH and the concentrations of organic acids, ammonia, and indole, these data were analyzed using two-way analysis of variance (ANOVA) for repeated measures with the factors, treatment and time. When significant interactions (treatment × time) were found (p < 0.05), one-way ANOVA, followed by Tukey’s post hoc test, was used to analyze statistical significance for each time point. Relative abundances of bacteria and bacterial genes were analyzed by one-way ANOVA with Tukey’s post hoc test. The analyses were performed using GraphPad Prism version 8.3.0 (GraphPad Software, San Diego, CA, USA). p < 0.05 was considered statistically significant.
Results
DP distribution of NIN and SIN
GPC was carried out to confirm the DP distribution of NIN and SIN. NIN had a peak DP of 25.1, weight average DP (DPw) of 21.7, and number average DP (DPn) of 14.5, while SIN demonstrated a peak DP of 22.3, DPw of 20.5, and DPn of 18.5 (). The width of the distribution (polydispersity) of both inulins was measured as the ratio of DPw to DPn (DPw/DPn). The DPw/DPn of NIN was 1.49 and that of SIN was 1.11, indicating that the range of the DP of NIN was wider than that of SIN because a larger DPw/DPn represents a wider distribution of molecular weight.
Table 2. Peak degree of polymerization (DP), weight average DP (DPw), number average DP (DPn), and polydispersity (DPw/DPn) of native chicory inulin (NIN) and synthetic inulin (SIN).
Changes in pH
Changes in pH in each sample were monitored for 48 h to investigate the progress of fermentation in fecal cultures (). The pH values of the control cultures increased gradually after a slight decrease in pH was observed at 12 h. The two-way ANOVA for repeated measures indicated a significant effect of treatment (F(2,12) = 809.6, p < 0.001) and a significant treatment × time interaction (F(4,24) = 131.7, p < 0.001). The one-way ANOVA, followed by Tukey’s post hoc test, showed that NIN and SIN had significantly lower pH values than the control at every time point. At 12 h, NIN exhibited a significantly lower pH than SIN. The pH pattern was maintained throughout the fermentation period. The lowest pH was achieved by NIN, with a value of 6.10 at 48 h of fermentation.
SCFA concentration
The cumulative concentrations of SCFA during in vitro fecal fermentation were measured. Acetate was the most abundant SCFA in all groups, exceeding 60 mM at 48 h ()). The two-way repeated measures ANOVA revealed a significant effect of treatment (F(2,12) = 51.85, p < 0.001) and a significant treatment × time interaction (F(4,24) = 11.90, p < 0.001). There were significant increases in acetate production with NIN and SIN compared to those in the control at every time point as determined from the one-way ANOVA analysis, followed by Tukey’s post hoc test. A significant difference in the concentration of acetate between NIN and SIN was observed at 12 h, but not after 24 h. The two-way repeated measures ANOVA revealed significant effects of treatment and significant treatment × time interactions for concentrations of propionate (treatment: F(2,12) = 715.5, p < 0.001, treatment × time: F(4,24) = 460.9, p < 0.001), butyrate (treatment: F(2,12) = 1309, p < 0.001, treatment × time: F(4,24) = 275.3, p < 0.001), and total SCFA (treatment: F(2,12) = 224.7, p < 0.001, treatment × time: F(4,24) = 89.56, p < 0.001). The fermentation of NIN and SIN resulted in a significant increase in propionate after 24 h, compared to that in the control ()). The concentration of propionate for NIN was significantly higher than that for SIN at 24 and 48 h. Quantitative measurement confirmed a time-dependent increase in butyrate production in NIN and SIN groups ()). Interestingly, NIN showed significantly higher butyrate production than SIN at every time point. The final butyrate concentrations in NIN and SIN were 3.6 and 3.0 times higher than those in the control, respectively. Total SCFA production tended to follow the same trend as that of acetate ()). In other words, the total SCFA for both inulin groups was significantly higher than that of the control at every time point, with the levels in SIN being similar to those in NIN at 48 h.
Lactate and succinate concentration
In the gut, lactate and succinate are precursors of propionate production, and some butyrate producers can produce butyrate from lactate [Citation4,Citation21]. To elucidate the reasons for the differences in the production of propionate and butyrate between the NIN and SIN groups, we measured the concentrations of lactate and succinate during in vitro fecal fermentation (). The two-way repeated measures ANOVA revealed significant effects of treatment and significant treatment × time interactions for concentrations of lactate (treatment: F(2,12) = 489.5, p < 0.001, treatment × time: F(4,24) = 82.96, p < 0.001) and succinate (treatment: F(2,12) = 1897, p < 0.001, treatment × time: F(4,24) = 382.8, p < 0.001). Lactate and succinate production for NIN and SIN was significantly increased at every time point, except for lactate in the SIN group at 12 h, compared to that in the control. The concentrations of both organic acids in the NIN group were significantly higher than those in the SIN group throughout the fermentation.
BCFA, ammonia, and indole concentration
Gut bacteria can utilize proteins as an energy source if carbohydrates are not sufficiently available, increasing the concentrations of potentially toxic end-products such as BCFA, ammonia, and indole [Citation8]. The two-way repeated measures ANOVA revealed significant effects of treatment and significant treatment × time interactions for concentrations of iso-butyrate (treatment: F(2,12) = 43.79, p < 0.001, treatment × time: F(4,24) = 26.20, p < 0.001), ammonia (treatment: F(2,12) = 45.43, p < 0.001, treatment × time: F(4,24) = 2.779, p < 0.05), and indole (treatment: F(2,12) = 220.3, p < 0.001, treatment × time: F(4,24) = 43.27, p < 0.001). The iso-butyrate for NIN and SIN was significantly reduced compared to that for the control at 24 and 48 h ()); iso-valerate was not detected in any samples. The concentrations of ammonia and indole were increased in the control cultures after 12 h (). The levels were significantly lower in the NIN and SIN groups than in the control group at 24 and 48 h. There was no difference in the inhibitory effects on the formation of iso-butyrate, ammonia, and indole between the inulin-types.
Quantitative real-time PCR analysis of Bifidobacterium and Lactobacillus species
It has been shown that inulin stimulates the growth of Bifidobacteria and Lactobacilli in the colon [Citation23]. We investigated the effects of NIN and SIN on the relative abundance of several species of Bifidobacterium and Lactobacillus, which are known to be present in the gut of humans and swine, in fecal fermentation samples after 48 h of incubation. Increased abundances of the Bifidobacterium catenulatum group (comprising B. catenulatum and Bifidobacterium pseudocatenulatum) and Bifidobacterium dentium were observed the NIN and SIN groups compared to those in the control (). Interestingly, the effects of NIN on the growth of the B. catenulatum group and B. dentium were significantly stronger than those of SIN. The growth of Bifidobacterium adolescentis was not promoted by NIN and SIN ()). As shown in , both inulins induced the growth of the Lactobacillus reuteri subgroup and Lactobacillus fermentum but had no effect on the relative abundance of Lactobacillus salivarius. Of note, NIN increased the relative abundance of the L. reuteri subgroup over SIN, while NIN and SIN did not differ for the growth of L. fermentum.
Quantitative real-time PCR analysis of mmdA and BCoAT genes
The relative abundances of mmdA and BCoAT in the fermentation samples at 48 h were examined to confirm the difference in the effects of NIN and SIN on the production of propionate and butyrate (). A significant increase in the relative abundance of Bacteroides-associated mmdA was observed only with NIN. In contrast, the relative abundance of Prevotella-associated mmdA was reduced by the incubation of both inulins compared to that in the control. The addition of NIN and SIN to fecal cultures resulted in significant increases in BCoAT after 48 h compared to that in the control. The abundance of BCoAT for NIN was significantly higher than that for SIN.
Discussion
ITF are the most studied prebiotics and are widely used in commercial applications. ITF have been reported to show beneficial effects on host health, such as promotion of growth of Bifidobacteria and Lactobacilli, increased SCFA, and reduced protein fermentation [Citation23–Citation25]. There are many studies on the prebiotic properties of NIN, OF, and FOS, but only a limited number regarding SIN. The present study investigated the prebiotic properties of NIN and SIN using in vitro swine fecal cultures. We found that fermentations of both inulins increased SCFA production and reduced the concentration of protein fermentation products. In addition, both inulins enhanced the growth of several species of Bifidobacterium and Lactobacillus. These results are in line with previous studies that examined the prebiotic properties of NIN, OF, and FOS in human and swine feces [Citation24,Citation26,Citation27].
There were some notable differences in the fermentation profiles of NIN and SIN. ITF with various averages and ranges of DP have been shown to exert different influences on the fermentation capacity of gut bacteria [Citation28]. Stewart et al. [Citation27] reported that FOS (DP < 10) was fermented rapidly and that inulin (DP > 10) was fermented more slowly by the human gut microbiota in vitro. In particular, the differences in chain length were clearly seen in the production of acetate and total SCFA [Citation27]. The chain length of inulin also seems to affect its fermentability by swine gut microbiota, as inulin with a lower DP leads to a more rapid rate of SCFA production than that with higher DP [Citation24]. In this study, fermentation of NIN resulted in a higher production of acetate and total SCFA than that of SIN at 12 h. However, the concentrations of acetate and total SCFA for both inulins reached similar levels at 48 h. NIN contains higher levels of OF (DP < 10) than SIN because NIN is an unfractionated, spray-dried inulin isolated from chicory roots, although they both contain ITF of DP > 10 [Citation15]. The OF present in NIN might explain why NIN exhibits a more rapid increase in acetate and total SCFA than SIN.
The dependence of shorter chain molecules of ITF on propionate production is not as great as that of acetate and total SCFA. For instance, Kaur et al. [Citation29] reported that the fermentation of long-chain inulin (range of DP 10 to 60, average DP 25 [Citation14,Citation30]) resulted in the production of propionate to the same extent as that of FOS. Interestingly, it has been shown that NIN and long-chain inulin fermentations produce more propionate in vitro than those of OF and FOS [Citation25,Citation31]. We found a higher concentration of propionate in the NIN group than in the SIN group at 24 and 48 h. The DP range of NIN is from 3 to 60, while the maximum DP of SIN is less than 30. Longer chain molecules containing NIN might be more effective as fermentative substrates for propionate production.
Propionate is synthesized from succinate via the succinate pathway or from lactate via the acrylate pathway [Citation21,Citation32,Citation33]. The notable increased production of succinate and lactate by fermentation of NIN might explain why the concentration of propionate was higher than that of SIN. Succinate is mainly produced in the gut by bacteria belonging to the phylum Bacteroidetes, which are abundant in the gut microbiota [Citation20]; Bacteroides thetaiotaomicron and Bacteroides fragilis can ferment inulin to produce succinate [Citation34]. Furthermore, succinate levels and the relative abundance of Bacteroides are increased in the cecum of mice fed FOS [Citation35]. Therefore, it is suspected that the significant amount of propionate following the fermentation of NIN was due to the production of succinate by Bacteroides.
While there is consensus that ITF with a lower DP, such as FOS, leads to a more rapid rate of butyrate production [Citation24,Citation27,Citation29], the effect of chain length of ITF on the amount of butyrate is controversial. For instance, Kaur et al. [Citation29] showed that fermentation of FOS resulted in a higher production of butyrate than did that of long-chain inulin, while there are some reports that the chain length of ITF does not affect the final amount of butyrate produced [Citation24,Citation26]. It has been reported that ITF with a wider range of DP, such as NIN and oligofructose-enriched inulin (mixture of OF and long-chain inulin), have a higher butyrate production than OF, FOS, and long-chain inulin alone. Contrastingly, butyrate production by OF and FOS is equivalent to that by long-chain inulin at the end of fermentation [Citation26]. Therefore, it is suspected that both the shorter and longer chain molecules of ITF are strongly involved in butyrate production. This is in line with our finding that NIN with a wider range of DP showed significantly higher butyrate production than SIN at every time point.
Acetate and lactate generated by Bifidobacteria and Lactobacilli degrading ITF are important to produce butyrate in the gut [Citation36,Citation37]. These metabolites are used as substrates for butyrate-producing bacteria and induce the production of butyrate by them. Bifidobacteria prefer short-chain ITF for the production of acetate and lactate, while some Lactobacillus strains completely degrade long-chain inulin and produce lactate [Citation36,Citation37]. This means that ITF with a wider range of DP can effectively induce production of lactate for use as a substrate for butyrate-producing bacteria. In fact, the present study showed that NIN fermentation produced more butyrate than SIN with a narrow range of DP.
The succinate pathway is known as the main route for propionate formation and is mainly found in the genera Bacteroides and Prevotella in the gut [Citation20]. In these bacteria, the succinate pathway is mediated by methylmalonyl-CoA decarboxylase, which is encoded by mmdA [Citation21,Citation22]. The final step of butyrate formation is catalyzed by BCoAT in butyrate-producing bacteria [Citation22,Citation38]. The NIN group had significantly higher relative amounts of Bacteroides-associated mmdA and BCoAT than the control and SIN groups. These results support the higher production of propionate and butyrate by NIN fermentation. Unexpectedly, both inulin fermentations suppressed the relative abundances of Prevotella-associated mmdA compared to that of the control. Chen et al. [Citation39] reported that fermentation of FOS by a Prevotella-dominated microbiota increased propionate production compared to that by a Bacteroides-dominated microbiota, in an in vitro fecal culture study. On the contrary, propionate production is increased in the cecum of mice colonized with B. thetaiotaomicron, while propionate is not detected in colonization of Prevotella copri [Citation40]. Further research is needed to investigate whether ITF induce the production of propionate in the genus Prevotella.
The pH of the gut lumen is an indicator of fermentation and plays a role in regulating the gut ecosystem. In this study, both inulins caused a decrease in pH, but the pH drop due to NIN fermentation was significantly stronger than that due to SIN, indicating that NIN was fermented to a greater degree by fecal bacteria. Lowering the pH has potential positive effects on host gut health, as it has been shown to inhibit the growth of some harmful bacteria. Furthermore, acidic pH can reduce protein fermentation products such as BCFA, ammonia, and indole by inhibiting proteolytic fermentation [Citation8]. However, NIN and SIN equally reduced products of protein fermentation in this study. It has been shown that the protein fermentation products were suppressed to the same extent by the addition of ITF with various DPs [Citation41], indicating that the average and range of DP of ITF do not affect protein fermentation in the gut. The inhibitory effects of ammonia and indole, considered potentially harmful to host health, might not differ between NIN and SIN.
Prebiotics including ITF modulate the composition of gut microbiota at the species level [Citation42]. The quantitative real-time PCR results showed that NIN and SIN have different prebiotic properties for several Bifidobacterium and Lactobacillus species commonly present in the gut of humans and swine. Compared to SIN, NIN significantly induced the growth of the B. catenulatum group and B. dentium among the three species of Bifidobacterium and the L. reuteri subgroup among the three species of Lactobacillus, indicating that NIN and SIN are different not only in the metabolic activity but also in changing the composition of gut microbiota. The increases of the B. catenulatum group, B. dentium, and the L. reuteri subgroup in the gut are considered beneficial for host health [Citation43–Citation45].
We acknowledge a few limitations in this study. First, the in vitro model used might not reflect the actual situation in vivo. For instance, our model does not absorb metabolites such as SCFA produced as a result of fermentation. Therefore, unlike an in vivo model, a reduction in pH occurs during the fermentation of inulin. On the other hand, the notable results of this study were that NIN showed significantly higher propionate and butyrate production than SIN at every time point, while acetate production by SIN was similar to that by NIN at 48 h. It is difficult to confirm such changes in fermentation metabolites over time in an in vivo study because the majority of SCFA are absorbed by colonic epithelial cells [Citation46]. We therefore selected this in vitro model to examine the time-dependent differences in the fermentation properties of NIN and SIN. Second, swine feces were used as inocula for this in vitro fermentation study. It might be difficult to predict the effects of NIN and SIN on human health from our results. It is known that there are large inter-differences in gut microbiota composition in humans, and the composition and activity of gut microbiota are influenced by diet [Citation1]. Swine, unlike humans, always consume the same diet, which suggests that the inter-individual differences in the gut microbiota composition in swine are smaller than those in humans. Therefore, we used swine feces instead of human feces in this study for more stable results. Swine have been used to assess the gut microbiota of humans because of their similarities, including gastrointestinal function and nutritional requirements [Citation47]. In addition, the gut microbiota of swine has been reported to be similar to that of humans, for example, in terms of diversity patterns and dominant phyla [Citation48]. Nevertheless, a fermentation assay using human feces might be required to determine the effects of NIN and SIN on human gut microbiota.
In conclusion, the present study showed that NIN and SIN, including ITF with DP > 10, exerted beneficial effects as prebiotics in vitro in swine fecal cultures. However, NIN more strongly induced the production of propionate and butyrate and the growth of certain species of Bifidobacterium and Lactobacillus than did SIN. These differences in prebiotic properties between the two inulins might be related to the fact that NIN contains ITF with a wider range of DP than SIN. Taken together, administration of NIN, rather than SIN, may elicit a better host response due to its overall superior prebiotic properties; further investigation into the properties of NIN will reveal its full potential.
Author contribution
All members designed the experiments and discussed the results for the completion of the manuscript. YN, NK, TT, SA, and KT performed the experiments and analyzed data. YN wrote the draft of manuscript. TT, AN, and EK edited the manuscript. All authors reviewed and approved the manuscript.
Acknowledgments
We would like to thank Mayumi Shoji for her secretarial work. The authors would like to thank Editage (www.editage.com) for English language editing.
Disclosure statement
YN, NK, TT, SA, KT, and EK are employees of Teijin Limited, the sales company of Frutafit® IQ (chicory root-derived native inulin) in Japan. AK has no conflict of interest to disclose.
References
- Mills M, Stanton S, Lane L, et al. Precision nutrition and the microbiome, part I: current state of the science. Nutrients. 2019;11(4):923.
- Das B, Nair GB. Homeostasis and dysbiosis of the gut microbiome in health and disease. J Biosci. 2019;44(5):117.
- Cani PD, Everard A, Duparc T. Gut microbiota, enteroendocrine functions and metabolism. Curr Opin Pharmacol. 2013;13(6):935–940.
- Louis P, Flint HJ. Formation of propionate and butyrate by the human colonic microbiota. Environ Microbiol. 2017;19(1):29–41.
- McNabney SM, Henagan TM. Short chain fatty acids in the colon and peripheral tissues: A focus on butyrate, colon cancer, obesity and insulin resistance. Nutrients. 2017;9(12):1348.
- Cui J, Lian Y, Zhao C, et al. Dietary fibers from fruits and vegetables and their health benefits via modulation of gut microbiota. Compr Rev Food Sci Food Saf. 2019;18(5):1514–1532.
- Riviere A, Selak M, Lantin D, et al. Bifidobacteria and butyrate-producing colon bacteria: importance and strategies for their stimulation in the human gut. Front Microbiol. 2016;7:979.
- Diether NE, Willing BP. Microbial fermentation of dietary protein: an important factor in diet–microbe–host interaction. Microorganisms. 2019;7(1):19.
- Delcour JA, Aman P, Courtin CM, et al. Prebiotics, fermentable dietary fiber, and health claims. Adv Nutr. 2016;7(1):1–4.
- Slavin J. Fiber and prebiotics: mechanisms and health benefits. Nutrients. 2013;5(4):1417–1435.
- Meyer D, Stasse-Wolthuis M. The bifidogenic effect of inulin and oligofructose and its consequences for gut health. Eur J Clin Nutr. 2009;63(11):1277–1289.
- Mensink MA, Frijlink HW, van der Voort Maarschalk K, et al. Inulin, a flexible oligosaccharide I: review of its physicochemical characteristics. Carbohydr Polym. 2015;130:405–419.
- Shoaib M, Shehzad A, Omar M, et al. Inulin: properties, health benefits and food applications. Carbohydr Polym. 2016;147:444–454.
- Van Loo J. The specificity of the interaction with intestinal bacterial fermentation by prebiotics determines their physiological efficacy. Nutr Res Rev. 2004;17(1):89–98.
- Wada T, Sugatani J, Terada E, et al. Physicochemical characterization and biological effects of inulin enzymatically synthesized from sucrose. J Agric Food Chem. 2005;53(4):1246–1253.
- Sugatani J, Osabe M, Wada T, et al. Comparison of enzymatically synthesized inulin, resistant maltodextrin and clofibrate effects on biomarkers of metabolic disease in rats fed a high-fat and high-sucrose (cafeteria) diet. Eur J Nutr. 2008;47(4):192–200.
- Takemura N, Ozawa K, Kimura N, et al. Inulin-type fructans stimulated the growth of exogenously administered Lactobacillus plantarum no. 14 in the mouse gastrointestinal tract. Biosci Biotechnol Biochem. 2010;74(2):375–381.
- Yu Z-T, Liu B, Mukherjee P, et al. Trametes versicolor extract modifies human fecal microbiota composition in vitro. Plant Foods Hum Nutr. 2013;68(2):107–112.
- Aoki T, Yamaji I, Hisamoto T, et al. Irregular bowel movement in gastrectomized subjects: bowel habits, stool characteristics, fecal flora, and metabolites. Gastric Cancer. 2012;15(4):396–404.
- Connors J, Dawe N, Van Limbergen J. The role of succinate in the regulation of intestinal inflammation. Nutrients. 2019;11(1):25.
- Reichardt N, Duncan SH, Young P, et al. Phylogenetic distribution of three pathways for propionate production within the human gut microbiota. ISME J. 2014;8(6):1323–1335.
- Mu C, Yang Y, Luo Z, et al. Temporal microbiota changes of high-protein diet intake in a rat model. Anaerobe. 2017;47:218–225.
- Gupta N, Jangid AK, Pooja D, et al. Inulin: a novel and stretchy polysaccharide tool for biomedical and nutritional applications. Int J Biol Macromol. 2019;132:852–863.
- Han K-H, Kobayashi Y, Nakamura Y, et al. Comparison of the effects of longer chain inulins with different degrees of polymerization on colonic fermentation in a mixed culture of swine fecal bacteria. Nutr Sci Vitaminol. 2014;60(3):206–212.
- van de Wiele T, Boon N, Possemiers S, et al. Inulin-type fructans of longer degree of polymerization exert more pronounced in vitro prebiotic effects. J Appl Microbiol. 2007;102(2):452–460.
- Hernot DC, Boileau TW, Bauer LL, et al. In vitro fermentation profiles, gas production rates, and microbiota modulation as affected by certain fructans, galactooligosaccharides, and polydextrose. J Agric Food Chem. 2009;57(4):1354–1361.
- Stewart ML, Timm DA, Slavin JL. Fructooligosaccharides exhibit more rapid fermentation than long-chain inulin in an in vitro fermentation system. Nutr Res. 2008;28(5):329–334.
- Kelly G. Inulin-type prebiotics--a review: part 1. Altern Med Rev. 2008;13(4):315–329.
- Kaur A, Rose DJ, Rumpagaporn P, et al. In vitro batch fecal fermentation comparison of gas and short-chain fatty acid production using “slowly fermentable” dietary fibers. J Food Sci. 2011;76(5):H137–142.
- Roberfroid MB. Introducing inulin-type fructans. Br J Nutr. 2005;93(Suppl S1):S13–25.
- Gomez E, Tuohy KM, Gibson GR, et al. In vitro evaluation of the fermentation properties and potential prebiotic activity of agave fructans. J Appl Microbiol. 2010;108(6):2114–2121.
- Koh A, De Vadder F, Kovatcheva-Datchary P, et al. From dietary fiber to host physiology: short-chain fatty acids as key bacterial metabolites. Cell. 2016;165(6):1332–1345.
- Sun M, Wu W, Liu Z, et al. Microbiota metabolite short chain fatty acids, GPCR, and inflammatory bowel diseases. J Gastroenterol. 2017;52(1):1–8.
- Rios-Covian D, Arboleya S, Hernandez-Barranco AM, et al. Interactions between Bifidobacterium and Bacteroides species in cofermentations are affected by carbon sources, including exopolysaccharides produced by bifidobacteria. Appl Environ Microbiol. 2013;79(23):7518–7524.
- De Vadder F, Kovatcheva-Datchary P, Zitoun C, et al. Microbiota-produced succinate improves glucose homeostasis via intestinal gluconeogenesis. Cell Metab. 2016;24(1):151–157.
- De Vuyst L, Moens F, Selak M, et al. Summer meeting 2013: growth and physiology of bifidobacteria. J Appl Microbiol. 2014;116(3):477–491.
- Moens F, Verce M, De Vuyst L. Lactate- and acetate-based cross-feeding interactions between selected strains of lactobacilli, bifidobacteria and colon bacteria in the presence of inulin-type fructans. Int J Food Microbiol. 2017;241:225–236.
- Anand S, Kaur H, Mande SS. Comparative in silico analysis of butyrate production pathways in gut commensals and pathogens. Front Microbiol. 2016;7:1945.
- Chen T, Long W, Zhang C, et al. Fiber-utilizing capacity varies in Prevotella- versus Bacteroides-dominated gut microbiota. Sci Rep. 2017;7(1):2594.
- Kovatcheva-Datchary P, Nilsson A, Akrami R, et al. Dietary fiber-induced improvement in glucose metabolism is associated with increased abundance of Prevotella. Cell Metab. 2015;22(6):971–982.
- van Nuenen MHMC, Meyer PD, Venema K. The effect of various inulins and clostridium difficileon the metabolic activity of the human colonic microbiota in vitro. Microb Ecol Health Dis. 2003;15(2–3):137–144.
- Chung WSF, Walker AW, Louis P, et al. Modulation of the human gut microbiota by dietary fibres occurs at the species level. BMC Biol. 2016;14(1):3.
- Engevik MA, Luk B, Chang-Graham AL, et al. Bifidobacterium dentium fortifies the intestinal mucus layer via autophagy and calcium signaling pathways. MBio. 2019;10(3):e01087–19.
- Kerckhoffs AP, Samsom M, van der Rest MEVD, et al. Lower Bifidobacteria counts in both duodenal mucosa-associated and fecal microbiota in irritable bowel syndrome patients. World J Gastroenterol. 2009;15(23):2887–2892.
- Mu Q, Tavella VJ, Luo XM. Role of Lactobacillus reuteri in human health and diseases. Front Microbiol. 2018;9:757.
- Wang M, Wichienchot S, He X, et al. In vitro colonic fermentation of dietary fibers: fermentation rate, short-chain fatty acid production and changes in microbiota. Trends Food Sci Technol. 2019;88:1–9.
- Heinritz SN, Mosenthin R, Weiss E. Use of pigs as a potential model for research into dietary modulation of the human gut microbiota. Nutr Res Rev. 2013;26(2):191–209.
- Turner PV. The role of the gut microbiota on animal model reproducibility. Animal Model Exp Med. 2018;1(2):109–115.