Abstract
Objective
Granular cells (GCs) are involved in polycystic ovarian syndrome (PCOS) progression. MicroRNA (miR)-23a downregulation is linked to PCOS development. Therefore, this research explored the influences of miR-23a-3p on GC proliferation and apoptosis in PCOS.
Methods
Reverse transcription-quantitative polymerase chain reaction (RT-qPCR) and western blotting were conducted to examine miR-23a-3p and HMGA2 expression in GCs of patients with PCOS. Then, miR-23a-3p and/or HMGA2 expression was altered in GCs (KGN and SVOG), after which miR-23a-3p, HMGA2, Wnt2, and β-catenin expression, GC viability, and GC apoptosis were measured by RT-qPCR and western blotting, MTT assay, and flow cytometry, respectively. A dual-luciferase reporter gene assay was utilized to assess the targeting relationship between miR-23a-3p and HMGA2. Finally, GC viability and apoptosis were tested after the combined treatment of miR-23a-3p mimic and pcDNA3.1-HMGA2.
Results
miR-23a-3p was poorly expressed but HMGA2 was overexpressed in GCs of patients with PCOS. Mechanistically, HMGA2 was negatively targeted by miR-23a-3p in GCs. Furthermore, miR-23a-3p inhibition or HMGA2 upregulation elevated viability and reduced apoptosis of KGN and SVOG cells, along with increased Wnt2 and β-catenin expression. In KNG cells, HMGA2 overexpression abrogated the impacts of miR-23a-3p overexpression on GC viability and apoptosis.
Conclusions
Collectively, miR-23a-3p decreased HMGA2 expression to block the Wnt/β-catenin pathway, thereby depressing viability and facilitating apoptosis of GCs.
Introduction
As a common endocrine disorder disturbing 5%–20% of females of childbearing age worldwide, polycystic ovarian syndrome (PCOS) is featured by polycystic ovarian morphology, anovulation, hyperandrogenism, and other metabolic abnormalities including insulin resistance and compensatory hyperinsulinemia [Citation1]. However, the accurate diagnosis and effective therapies for PCOS is still lacking because its pathogenesis has not been fully elucidated [Citation2]. Granular cells (GCs), flat cells surrounding oocytes, proliferate and experience multiple biochemical processes in follicles of mammalian ovary [Citation3]. Moreover, GC apoptosis affects the follicular development and reproductive capability of mammals [Citation4]. Previous research elaborated the abnormal proliferation and apoptosis of GCs in patients with PCOS [Citation5], suggesting the correlation between GC deregulation and PCOS development. Therefore, it is of great necessity to explore the molecular mechanism underlying the function of GCs for PCOS therapies.
microRNAs (miRs) have been implicated in insulin resistance, hormone secretion, hyperandrogenism, chronic anovulation, and polycystic ovaries during PCOS [Citation6–8]. In addition, the aberrant expression of miRs is measured in the serum and GCs of patients with PCOS, which indicates that miRs may be associated with the occurrence and progression of PCOS [Citation9,Citation10]. For instance, miR-23a overexpression induces apoptosis in human GCs [Citation11]. Moreover, miR-23a-3p, located in the miR-23a∼27a∼24-2 cluster, has been confirmed to be downregulated during TNF-α-induced insulin resistance [Citation12]. These observations suggest that miR-23a-3p may be implicated in PCOS development. Of note, many genes have been observed as target genes of miR-23a in diseases [Citation13]. For example, high mobility group A2 (HMGA2), a non-histone chromosomal protein, is targeted by miR-23a in cell senescence [Citation14]. Moreover, the variant allele of HMGA2 affects the risk of PCOS [Citation15]. In addition, HMGA2 has been observed to facilitate GC proliferation in PCOS [Citation16] and influence PCOS development [Citation17]. More importantly, as a downstream pathway of HMGA2 in diseases, the Wnt/β-catenin pathway correlates to insulin resistance and estrogen deficiency in PCOS [Citation18–20].
In this context, we hypothesized that miR-23a-3p might orchestrate GCs in PCOS via HMGA2 and the Wnt/β-catenin pathway. Thus, this research probed whether miR-23a-3p targeted HMGA2 and mediated the Wnt/β-catenin pathway to control the proliferation and apoptosis of GCs in PCOS.
Materials and methods
Clinical samples
The clinical samples were harvested from 30 patients who were diagnosed with PCOS and 30 control patients in Urumqi Maternal and Child Health Hospital. All patients received in vitro fertilization-embryo transfer (IVF-ET) treatment. Patients who suffered from infertility due to PCOS were arranged in the experiment group, whereas individuals who suffered from infertility due to other factors were arranged in the control group. The clinical characteristic of the participants are listed in . Patients were diagnosed with PCOS by more than two physicians with the title of attending doctor or above according to Rotterdam criteria [Citation21]. In addition, patients met two out of the following three criteria at the same time: (1) oligo-ovulation or anovulation (the menstrual cycle is longer than 35 days or the times of menstruation are less than 8 times per year); (2) clinical or biochemical manifestation of hyperandrogenism (total circulating testosterone is over 45 ng/dL or the appearance of hirsutism); (3) polycystic ovaries (ultrasonic detection of over 12 ovarian follicles with a diameter smaller than 9 mm) with other etiological factors excluded. The inclusion criteria of the control group were as follows: normal ovarian function and morphology, with regular menstrual cycle (26–35 days), androgen levels < 45 ng/dL, 6–10 antral follicles in bilateral ovaries, and infertility attributed to male and/or fallopian tube factors. The exclusion criteria of patients were as follows: patients aged over 35 years, with a history of ovarian diseases, a history of ovarian surgery or chemoradiotherapy, excessive prolactin levels, endometriosis, chromosomal abnormality, or hyperthyroidism; patients who used medications that affected glucolipid metabolism and hormone levels (diabetes, hypertension, and chronic kidney disease) within six months prior to consultation. The inclusion and exclusion criteria referred to a previous reference [Citation13]. Before the study, all participants had known the objectives and requirements of this study and provided their written informed consent. The study project was approved by the Ethic Committee of Urumqi Maternal and Child Health Hospital and conformed to the Declaration of Helsinki.
Table 1. The basic clinical characteristics of participants.
Extraction of GCs from PCOS patients
GCs were extracted according to experimental methods in prior documents [Citation22,Citation23]. The follicular development of patients was observed by ultrasound every 1–3 days, and estradiol and progesterone levels in blood were tested. Human chorionic gonadotropin (HCG) was injected when two or more follicles with the diameter of >18 mm were observed. After HCG injection for 36 h, ovum (the diameter > 15 mm) was extracted under the guidance of transvaginal ultrasound. About 3 days later, healthy embryos (less than 3) were implanted in the uterus. Fourteen days after embryo implantation, HCG in the serum and urine were detected. If the HCG result was positive, pregnancy was verified by ultrasound 2 weeks later. On the day of ovum extraction, once the oocyte-corona-cumulus complex was extracted, the remaining mixture was collected for centrifugation at 2000 rpm for 10 min at room temperature. The supernatant was follicular fluid and the precipitate was GCs. The precipitate was then digested with certain amount of hyaluronidase for a water bath at 37 °C for 30 min, during which the precipitate was shaken repeatedly, each for 30 s. Then, the cell suspension was transferred into a lymphocyte separation medium (5 mL) pre-warmed at room temperature before centrifugation at 1500 rpm for 10 min. After that, the suspended GCs in the middle layer were observed and transferred into a 1.5-mL RNase-free Eppendorf tube, followed by phosphate buffered saline (PBS) washing and 3 min of centrifugation at 3000 rpm. The supernatant was discarded and the suspension was centrifuged for 30 s again, with the excessive liquid removed. The cell pellets in the bottom of the tube were GCs.
Cell culture
Human ovarian GCs (KGN and SVOG) and human embryonic kidney cells HEK-293T were purchased from American Type Culture Collection (Manassas, VA, USA). All cells were cultured in a Dulbecco’s modified Eagle’s medium (DMEM) encompassing 10% fetal bovine serum (Thermo Fisher Scientific, Waltham, MA, USA), 100 U/mL penicillin, and 100 µg/mL streptomycin (Thermo Fisher Scientific) at 37 °C with 5% CO2.
Cell transfection
Logarithmically growing KGN and SVOG cells were transfected with 2 µg pcDNA3.1, pcDNA3.1-HMGA2, short hairpin RNA (sh)-negative control (NC), or sh-HMGA2 plasmids and/or 100 nM mimic NC, miR-23a-3p mimic, inhibitor NC, or miR-23a-3p inhibitor (RiboBio, Guangzhou, China). Cell transfection was performed per the manuals of the lipofectamine 2000 kit (Thermo Fisher Scientific). The transfected cells were cultured with a DMEM in an incubator at 37 °C containing 5% CO2.
3-(4, 5-Dimethylthiazol-2-yl)-2, 5-diphenyltetrazolium bromide assay
Cells were counted at 24 h, 48 h, 72 h, and 96 h after treatment. Thereafter, 100 μL cell suspensions (104–105 cells) were added into a 96-well plate with three duplicated wells and cultured in incubator at 37 °C containing 5% CO2. 3-(4, 5-Dimethylthiazol-2-yl)-2, 5-diphenyltetrazolium bromide (MTT) solution (20 μL; 5 mg/mL, Sigma, St. Louis, MO, USA) was added into each well for 4 h of culture. Next, the culture was ceased, with the culture medium discarded. Dimethyl sulfoxide (150 μL) was added into each well and gently shaken for 10 min to dissolve crystallization. Optical density values at 450 nm (OD450 values) were measured with a MTT enzyme-linked immunometric meter. MTT curves were plotted with the OD values as the X coordinate and time as the Y coordinate. The OD values were repeatedly measured three times and averaged.
Flow cytometry
The density of cell suspension was adjusted to 105 cells/mL. The 3 mL cell suspension of each specimen was placed in a centrifuge tube for 5-min centrifugation at 500 r/min, followed by the removal of the culture medium. After that, the suspension was washed with PBS and the supernatant was discarded subsequent to 5-min centrifugation at 500 r/min. Cells were resuspended with 100 μL Labeling Solution before 10-min culture at room temperature in dark. Cells were supplemented with 5 μL Annexin V-fluorescein isothiocyanate (FITC) and 5 μL propidium iodide (PI) and mixed completely, followed by 10-min reaction at room temperature in dark. FITC and PI fluorescence was measured with a flow cytometer to analyze cell apoptosis.
Reverse transcription-quantitative polymerase chain reaction (RT-qPCR)
The extracted GCs, KGN, and SVOG cells were dissolved with 1 mL Trizol (Thermo Fisher Scientific). RNA was extracted as instructed in the manuals of Trizol, quantified, and reverse-transcribed into cDNA. The reaction system was prepared per the protocols of a fluorescent quantitative PCR kit (Takara, Dalian, China), and reaction conditions were set. Real-time qPCR was carried out on the ABI7500 quantitative PCR instrument with the following reaction conditions: 10-min pre-denaturation at 95 °C and 40 cycles of 10-s denaturation at 95 °C, 20-s annealing at 60 °C, and 34-s extension at 72 °C. All primers were synthesized by GENEWIZ (Beijing, China, ). Glyceraldehyde-3-phosphate dehydrogenase (GAPDH) was utilized as the internal reference for mRNA and U6 as the internal reference for miRs in cells. The 2–ΔΔCt method [Citation24] was utilized for analysis: ΔΔCt = [Ct(target DNA) – Ct(internal reference)] experimental group – [Ct(target DNA) – Ct(internal reference)] control group.
Table 2. Primer sequences.
Western blotting
KGN and SVOG cells were washed three times with precooled PBS, lysed with protein extraction lysis buffer, and placed on ice for 30 min. Afterward, cells were centrifuged at 4 °C and 12,000 rpm for 10 min, and the supernatant was aliquoted into 0.5 mL centrifuge tubes and preserved at –20 °C. The protein was quantified with a bicinchoninic acid kit (Sigma-Aldrich), and 6 × sodium dodecyl sulfate (SDS) sample loading buffer was added to denature protein at 100 °C. The protein was separated with SDS electrophoresis and transferred into membranes with 4 °C-precooled transmembrane fluids for 1.5 h. The membranes were blocked with Tris-buffered saline with Tween 20 (TBST)-prepared 5% nonfat milk for 1 h, followed by overnight incubation at 4 °C with TBST-prepared primary rabbit antibodies (Abcam, Cambridge, UK) against GAPDH (1:10,000, ab181602), HMGA2 (1:1000, ab207301), Wnt2 (1:1000, ab109222), and β-catenin (1:5000, ab32572). After the membranes were washed three times with TBST for 10 min each time, they were incubated with goat anti-rabbit or goat anti-mouse Immunoglobulin G (1:5000, ComWin, Beijing, China) at room temperature for 2 h. Subsequent to washing with TBST and developing, the expression of proteins was tested.
Dual-luciferase reporter gene assay
The online prediction software Starbase was utilized to confirm the binding sites between miR-23a-3p and HMGA2, followed by the design of mutation (MUT) and wild-type (WT) sequences of the binding site. Thereafter, the fragments of MUT and WT sequences were cloned and introduced into pmirGLO carriers, namely MUT-HMGA2 and WT-HMGA2. After MUT-HMGA2 or WT-HMGA2 was co-transfected with miR-23a-3p mimic or miR-23a-3p inhibitor into HEK-293T cells, the cells were incubated in a DMEM at 37 °C with 5% CO2 for 48 h, followed by the measurement of the luciferase activity in cells with a dual-luciferase reporter gene kit (Promega, Madison, WI, USA).
Statistical analysis
SPSS 18.0 (IBM, Armonk, NY, USA) and GraphPad Prism 6.0 (GraphPad Software, San Diego, CA, USA) were utilized for statistical analyses. Measurement data were presented as mean ± standard deviation. The T test was utilized for comparisons between two groups, and one-way analysis of variance for comparisons among multiple groups. The correlation between miR-23a-3p and HMGA2 was analyzed with Pearson correlation coefficient. p < 0.05 was considered a statistically significant difference.
Results
MiR-23a-3p is lowly expressed while HMGA2 is highly expressed in GCs of patients with PCOS
The results of qPCR and western blotting showed that miR-23a-3p expression was obviously lower (< 0.01) and HMGA2 mRNA and protein expression was significantly higher (,C, p < 0.001; p < 0.01) in GCs of patients with PCOS versus the control patients. Pearson correlation analyses revealed a marked negative correlation between HMGA2 and miR-23a-3p expression ().
Figure 1. MiR-23a-3p is downregulated while HMGA2 is upregulated in GCs of patients with PCOS. Notes: A and B: RT-qPCR to detect the miR-23a-3p expression (A) and HMGA2 mRNA expression (B); (C) Western blotting of HMGA2 protein expression; (D) Pearson correlation analysis between HMGA2 and miR-23a-3p expression. Data were presented as mean ± standard deviation. **p < 0.01, ***p < 0.001. PCOS, polycystic ovarian syndrome; miR, microRNA; HMGA2, high-mobility group at hook 2; RT-qPCR, reverse transcription-quantitative polymerase chain reaction; GCs, granular cells.
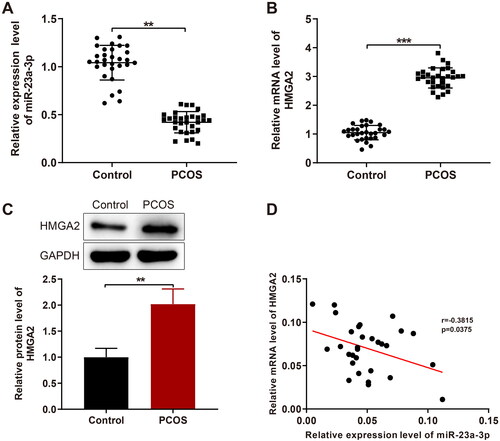
MiR-23a-3p suppresses the Wnt/β-catenin pathway to inhibit viability and promote apoptosis of GCs
MiR-23a-3p activity was mimicked or inhibited in KGN and SVOG cells. RT-qPCR results exhibited that miR-23a-3p mimic substantially increased (p < 0.001) but miR-23a-3p inhibitor declined (p < 0.01) miR-23a-3p expression in KGN and SVOG cells (). Afterward, MTT assay data demonstrated that KGN and SVOG cell viability was markedly diminished by miR-23a-3p mimic and substantially enhanced by miR-23a-3p inhibitor (,C, p < 0.05). Flow cytometry (FCM) revealed that KGN and SVOG cell apoptosis was considerably augmented by miR-23a-3p mimic and significantly lowered by miR-23a-3p inhibitor (,E, p < 0.05).
Figure 2. MiR-23a-3p causes repressed proliferation and accelerated apoptosis of GCs. Notes: MiR-23a-3p was overexpressed or silenced in KGN and SVOG cells. (A): RT-qPCR to measure the transfection efficiency; (B and C) MTT assay to assess cell viability; (D–E) FCM to determine the apoptotic rate of cells; (F–I) RT-qPCR and western blotting to test the mRNA and protein expression of Wnt2 and β-catenin in cells. Data were presented as mean ± standard deviation. *p < 0.05, **p < 0.01, ***p < 0.001. miR, microRNA; NC, negative control; GC, granular cells; RT-qPCR, reverse transcription-quantitative polymerase chain reaction; MTT, methyl thiazolyl tetrazolium; FCM, flow cytometry; mRNA, message RNA; Wnt2, wingless-type MMTV integration site family, member 2.
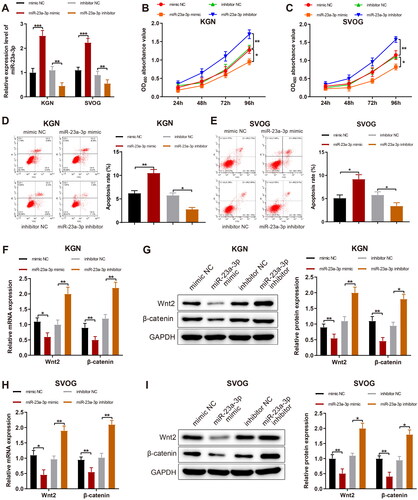
As reflected by qPCR and western blotting data, miR-23a-3p mimic prominently reduced but miR-23a-3p inhibitor markedly elevated Wnt2 and β-catenin expression in KGN and SVOG cells (I, p < 0.05).
HMGA2 induces viability and restrained apoptosis of GCs by activating the Wnt/β-catenin pathway
HMGA2 was overexpressed or silenced in KGN and SVOG cells and the transfection efficiency was tested. Results manifested that HMGA2 expression obviously increased after pcDNA3.1-HMGA2 transfection and remarkably declined subsequent to sh-HMGA2 transfection (,B, p < 0.01). Afterward, pcDNA3.1-HMGA2 substantially augmented cell viability and markedly reduced cell apoptosis, whilst sh-HMGA2 contributed to contrary trends (F, p < 0.05). With respect to qPCR and western blotting data, Wnt2 and β-catenin expression was remarkably enhanced by pcDNA3.1-HMGA2 but significantly diminished by sh-HMGA2 (J, p < 0.05).
Figure 3. HMGA2 facilitates GC proliferation and suppresses their apoptosis. Notes: HMGA2 was overexpressed or silenced in KGN and SVOG cells. (A and B): The expression of HMGA2 determined by RT-qPCR and western blotting; (C and D) The viability of cells detected by MTT assay; (E and F) The apoptotic rate of cells assessed by FCM; (G–J) RT-qPCR and western blotting to evaluate the expression of Wnt2 and β-catenin in cells. Data were presented as mean ± standard deviation. *p < 0.05, **p < 0.01, ***p < 0.001. HMGA2, high-mobility group at hook 2; sh, short hairpin; GC, granular cells; mRNA, message RNA; RT-qPCR, reverse transcription-quantitative polymerase chain reaction; MTT, methyl thiazolyl tetrazolium; FCM, flow cytometry; Wnt2, wingless-type MMTV integration site family, member 2.
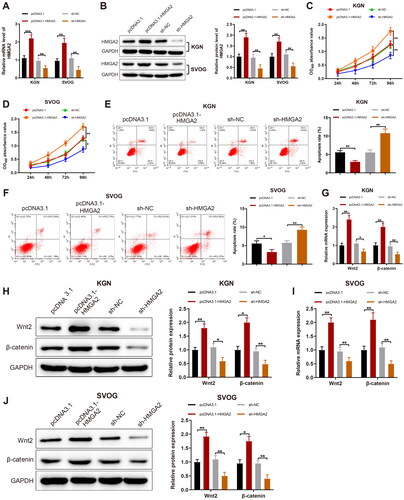
MiR-23a-3p negatively targets HMGA2
Binding sites of miR-23a-3p on HMGA2 were found through the prediction with Starbase (). Therefore, a conjecture was then proposed that miR-23a-3p modulated the proliferation and apoptosis of GCs through HMGA2. To validate the conjecture, miR-23a-3p activity was mimicked or inhibited in KGN and SVOG cells, followed by the detection of HMGA2 expression. It was exhibited that HMGA2 expression was markedly diminished by miR-23a-3p mimic and substantially upregulated by miR-23a-3p inhibitor (,C, p < 0.01). Furthermore, the dual-luciferase reporter gene assay displayed that miR-23a-3p mimic transfection signally reduced but miR-23a-3p inhibitor transfection considerably enhanced the relative luciferase activity of WT-HMGA2 (< 0.01), indicating that miR-23a-3p negatively targeted HMGA2 expression.
Figure 4. MiR-23a-3p targets and negatively mediates HMGA2 in GCs. Notes: (A) The biological online software Starbase to predict the binding site between miR-23a-3p and HMGA2 and the designed mutation site of HMGA2; (B and C) RT-qPCR and western blotting to detect expression of HMGA2 after the overexpression and inhibition of miR-23a-3p; (D) Dual-luciferase reporter gene assay to detect luciferase activity. Data were presented as mean ± standard deviation. ns, no significance, **p < 0.01, ***p < 0.001. miR, microRNA; HMGA2, high-mobility group at hook 2; GC, granular cells; NC, negative control; RT-qPCR, reverse transcription-quantitative polymerase chain reaction; mRNA, message RNA; WT, wild type; MUT, mutation.
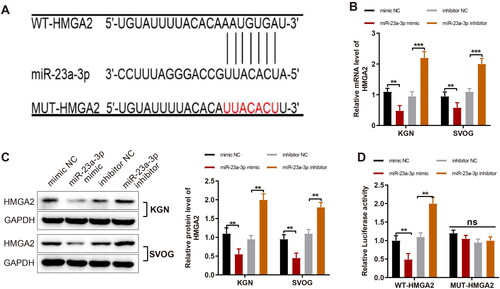
MiR-23a-3p targets HMGA2 to influence the viability and apoptosis of GCs
miR-23a-3p mimic was individually transfected or co-transfected with pcDNA3.1-HMGA2 in KGN cells, followed by the measurement of HMGA2 expression. The data manifested that HMGA2 expression significantly increased in response to pcDNA3.1-HMGA2 treatment in the presence of miR-23a-3p mimic (,B, p < 0.01). Meanwhile, the co-treatment of pcDNA3.1-HMGA2 and miR-23a-3p mimic substantially augmented viability and significantly declined apoptosis of KGN cells compared with the individual treatment of miR-23a-3p mimic, accompanied by substantially upregulated Wnt and β-catenin (F, p < 0.05).
Figure 5. MiR-23a-3p represses HMGA2 to restrain GC proliferation and facilitate their apoptosis. Notes: miR-23a-3p mimic was separately transfected or co-transfected with pcDNA3.1-HMGA2 in KGN cells: (A and B) RT-qPCR and western blotting to measure the expression of HMGA2; (C) MTT assay to assess cell viability; (D) FCM to test cell apoptosis; (E and F) RT-qPCR and western blotting measurement of the expression of Wnt2 and β-catenin in cells. Data were presented as mean value ± standard deviation. *p < 0.05, **p < 0.01, ***p < 0.001. miR, microRNA; HMGA2, high-mobility group at hook 2; GC, granular cells; NC, negative control; RT-qPCR, reverse transcription-quantitative polymerase chain reaction; mRNA, message RNA.
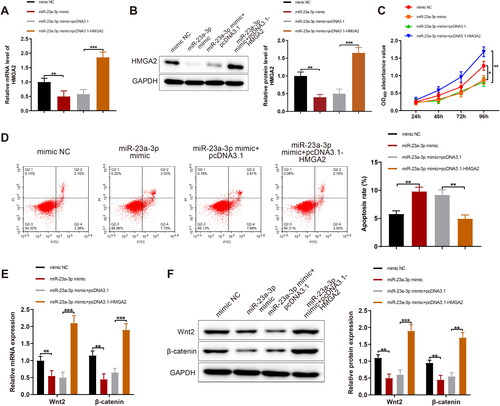
Discussion
The etiological factors of PCOS remain unclear, which causes the difficulties of accurate diagnosis and efficient therapies for patients [Citation1,Citation2]. MiR-23a-3p can modulate various tumor cells, including hepatocellular carcinoma [Citation25] and colorectal cancer [Citation26]. Nevertheless, the function of miR-23a-3p in PCOS development remains to be discussed. Hence, this research explored the expression of miR-23a-3p in GCs from patients with PCOS and its potential role and mechanism in GC proliferation and apoptosis. Our data unveiled that miR-23a-3p inhibited viability and promoted apoptosis of GCs by targeting HMGA2 and blocking the Wnt/β-catenin pathway.
Based on a prior study reporting the low expression of miR-23a in patients with PCOS [Citation13], miR-23a-3p expression was assessed in GCs of patients with PCOS in our research. Consistently, our data depicted that miR-23a-3p was downregulated in GCs of patients with PCOS. Of note, diminished GC apoptosis and augmented GC proliferation has been noted in patients with PCOS, illustrating that accelerated proliferation and repressed apoptosis of GCs play crucial roles in PCOS development [Citation5]. Therefore, GCs were selected as the subject of the following experiments. After altering miR-23a-3p expression in GCs, we noted that miR-23a-3p upregulation suppressed viability and facilitated apoptosis of GCs. Correspondingly, the research by Nie et al. elucidated that ectopic miR-23a caused an elevation in human GC apoptosis [Citation27]. Another study disclosed depressed proliferation and facilitated apoptosis after GCs were transfected with miR-23a mimic [Citation28]. Nevertheless, the function of miR-23a-3p in GCs warrants more in-depth explorations.
It has been widely recognized that miRs modulate gene expression by targeting genes [Citation29]. For instance, former research elucidated that miR-23a lowered HMGA2 expression during cellular senescence [Citation14]. Similarly, we discovered via Starbase prediction and dual-luciferase reporter gene assay that HMGA2 was inversely targeted by miR-23a-3p. In addition, RT-qPCR and western blotting results displayed the high expression of HMGA2 in GCs of patients with PCOS. Concordant with our findings, an early study unraveled that HMGA2 was highly expressed in GCs of PCOS women [Citation16]. Moreover, it was found that HMGA2 overexpression augmented GC cell viability and diminished their apoptosis in the presence of miR-23a-3p mimic. Li et al. observed in their study that the overexpression of HMGA2 enhanced the proliferation of ovarian GCs [Citation16], which was concurrent with our results. In addition, several studies demonstrated that HMGA2 played a crucial role in promoting the proliferation of ovary cancer cells [Citation30,Citation31], which synchronously implicated the exploration feasibility of the function of HMGA2 in ovarian diseases.
Furthermore, the Wnt/β-catenin pathway has been confirmed to be closely associated with PCOS progression [Citation32,Citation33]. In addition, the Wnt pathway has been documented to inhibit GC apoptosis [Citation34]. Xu et al. demonstrated that HMGA2 induced the activation of the Wnt/β-catenin pathway [Citation35]. All these exploration data indicate the necessity of measuring Wnt2 and β-catenin expression in our experiments. It was then revealed in our research that miR-23a-3p inhibition or HMGA2 overexpression contributed to the upregulation of Wnt2 and β-catenin expression in GCs.
In summary, our research unveiled that miR-23a-3p overexpression negatively targeted HMGA2 to block the Wnt/β-catenin pathway, thereby suppressing viability and facilitating apoptosis of GCs. This research offers useful research data for the further study on mechanism behind the proliferation and apoptosis of GCs in patients with PCOS. However, animal experiments were not carried out for further validation. In addition, there is still a certain gap between cell experiments and clinical application. Therefore, more in-depth studies are merited to verify the impacts of miR-23a-3p and HMGA2 on PCOS.
Author contributions
X.H. designed the study and revised the paper. P.C. instructed experiments and helped to write the paper. J.W. carried out the experiments and wrote the paper. M.K. and L.Z. contributed to analyze the results. X.H. performed transvaginal egg retrieval. S.L. and B.N. helped to collect the samples of follicular fluid. All authors discussed the results and commented on the manuscript.
Disclosure statement
The authors declare that they have no known competing financial interests or personal relationships that could have appeared to influence the work reported in this paper.
Additional information
Funding
References
- Azziz R, Carmina E, Chen Z, et al. Polycystic ovary syndrome. Nat Rev Dis Primers. 2016;2:1.
- Liu J, Ding J, Qu B, et al. CircPSMC3 alleviates the symptoms of PCOS by sponging miR-296-3p and regulating PTEN expression. J Cell Mol Med. 2020;24(18):11001–9.
- Jozkowiak M, Hutchings G, Jankowski M, et al. The Stemness of human ovarian granulosa cells and the role of resveratrol in the differentiation of MSCs – a review based on cellular and molecular knowledge. Cells. 2020;9(6):1418.
- Zhang X, Tao Q, Shang J, et al. MiR-26a promotes apoptosis of porcine granulosa cells by targeting the 3beta-hydroxysteroid-Delta24-reductase gene. Asian-Australas J Anim Sci. 2020;33(4):547–555.
- Das M, Djahanbakhch O, Hacihanefioglu B, et al. Granulosa cell survival and proliferation are altered in polycystic ovary syndrome. J Clin Endocrinol Metab. 2008;93(3):881–887.
- McAllister JM, Han AX, Modi BP, et al. miRNA profiling reveals miRNA-130b-3p mediates DENND1A variant 2 expression and androgen biosynthesis. Endocrinology. 2019;160(8):1964–1981.
- Huang X, Wu B, Chen M, et al. Depletion of exosomal circLDLR in follicle fluid derepresses miR-1294 function and inhibits estradiol production via CYP19A1 in polycystic ovary syndrome. Aging (Albany NY). 2020;12(15):15414–15435.
- Wang M, Liu M, Sun J, et al. MicroRNA-27a-3p affects estradiol and androgen imbalance by targeting Creb1 in the granulosa cells in mouse polycytic ovary syndrome model. Reprod Biol. 2017;17(4):295–304.
- Jiang X, Li J, Zhang B, et al. Differential expression profile of plasma exosomal microRNAs in women with polycystic ovary syndrome. Fertil Steril. 2021;115(3):782–792.
- Chen B, Xu P, Wang J, et al. The role of MiRNA in polycystic ovary syndrome (PCOS). Gene. 2019;706:91–96.
- Luo H, Han Y, Liu J, et al. Identification of microRNAs in granulosa cells from patients with different levels of ovarian reserve function and the potential regulatory function of miR-23a in granulosa cell apoptosis. Gene. 2019;686:250–260.
- Lozano-Bartolome J, Llaurado G, Portero-Otin M, et al. Altered Expression of miR-181a-5p and miR-23a-3p is associated with obesity and TNFα-Induced insulin resistance. J Clin Endocrinol Metab. 2018;103(4):1447–1458.
- Xiong W, Lin Y, Xu L, et al. Circulatory microRNA 23a and microRNA 23b and polycystic ovary syndrome (PCOS): the effects of body mass index and sex hormones in an Eastern Han Chinese population. J Ovarian Res. 2017;10(1):10.
- Lee S, Jung JW, Park SB, et al. Histone deacetylase regulates high mobility group A2-targeting microRNAs in human cord blood-derived multipotent stem cell aging. Cell Mol Life Sci. 2011;68(2):325–336.
- Jiao X, Chen W, Zhang J, et al. Variant Alleles of the ESR1, PPARG, HMGA2, and MTHFR genes are associated with polycystic ovary syndrome risk in a Chinese population: a case–control study. Front Endocrinol (Lausanne). 2018;9(504):1–15.
- Li M, Zhao H, Zhao SG, et al. The HMGA2-IMP2 pathway promotes granulosa cell proliferation in polycystic ovary syndrome. J Clin Endocrinol Metab. 2019;104(4):1049–1059.
- Yang Y, Jiang H, Xiao L, et al. MicroRNA-33b-5p is overexpressed and inhibits GLUT4 by targeting HMGA2 in polycystic ovarian syndrome: an in vivo and in vitro study. Oncol Rep. 2018;39(6):3073–3085.
- Ou W, Lv J, Zou X, et al. Propofol inhibits hepatocellular carcinoma growth and invasion through the HMGA2-mediated wnt/beta-catenin pathway. Exp Ther Med. 2017;13(5):2501–2506.
- Qiao GY, Dong BW, Zhu CJ, et al. Deregulation of WNT2/FZD3/beta-catenin pathway compromises the estrogen synthesis in cumulus cells from patients with polycystic ovary syndrome. Biochem Biophys Res Commun. 2017;493(1):847–854.
- Yang S, Gu Y, Wang G, et al. HMGA2 regulates acute myeloid leukemia progression and sensitivity to daunorubicin via wnt/beta-catenin signaling. Int J Mol Med. 2019;44(2):427–436.
- Rotterdam EA-SPCWG. Revised 2003 consensus on diagnostic criteria and long-term health risks related to polycystic ovary syndrome. Fertil Steril. 2004;81(1):19–25.
- Matsubara H, Ikuta K, Ozaki Y, et al. Gonadotropins and cytokines affect luteal function through control of apoptosis in human luteinized granulosa cells. J Clin Endocrinol Metab. 2000;85(4):1620–1626.
- Iwase A, Goto M, Harata T, et al. Insulin attenuates the insulin-like growth factor-I (IGF-I)-akt pathway, not IGF-I-extracellularly regulated kinase pathway, in luteinized granulosa cells with an increase in PTEN. J Clin Endocrinol Metab. 2009;94(6):2184–2191.
- Burja B, Kuret T, Janko T, et al. Olive Leaf extract attenuates inflammatory activation and DNA damage in human arterial endothelial cells. Front Cardiovasc Med. 2019;6(56):56.
- Xiang Y, Yang Y, Lin C, et al. MiR-23a-3p promoted G1/S cell cycle transition by targeting protocadherin17 in hepatocellular carcinoma. J Physiol Biochem. 2020;76(1):123–134.
- Lee Y, Kim SJ, Choo J, et al. miR-23a-3p is a key regulator of IL-17C-Induced tumor angiogenesis in colorectal cancer. Cells. 2020;9(6):1363.
- Nie M, Yu S, Peng S, et al. miR-23a and miR-27a promote human granulosa cell apoptosis by targeting SMAD5. Biol Reprod. 2015;93(4):98.
- Lin J, Huang H, Lin L, et al. MiR-23a induced the activation of CDC42/PAK1 pathway and cell cycle arrest in human cov434 cells by targeting FGD4. J Ovarian Res. 2020;13(1):90.
- Mollaei H, Safaralizadeh R, Rostami Z. MicroRNA replacement therapy in cancer. J Cell Physiol. 2019;234(8):12369–12384.
- Xing F, Song Z, He Y. MiR-219-5p inhibits growth and metastasis of ovarian cancer cells by targeting HMGA2. Biol Res. 2018;51(1):50.
- Xi YN, Xin XY, Ye HM. Effects of HMGA2 on malignant degree, invasion, metastasis, proliferation and cellular morphology of ovarian cancer cells. Asian Pac J Trop Med. 2014;7(4):289–292.
- Zheng Q, Liu M, Fu J. ALG2 inhibits the epithelial-to-mesenchymal transition and stemness of ovarian granulosa cells through the wnt/beta-catenin signaling pathway in polycystic ovary syndrome. Reprod Biol. 2022;22(4):100706.
- Ismail AB, Naji MS, Nebih I, et al. The expression profile of WNT/beta-catanin signalling genes in human oocytes obtained from polycystic ovarian syndrome (PCOS) patients. Zygote. 2022;30(4):536–542.
- Du X, Li Q, Yang L, et al. SMAD4 activates wnt signaling pathway to inhibit granulosa cell apoptosis. Cell Death Dis. 2020;11(5):373.
- Xu X, Wang Y, Deng H, et al. HMGA2 enhances 5-fluorouracil chemoresistance in colorectal cancer via the Dvl2/wnt pathway. Oncotarget. 2018;9(11):9963–9974.