ABSTRACT
In the present research, we studied the binding characterization of a single chain fragment variable (scFv) antibody S12B8 with abscisic acid (ABA) and conjugated ABA by competitive enzyme-linked immunosorbent assay (ELISA) and in silico molecular docking. The nucleotide sequences of variable light (VL) and variable heavy (VH) were obtained from hybridoma cells (F12B8), which secrete monoclonal antibodies recognizing ABA and conjugated ABA. And then the genes of VL and VH were constructed to a scFvS12B8 with a nucleotide sequence of a flexible linker (G4S)3 by splicing overlap extension PCR (SOE-PCR). The fragment of S12B8 was cloned into pMAL-c2x with a maltose binding protein tag and expressed in Escherichia coli with a calculated molecular mass of 68 kDa. The results of ELISA showed dose-dependent inhibition of the purified recombinant protein S12B8 by ABA, (ABA-ME) and ABA glucose ester tetra acetyl (ABAGE tetra acetyl). The detection limits (10% inhibition) of ABA, ABA-ME and ABAGE tetra acetyl were 19.71, 8.06 and 3.95 μg/ml, respectively. On the other hand, we investigated the interactions between S12B8 and ligands by homology modeling using RosettaAntibody and molecular docking by Discovery studio 2.5 (DS 2.5). The binding energy of ABA, ABA-ME and ABAGE when fused to the S12B8 antibody was −37.1, −69.6 and −112.0 kcal/mol, respectively, which agreed well with our competitive ELSIA results.
1. Introduction
Immunomodulation is a method that recombinant antibodies expressed ectopically in plant cells recognizes functional molecule, such as phytohormones and regulatory proteins in vivo. This method to block functional molecules directly in different compartments of plant cells could be used to study plant development and physiology (Conrad & Manteuffel, Citation2002). Nowadays, the single chain fragment variable (scFv) antibodies have been successfully applied in immunomodulation to analyze the function of plant phytohormones, such as abscisic acid (ABA), jasmonic acid (JA) and gibberellic acid (GA) (Artsaenko et al., Citation1995; ten Hoopen et al., Citation2007; Suzuki, Mizuno, Urakami, Yamaguchi, & Asami, Citation2008; Wigger et al., Citation2002). Artsaenko et al. (Citation1995) illustrated scFv antibody against ABA in tobacco plants and showed that the plants were more sensitive to drought stress for the loss of ABA function, which promotes stomatal closure and guard cell development in response to drought (Wigger et al., Citation2002). The transgenic tobacco expressing anti-JA scFv antibodies caused JA-deficient phenotypes, which were less sensitive to methyl jasmonate, thus inhibiting seed germination and wound-induced gene expression (ten Hoopen et al., Citation2007).
Although reports have proven the tremendous application potential of immunomodulation in plant physiology study, it must be pointed out that the success of such experiments depends strongly on the specificity and high affinity of the recombinant antibodies (Conrad & Manteuffel, Citation2002). Before genes of recombinant antibodies were used in immunomodulation study, the binding characterization of recombinant antibodies with their ligands should be studied (Artsaenko, Weller, Muntz, & Conrad, Citation1994; Girgis et al., Citation2011; Sartorius et al., Citation2015; Singh et al., Citation2010; Suzuki et al., Citation2005; Zhang et al., Citation2015). Methods such as enzyme-linked immunosorbent assays (ELISAs) (Artsaenko et al., Citation1994; Sartorius et al., Citation2015; Singh et al., Citation2010; Suzuki et al., Citation2005; Zhang et al., Citation2015), immunohistochemistry (Girgis et al., Citation2011) and in silico molecular docking (Fan et al., Citation2013; Feng et al., Citation2015; Yoshikawa et al., Citation2013) have been used to research the binding characterization of scFv antibodies with their ligands.
In the previous work (Liu, Citation2010), we obtained monoclonal hybridoma cells F12B8 secreting antibodies against ABA and conjugated ABA with an immunogen by coupling of carrier protein and ABA through C1 (carboxyl group). Until now, different hapten-carrier protein conjugates have been generated for ABA antibodies development. According to the previous reports, the antibodies generated by coupling ABA to carrier protein through C4’ (carbonyl group) have a high affinity and specificity for free ABA, but do not recognize ABA conjugates, such as ABA glucose ester (ABAGE), ABAGE tetra acetyl and ABA-methyl ester (ABA-ME) conjugates (Barrieu & Simonneau, Citation2000; Mertens, Deus-Neumann, & Weiler, Citation1983; Weiler, Citation1980), while the antibodies generated by coupling of ABA through C1 (carboxyl group) recognize both free ABA and ABA conjugates, which was agreed with our previous work (Perata et al., Citation1990; Weiler, Citation1980).
We try to use the scFv to study the physiology function of conjugated ABA. ABAGE is the major form of conjugated ABA in plant (Cutler & Krochko, Citation1999). It could be hydrolyzed to ABA by β-glucosidase with redundant activity genes, which have 48 members and the functions are redundant (Xu et al., Citation2004). According to previous reports, the function of ABAGE was illuminated by mutating one of β-glucosidase genes or adding exogenous ABAGE (Lee et al., Citation2006; Noguchi & Tanaka, Citation2008; Xu et al., Citation2012). However, the function of ABAGE was not totally clarified within plant development. The reported antibodies, generated with immunogen by coupling ABA with carrier protein through C1 (carboxyl group), usually have higher affinity with ABA-ME and ABAGE than that with ABA (Mertens et al., Citation1983; Perata et al., Citation1990).
In the present work, we successfully cloned the genes of variable light (VL) and variable heavy (VH) chain domains from RNA of hybridoma F12B8. And then the gene of scFv S12B8 was constructed. To improve the specificity of S12B8 antibody with conjugated ABA for immunomodulation study, we studied the binding characterizations of S12B8 antibody through ELISA using expressed and purified antibody in E. coli. A 3D model for S12B8 was constructed by RosettaAntibody server (Sircar, Kim, & Gray, Citation2009). The binding complexes of the 3D model and the ligands were simulated by using CDOCKER in Discovery Studio™ 2.5 (DS, Accelrys, San Diego, CA, USA) environment (Wu, Robertson, Brooks, & Vieth, Citation2003).
2. Materials and methods
2.1. Hybridoma, plasmid and bacteria strain
Murine monoclonal hybridoma cells F12B8 were obtained in our previous study (Liu, Citation2010). E. coli DH5α and plasmid pMD-18T simple vector were purchased from Takara (Beijing, China). Bacterial expression plasmid pMAL-c2x and expressed bacteria strain TB1 (NEB, America) were kindly provided by Dr Tao Wang at China Agricultural University.
2.2. PCR amplification of VH and VL
mRNA was extracted from 5 × 106 of F12B8 hybridoma cells using an mRNA purification kit (Huayueyang, China) according to the manufacturer's instruction (Essono, Frobert, Grassi, Creminon, & Boquet, Citation2003). First-strand cDNA was synthesized by Moloney Murine Leukemia Virus reverse transcriptase (Takara, China). The mixture containing 5 μl of RNA (2 μg), 1.25 μl of dNTP, 2.5 μl of primers oligo (dT) and 9.6 μl of RNase-free water was heated at 70 °C for 5 min. Five microliter of MLV reverse transcriptase enzyme (1 U/Al) and 0.625 μl of RNase inhibitor (1 U/Al) were added to the mixture subsequently. Then the reverse transcription was carried out at 42 °C for 60 min. The cDNA products were kept at −20 °C.
The VH and VL genes were amplified by PCR with degenerate primers (Krebber et al., Citation1997; Wang et al., Citation2000) and KOD-PLUS DNA polymerase (Toyobo, Japan). The PCR reactions for VH or VL in 50 μl volume contained 2 μl of first-stand cDNA, 1 μl of variable heavy chain forward primer (VHF) (or VLF) (), 1 μl of VHR (or VLR) (), 200 μM dNTPs, 0.8–1.2 mM MgCl2 and 5 μl of 10× concentrated buffer. PCR was performed as follows: heating at 94 °C for 2 min, followed by 30 cycles of 94 °C for 15 s, 60 °C for 30 s and 68 °C for 1 min, and then the final elongation at 68 °C for 7 min. PCR products were analyzed in 1% agarose gel. The four framework regions (FRs) and three complementarity-determining regions (CDRs) of VH and VL were analyzed according to the Chothia scheme (http://www.bioinf.org.uk/abs/) (Abhinandan & Martin, Citation2008).
Table 1. Primer sequences of SOE-PCR.
2.3. Assembly of S12B8 gene
PCR products of VH and VL were purified using the Tiangel Midi purification kit (Tiangel, China). VH and VL were fused into a single DNA fragment via a flexible linker (Horton, Hunt, Ho, Pullen, & Pease, Citation1989) () by SOE-PCR (McCafferty, Griffiths, Winter, & Chiswell, Citation1990). Firstly, 8 ng of purified VH and VL products was mixed with 400 µM dNTP, 5 µL of 10× PCR buffer, 0.5 U of KOD-plus DNA polymerase and 40 µL of water. The mixture was heated at 94 °C for 2 min, followed by 10 cycles (94 °C for 15 s, 55 °C for 60 s and 68 °C for 1 min) and a final elongation at 68 °C for 7 min. Then, we amplified the assembled products with the addition of 0.5 μl of DNA polymerase and 2 μM of the VHF and VLR primers. The PCR program was as follows: 94 °C for 2 min, 30 cycles of 94 °C for 15 s, 68 °C for 1 min, 68 °C for 1 min and a final elongation at 68 °C for 7 min. PCR products were purified and cloned into the pMD-18T vector according to the manufacturer's instruction. Positive clones were identified by PCR and then sequenced (Invitrogen, USA).
2.4. Expression of recombinant S12B8 antibody in E. coli
To express scFv S12B8, the digested scFv S12B8 fragment was gel-purified after cleavage by EcoRI and HindIII for 3 h at 37 °C, and then the purified scFv S12B8 fragment was subcloned into pMAL-c2x with a maltose binding protein (MBP) tag at the N-terminus of the recombinant antibody. The MBP tag can improve solubility, stable and does not disrupt the antibody activities of recombinant protein (Vaks & Benhar, Citation2014; Zhao, Cascio, Sawaya, & Eisenberg, Citation2011). The construction pMAL-c2x-S12B8 was transformed into E. coli strain TB1 by heat-shock for cytoplasmic expression. Clones were selected on Luria-Bertani broth (LB) plate with 100 μg/ml ampicillin and screened by PCR using VHF and VLR primers (). Fusion proteins were expressed and extracted according to the pMAL™ protein fusion and purification manual. Briefly, a single colony of transformed cells was inoculated in 5 mL LB (1% tryptone, 0.5% yeast extract, 0.5% NaCl, 0.2% glucose) containing 100 μg/mL ampicillin at 30 °C with shaking at 200 rpm, overnight. 1.2 ml of the overnight culture was transferred into 120 ml LB containing 100 μg/ml of ampicillin and continued shaking at 30 °C until optical density at 600 nm (OD600) reaches mid-log phase (0.6–0.8). Twenty milliliter of culture was divided into 6 sterile triangular flasks and continued shaking at 20 °C for 5 h after adding with isopropyl β-D-1-thiogalactopyranoside (IPTG) at the final concentration of 0, 0.1, 0.3, 0.5, 0.7 and 1 mM, respectively. We choose the lower temperature 30 °C and 20 °C in induction stage than the commonly 37 °C to improve the solubility of recombinant protein based on laboratory manual (Sambrook and Russell, Citation2005). Cells were harvested by centrifugation at 6000×g for 10 min at 4 °C, resuspended in 100 µL 1× SDS PAGE loading buffer [50 mM Tris-HCl, pH 6.8; 2% sodium dodecyl sulfate (SDS); 0.1% bromophenol blue; 10% glycerinum; 1% β-mercaptoethanol (β-ME)], heated at 100 °C for 3 min and detected by SDS polyacrylamide gel electrophoresis (SDS–PAGE). For the soluble analysis, the pellet was resuspended in 1 ml of column buffer (20 mM Tris-HCl, 200 mM NaCl, 1 mM EDTA and 10 mM β-ME), followed by sonication for 35 cycles in 3 s short pulses and 5 s pause in an ice-water bath. Centrifugation at 12,000×g at 4°C for 15 min, supernatant (soluble fraction) and inclusion body were obtained and detected by SDS–PAGE. To optimize scFv expression, we tested induction time of 0, 4, 8 and 16 h. Gray value of SDS–PAGE spectrogram was analyzed by ImageJ 1.48v.
2.5. Purification of recombinant S12B8 antibody
The induced, cultured E. coli cells of 500 ml were centrifuged at 4°C for 10 min at 6000×g. The pellet was resuspended in 25 ml of column buffer, followed by sonication for 35 cycles in 3 s short pulses and 5 s pauses in an ice-water bath. After centrifugation at 12,000×g at 4 °C for 15 min, the supernatant protein was purified at 4 °C by amylase affinity chromatography with the elution buffer of column buffer contained 10 mM maltose (Amresco, USA). The protein purified by affinity chromatography was collected with fractions of 500 µL, and each fraction was detected by SDS–PAGE. The fractions containing purified scFv antibody were combined and concentrated with an Amicon Ultra-15 Centrifugal Filter Units, 30 kD (Millipore, America). The purified scFv S12B8 kept at 4 °C was used for binding activity assay within seven days. Protein concentrations were determined by the Bradford assay during purification.
2.6. Analysis of S12B8 antibody activity
ELISA measurements were carried out to determine the antigen binding activity of scFv S12B8 antibody. The noncompetitive ELISA was applied to assay the binding activity of scFv S12B8 antibody with antigen ABA-C1-BSA. Briefly, the antigen ABA-C1-BSA diluted in 0.05 M carbonate buffer (pH 9.6) was coated on microtiter plates at 37 °C for 3 h. The purified S12B8 antibody was diluted into concentrations of 50, 20, 10, 5, 2, 1, 0.5 and 0.25 µg/mL and added to the detection wells of microtiter plates. MBP protein was added to control wells simultaneously as control. After 30 min incubation at 37 °C, bound scFv was detected by anti-MBP rabbit antibody (NEB, America) and anti-rabbit immunoglobulin conjugated to horseradish peroxidase (HRP). The enzymatic reaction was performed at 37 °C for 30 min, and detected with o-Phenylenediamine (OPD) as a substrate. The absorbance at 492 nm was measured after incubation for 10 min.
The competitive ELISA was applied to compare the binding activity of scFv S12B8 antibody with ligand of (+)-ABA, ABA-ME and ABAGE tetra acetyl, which was synthesized from ABA and α-bromo-d-glucose according to Zhou et al. (Citation2012). Microtiter plates were coated with ABA-C1-BSA as the noncompetitive ELISA. Then 50 µL of expressed scFv S12B8 and 50 µL of ABA (or conjugated ABA) were added into the microtiter plate wells. After 30 min of incubation at 37 °C, the detection by anti-MBP antibody and anti-rabbit immunoglobulin conjugated to HRP and subsequence OPD reaction were performed as the noncompetitive ELISA. The detection limit (10% inhibition) was analyzed by Origin8.0 software.
2.7. In silico analysis of S12B8 antibody binding with the ligands
The homology modeling of S12B8 was performed using RosettaAntibody server (http://antibody.graylab.jhu.edu). The full RosettaAntibody protocol, which additionally models the hyper-variable CDR H3 loop, was executed for high-resolution model. CDR H3 that lies in the center of the antigen-binding site plays a pivotal role in antigen recognition and is the hardest to be predicted due to its diversity in structure, length and sequence composition (Das & Baker, Citation2008; Morea, Lesk, & Tramontano, Citation2000; Kuroda, Shirai, Kobori, & Nakamura, Citation2008). CDR H3 loop conformation was predicted by Rosetta suite (Das & Baker, Citation2008). The high-resolution models generated by RosettaAntibody have been used for the successful prediction of antibody–antigen complex structures (Sircar et al., Citation2009).
The molecular docking procedure was performed using CDOCKER in DS 2.5 (Wu et al., Citation2003). CDOCKER, a docking algorithm based on molecular dynamics (MD) and a CHARMM force field, indeed is an optimization technique for molecular docking (Pearce, Langley, Kang, Huang, & Kulkarni, Citation2009). The ligand molecules of ABA, ABA-ME and ABAGE () were visualized by PubChem (http://www.ncbi.nlm.nih.gov/pccompound) and ChemBioOffice 2010 (Kerwin, Citation2010). The steric conformations and distances of ABA, ABA-ME and ABAGE were displayed by DS 2.5. The CHARMM force field was used for the molecular dynamics simulation and the binding energy minimization in the docking process (Brooks et al., Citation1983). The binding sites of receptor S12B8, predicted using the binding site tool in DS 2.5, which is the nearest to the CDRs was selected. The CDRs recognize a wide range of antigens and form a hydrophobic cavity by the solvent-accessible surface of 3D model (Messih, Lepore, Marcatili, & Tramontano, Citation2014). The sphere radius of binding site was determined according to the distances of ligands (), and it was set as 9.5 Å for ABA-ME and ABA, 16 Å for ABAGE. An energy minimized structure containing S12B8 was used as the receptor for substrate docking. The pose cluster radius was set as 0.5. The structures of the protein, ligands and their complexes were subjected to energy minimization using the CHARMM force field implemented in DS 2.5. The protein structure was kept rigid, and the ligand structures were flexible in the protein–substrate complex. For each ligand, 800 replicas were generated and randomly distributed around the center of the active site during every single docking run. Molecular dynamics simulations of S12B8 and ligands were performed using the standard dynamics cascade protocol in DS 2.5. Finally, docking poses were ranked according to the total docking (CDOCKER) energy including the intra-molecular energy for the ligands and the ligand–protein interactions, and the lowest energy structure was used for post-docking analysis and binding energy calculation.
3. Results and discussion
3.1. Sequence analysis of VH and VL and assembly of S12B8 scFv gene
The nucleotide and amino acid sequences of S12B8 with the CDRs and FRs, which based on key residues present in CDRs and FRs, are shown in . The VH gene contained 363 nucleotides, and encoded 121 amino acids. CDR H1 and CDR H2 are similar to class 1 and 4, respectively (Chothia et al., Citation1989). VH belongs to subgroup III. The VL gene included 333 nucleotides, and encoded 111 amino acids. Both CDR L2 and CDR L3 belong to canonical class 1. VL belongs to subgroup IV. These results suggest that the nucleotide sequences of S12B8 are correct. VH and VL genes were fused with a flexible linker (G4S)3, and then formed scFv S12B8 (VH-linker-VL) gene with 750 bp ().
3.2. Expression of S12B8 antibody
The S12B8 antibody protein was induced and expressed by using IPTG concentrations of 0, 0.1, 0.3, 0.5, 0.7 and 1.0 mM. A distinct protein band of approximately 68 kDa could be identified compared with the non-induced cells (0 mM, (a)). The gray values indicated that the S12B8 expression with IPTG concentrations of 0.1, 0.3, 0.5, 0.7 and 1.0 mM were 226%, 353%, 285%, 246% and 251% of that with 0 mM, respectively, which showed that the optimization of IPTG concentration for S12B8 expression in E. coli was 0.3 mM. The gray value of the expressed supernatant was approximately 70% of the total expressed scFv antibody, and inclusion body protein was only 30% ((b)). With different induction times ((c)), the gray value of the recombinant antibody reached 76.5% and 90.2% with the induction time of 4 h and 8 h respectively, compared to that of 16 h. The amount of recombinant antibody expression increased to 13.7% from 4 to 8 h, while the same increased to only 9.8% from 8 to 16 h. Considering the expression amount and time consumed, induction time of 8 h was chosen for S12B8 induction expression. The expression of recombinant antibody accounted for 14.5% of the total soluble protein by gray value analysis.
Figure 4. SDS–PAGE of optimization recombinant protein expression and purification. As shown by the arrows, recombinant S12B8 scFv size was approximately 68 kDa. M: Marker 10–170 kDa. (a) Overexpression of S12B8 scFv at various IPTG concentrations (0, 0.1, 0.3, 0.5, 0.7 and 1 mM). (b) Solubility of recombinant protein. (c) Overexpression of S12B8 scFv at different induction time (4, 8 and 16 h). (d) Purification of recombinant protein.
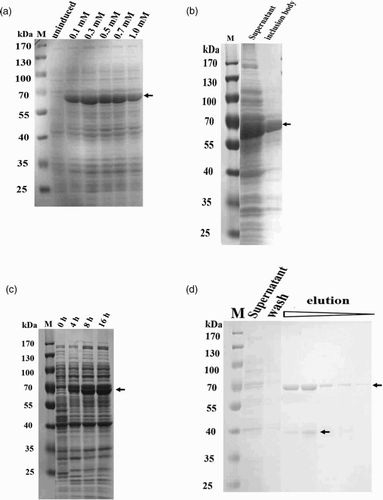
3.3. Purification of S12B8 antibody
The recombinant S12B8 antibody was purified by affinity chromatography with an amylose column. The elution solution was collected and detected by SDS–PAGE ((d)), which showed that the purified protein had a molecular weight of 68 kDa. A protein band of approximately 40 kDa was observed ((d)), which was the MBP protein (tag protein) hydrolyzed from the purified protein.
3.4. S12B8 activity analysis
For binding activity assay of S12B8 scFv antibody with antigen, the purified S12B8 protein was diluted to concentrations of 50, 20, 10, 5, 2, 1, 0.5 and 0.25 µg/mL with noncompetitive ELISA. The results showed that the S12B8 scFv antibody has binding activity with the antigen ABA-C1-BSA ((a)). The MBP protein was set as control and there was no binding activity ((a)).
Figure 5. Binding characterization of scFv S12B8 to the antigen ABA-C1-BSA, ABA ABA-ME and ABAGE tetra acetyl. (a) Antigen binding activity of scFv S12B8 antibody and MBP protein by noncompetitive ELISA. (b) Binding characterization of scFv S12B8 to ABA, ABA-ME and ABAGE tetra acetyl by competitive ELISA.
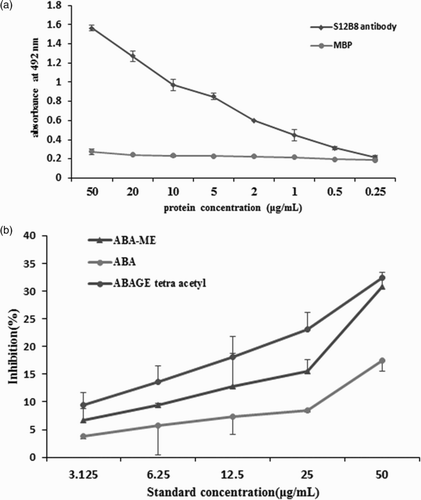
Competitive ELISAs were employed to analyze the scFv S12B8 binding character to the ligand ABA and conjugated ABA. The results of dose-dependent inhibition rates of conjugated ABA and ABA are observed in (b). The highest concentration that could be tested was 50 µg/ml of conjugated ABA or ABA. The detection limits (10% inhibition) of ABA, ABA-ME and ABAGE tetra acetyl are 19.7, 8.1 and 4.0 μg/ml, respectively. The inhibition rate of ABA-ME and ABAGE tetra acetyl was approximately 1.7- and 2.4-fold stronger than ABA.
3.5. Homology modeling and model validation
Ten top-scoring models were generated by the RosettaAntibody server with state-of-the-art algorithms. For the docking study, the model with the highest score was validated using PROCHECK (http://www.ebi.ac.uk/pdbsum/) (Laskowski, MacArthur, Moss, & Thornton, Citation1993) and verify3D (http://services.mbi.ucla.edu/Verify3D/) (Bowie, Luthy, & Eisenberg, Citation1991) to confirm its consistency. Results of Ramachandran Plot showed 87.6% of residues in the most favored regions, 10.8% in additionally allowed regions and 1.5% of residues in generously allowed regions. Altogether, 98.4% of the residues were placed into the combination of favored and allowed categories. Therefore, PROCHECK validated the folding integrity of the model and indicated that the model structure generated by RosettaAntibody was of higher quality in terms of protein folding. Verify3D profile for the final structure showed that 98.2% of the residues had an average 3D–1D score greater than or equal to 0.2, which indicated the simulated structure is reliable and accurate.
3.6. Docking study
Homology modeling techniques have been known to support the protein–ligand interaction, especially, with respect to substrate specificity and substrate recognition (Fan et al., Citation2014; McRobb, Capuano, Crosby, Chalmers, & Yuriev, Citation2010). For docking of S12B8 to each ligand, ABA, ABA-ME and ABAGE, 800 replicas were generated and randomly distributed around the center of the active site during every single docking run. Finally, docking poses were ranked based on the total docking (CDOCKER) energy including the intra-molecular energy for the ligands and the ligand–protein interactions, and the lowest energy structure was used for post-docking analysis ((a)). The binding energy of ABA, ABA-ME and ABAGE when fused to S12B8 was −37.1, −69.6 and −112.0 kcal.mol–1, respectively. The binding energy rates of ABA-ME/ABA and ABAGE/ABA to S12B8 were 1.87 and 3.0, which agreed well with our competitive ELISA results (1.7- and 2.4-fold).
Figure 6. The 3D binding poses and 2D representations of ABA and ABA-ME with S12B8. (a) The 3D binding poses of ABA (blue) and ABA-ME (magenta). (b) 2D representation of the ABA with the S12B8 model. (c) 2D representation of the ABA-ME with the S12B8 model. The residues involved in various events are represented as following. Hydrogen bond, charge or polar interactions (magenta-colored circles), van der Waals (green circles), hydrogen-bond interactions with amino acid side chains (blue dashed line). The distances shown are in Å.
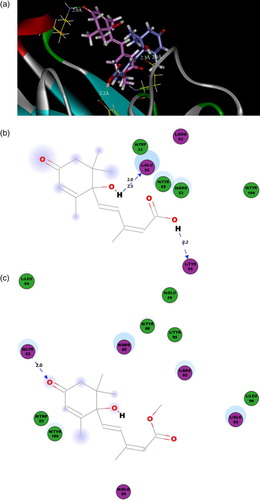
According to the docking result ((a)), the ABA and ABA-ME conjugate to the S12B8 in the cave with different orientation, which the cyclohexene ring of ABA exposed to cave edge, while that of ABA-ME embedded in the cave. The ABA adjoins to the loop of CDR L3, and there are three hydrogen bonds conjugated the two residues (CDR L3: Glu93-C1'OH, 2.0 Å, CDR L3: Glu93-C1'OH, 2.3 Å and CDR L3: Tyr95-C1OH, 2.2 Å). However, the ABA-ME molecule was more close to the loop of CDR H2, and only one hydrogen bond was observed to conjugate the ABA-ME and S12B8 (CDR H2: Lys53-C4'O, 2.0 Å). In addition to the hydrogen bonding, the polar interaction also participated in the binding of S12B8 and the ligands ((b and c)). Only one polar interaction was found in ABA-S12B8 (CDR L3: Arg92), but there are four in ABA-ME-S12B8 (CDR H2: Arg52, CDR L3: Arg92, CDR L3: Glu93 and CDR H2: Glu50). The difference between van der Waals of ABA-S12B8 (six residues) and ABA-ME-S12B8 (five residues) was not as significant as hydrogen bond or polar interaction ().
Table 2. The detail results of amino acid residues in molecular docking.
The binding energy of ABAGE when fused to S12B8 was −112.0 kcal mol–1, which was stronger than that of ABA-ME (−69.6 kcal mol–1). The binding orientation of ABA-ME and ABAGE with S12B8 was similar (). The cyclohexene ring embedded in the cave, resulting in a hydrogen bond between the Arg92 of S12B8 CDR L3 and carbonyl (2.2 Å). But for the larger size and polarity of glucose ester, the ABAGE had three more hydrogen bonds than ABA-ME (CDR H2: Tyr58 – C1O, 2.1 Å, CDR H2: Arg52 – C1O, 2.4 Å, CDR H2: Arg52 – glucose ester O, 2.3 Å, CDR H2: Arg52 – glucose ester OH 2.1 Å, CDR H2: Glu50 – glucose ester OH 2 Å and CDR L3: Glu93 – glucose ester OH, 2 Å). Four polar interaction residues were found in ABA-ME-S12B8, but there are only two in ABAGE-S12B8 (CDRL3: Leu94, CDRL3: Tyr95). Three van der Waals residues were found in ABAGE-S12B8, which also less than that of ABA-ME-S12B8 (five residues) ().
Figure 7. The 3D binding poses and 2D representations of ABAGE with S12B8. (a) The 3D binding poses of ABA-ME (magenta) and ABAGE (blue). (b) 2D representation of the ABAGE with the S12B8 model. The residues involved in various events are represented as following. Hydrogen bond, charge or polar interactions (magenta-colored circles), van der Waals (green circles), hydrogen-bond interactions with amino acid side chains (blue dashed line). The distances shown are in Å.
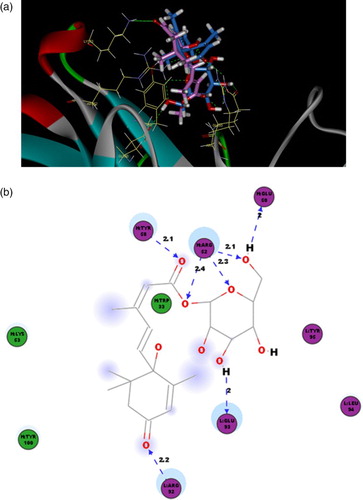
The CDR H1, H2, H3 and L3 are important for S12B8 conjugation with both ABA and conjugated ABA. All residues forming intermolecular forces contained in these CDRs, of which, the Trp33 in CDR H1, Glu50, Arg52, Tyr58 in CDR H2, Tyr100 in CDR H3 and Arg92, Glu93, Leu94, Tyr95 in CDR L3 are responsible for both three ligand conjugations, even though in different force type. The residue Lys53 in CDR H2 was applied in ABA-ME-S12B8 and ABAGE-S12B8 and not observed in the ABA-S12B8. The binding energy rate of ABAGE/ABA to S12B8 was 3, and it revealed that the binding affinity of ABAGE with S12B8 was far stronger than that of ABA. According to the binding energy, the highest binding affinity of S12B8 was ABAGE, then ABA-ME.
4. Discussion
Many site-directed mutagenesis experiments were confirmed based on homology modeling and molecular docking (Labrecque et al., Citation2011; Yang et al., Citation2012). Indeed, residue replacement resulted in the changes of specificity (Baharum et al., Citation2013). Our goal was to improve the specificity between conjugated ABA (ABA-ME and ABAGE) and scFv S12B8 and reduce the binding affinity between ABA and scFv S12B8. The CDR L3: Tyr 95 formed HB with carboxyl of ABA to S12B8, but did not have an important role of conjugated ABA (). So, we predicted the CDR L3: Tyr95 to mutate hydrophobicity Ala95, which cannot form hydrogen bond with carboxyl of ABA. So, binding affinity between ABA and scFv S12B8 may/would be reduced and the binding affinity of conjugated ABA with scFv S12B8 may/would be changed slightly, and finally, the specificity of conjugated ABA with scFv S12B8 may be improved.
5. Conclusion
In the present work, we cloned VH and VL genes of mAb F12B8 and fused with a flexible linker to allow for successful expression of the scFv fusion antibody in E. coli. The supernatant containing the soluble scFv was purified and then used to develop ELISAs for analysis of binding characterization, which showed that the S12B8 could conjugate to conjugated ABA and ABA. The detection limits (10% inhibition) of ABA, ABA-ME and ABAGE tetra acetyl were 19.7, 8.1 and 4.0 μg/ml, respectively. The inhibition rate of ABA-ME and ABAGE tetra acetyl was approximately 1.7- and 2.4-fold stronger than ABA. And then the molecular docking of S12B8 with ABA and conjugated ABA was performed by CDOCK in DS 2.5. The binding energy of ABA, ABA-ME and ABAGE when fused to the S12B8 antibody was −37.1, −69.6 and −112.0 kcal/mol, respectively, which agreed well with the ELISA results. The binding affinity of ABAGE with S12B8 was far stronger than that of ABA. In conclusion, we predicted the CDR L3: Tyr 95, which formed hydrogen bond with carboxyl of ABA to S12B8 but did not have an important role of conjugated ABA, to mutate hydrophobicity Ala95. This prediction may improve the specificity of conjugated ABA with scFv S12B8.
Acknowledgments
The authors are grateful to Prof. Qing X. Li (Department of Molecular Biosciences and Bioengineering, University of Hawaii, Mānoa, USA) for critically correcting the manuscript.
Disclosure statement
No potential conflict of interest was reported by the authors.
Notes on contributors
Xiaojiao Chen obtained her bachelor’s degree from China Agricultural University, Beijing, China in 2011. Now she is a PhD student in immunomodulation of plant hormones at China Agricultural University, Beijing, China.
Yao Lu obtained her bachelor’s degree and PhD degree in crop science from China Agricultural University, Beijing, China in 2005 and 2013, respectively. Her research interest is plant hormone immunoassay.
Guiyu Tan obtained her bachelor’s degree and PhD degree in crop science from China Agricultural University, Beijing, China in 2008 and 2013, respectively. Her research interest includes immunoassay and engineered antibody.
Zhen Cao obtained his bachelor’s degree from Huazhong Agricultural University, Wuhan, China in 2009 and his PhD degree in immunoassay from China Agricultural University, Beijing, China in 2013. Now he is postdoctoral in University of Hawaii, Honolulu, USA. His research interest is immunoassay.
Wei Liu obtained his bachelor’s degree from HuBei University, Wuhan, China in 2003 and his PhD degree in immunoassay from China Agricultural University, Beijing, China in 2010. His research interest is plant hormone immunoassay.
Baomin Wang obtained his bachelor’s degree and PhD degree in crop science from China Agricultural University, Beijing, China in 1989 and 1998, respectively. His research interest includes immunoassay and environment risk assessment of transgenic plants.
Mingcai Zhang obtained his bachelor’s degree and PhD degree in crop science from China Agricultural University, Beijing, China in 1998 and 2004, respectively. His research interest is crop physiology.
Zhaohu Li obtained his bachelor’s degree from China Agricultural University, Beijing, China in 1987 and his PhD degree in weed science from Auburn University, Alabama, USA in 2001. His research interest includes crop physiology and plant growth regulator.
Additional information
Funding
References
- Abhinandan, K. R., & Martin, A. C. R. (2008). Analysis and improvements to Kabat and structurally correct numbering of antibody variable domains. Molecular Immunology, 45(14), 3832–3839. doi:10.1016/j.molimm.2008.05.022
- Artsaenko, O., Peisker, M., Zurnieden, U., Fiedler, U., Weiler, E. W., Muntz, K., & Conrad, U. (1995). Expression of a single-chain Fv antibody against abscisic-acid creates a wilt phenotpe in transgenic tobacco. The Plant Journal, 8, 745–750. doi:10.1046/j.1365-313X.1995.08050745.x
- Artsaenko, O., Weller, E. W., Muntz, K., & Conrad, U. (1994). Construction and functional characterization of a single chain Fv antibody binding to the plant hormone abscisic acid. Journal of Plant Physiology, 144(3), 427–429. doi:10.1016/S0176-1617(11)81209-5
- Baharum, H., Chu, W. C., Teo, S. S., Ng, K. Y., Rahim, R. A., & Ho, C. L. (2013). Molecular cloning, homology modeling and site-directed mutagenesis of vanadium-dependent bromoperoxidase (GcVBPO1) from Gracilaria changii (Rhodophyta). Phytochemistry, 92, 49–59. doi:10.1016/j.phytochem.2013.04.014
- Barrieu, P., & Simonneau, T. (2000). The monoclonal antibody MAC252 does not react with the (–) enantiomer of abscisic acid. Journal of Experimental Botany, 51, 305–307. doi:10.1093/jexbot/51.343.305
- Bowie, J. U., Luthy, R., & Eisenberg, D. (1991). A method to identify protein sequences that fold into a known three-dimensional structure. Science, 253, 164–170. doi: 10.1126/science.1853201
- Brooks, B. R., Bruccoleri, R. E., Olafson, B. D., States, D. J., Swaminathan, S., & Karplus, M. (1983). CHARMM: A program for macromolecular energy, minimization, and dynamics calculations. Journal of Computational Chemistry, 4, 187–217. doi:10.1002/jcc.540040211
- Chothia, C., Lesk, A. M., Tramonontano, A., Levitt, M., Smithgill, S. J., Air, G., … Poljak, R. J. (1989). Conformations of immunoglobulin hypervariable regions. Nature, 342, 877–883. doi:10.1038/342877a0
- Conrad, U., & Manteuffel, R. (2002). Immunomodulation of phytohormones and functional proteins in plant cells. Trends in Plant Science, 6, 399–402. doi:10.1016/S1360-1385(01)02043-X
- Cutler, A. J., & Krochko, J. E. (1999). Formation and breakdown of ABA. Trends in Plant Science, 4, 472–478. doi:10.1016/S1360-1385(99)01497-1
- Das, R., & Baker, D. (2008). Macromolecular modeling with Rosetta. Annual Review of Biochemistry, 77, 363–382. doi:10.1146/annurev.biochem.77.062906.171838
- Essono, S., Frobert, Y., Grassi, J., Creminon, C., & Boquet, D. (2003). A general method allowing the design of oligonucleotide primers to amplify the variable regions from immunoglobulin cDNA. Journal of Immunological Methods, 279, 251–266. doi:10.1016/S0022-1759(03)00242-4
- Fan, H., Hitchcock, D. S., Seidel, R. D., Hillerich, B., Lin, H., Almo, S. C., … Raushel, F. M. (2013). Assignment of pterin deaminase activity to an enzyme of unknown function guided by homology modeling and docking. Journal of the American Chemical Society, 135, 795–803. doi:10.1021/ja309680b
- Fan, S., Xu, Y., Li, X., Tie, L., Pan, Y., & Li, X. (2014). Opposite angiogenic outcome of curcumin against ischemia and Lewis lung cancer models: In silico, in vitro and in vivo studies. Biochimica et Biophysica Acta, 1842, 1742–1754. doi:10.1016/j.bbadis.2014.06.019
- Feng, Z., Pearce, L. V., Xu, X., Yang, X., Yang, P., Blumberg, P. M., & Xie, X. Q. (2015). Structural insight into tetrameric hTRPV1 from homology modeling, molecular docking, molecular dynamics simulation, virtual screening, and bioassay validations. Journal of Chemical Information and Modeling, 55, 572–588. doi:10.1021/ci5007189
- Girgis, M. D., Olafsen, T., Kenanova, V., McCabe, K. E., Wu, A. M., & Tomlinson, J. S. (2011). Targeting CEA in pancreas cancer xenografts with a mutated scFv-Fc antibody fragment. European Journal of Nuclear Medicine and Molecular Imaging, 1, 24–34. doi:10.1128/AEM.01441-10
- ten Hoopen, P., Hunger, A., Müller, A., Hause, B., Kramell, R., Wasternack, C., … Conrad, U. (2007). Immunomodulation of jasmonate to manipulate the wound response. Journal of Experimental Botany, 58, 2525–2535. doi:10.1093/jxb/erm122
- Horton, R. M., Hunt, H. D., Ho, S. N., Pullen, J. K., & Pease, L. R. (1989). Engineering hybrid genes without the use of restriction enzymes: Gene splicing by overlap extension. Gene, 77, 61–68. doi:10.1016/0378-1119(89)90359-4
- Kerwin, S. M. (2010). ChemBioOffice ultra 2010 suite. Journal of the American Chemical Society, 132, 2466–2467. doi:10.1021/ja1005306
- Krebber, A., Bornhauser, S., Burmester, J., Honegger, A., Willuda, J., Bosshard, H. R., & Pluckthun, A. (1997). Reliable cloning of functional antibody variable domains from hybridomas and spleen cell repertoires employing a reengineered phage display system. Journal of Immunological Methods, 201, 35–55. doi:10.1016/S0022-1759(96)00208-6
- Kuroda, D., Shirai, H., Kobori, M., & Nakamura, H. (2008). Structural classification of CDR-H3 revisited: A lesson in antibody modeling. Proteins: Structure, Function, and Bioinformatics, 73, 608–620. doi:10.1002/prot.22087
- Labrecque, J., Metz, M., Lau, G., Darkes, M. C., Wong, R. S. Y., Bogucki, D., & Skerlj, R. T. (2011). HIV-1 entry inhibition by small-molecule CCR5 antagonists: A combined molecular modeling and mutant study using a high-throughput assay. Virology, 413, 231–243. doi:10.1016/j.virol.2011.02.016
- Laskowski, R. A., MacArthur, M. W., Moss, D. S., & Thornton, J. M. (1993). PROCHECK: A program to check the stereochemical quality of protein structures. Journal of Applied Crystallography, 26, 283–291. doi:10.1107/S0021889892009944
- Lee, K. H., Piao, H. L., Kim, H. Y., Choi, S. M., Jiang, F., Hartung, W., & Hwang, I. (2006). Activation of glucosidase via stress-induced polymerization rapidly increases active pools of abscisic acid. Cell, 126, 1109–1120. doi:10.1016/j.cell.2006.07.034
- Liu, W. (2010). Preparation of monoclonal antibodies against abscisci acid and conjugated abscisic acid as well as preliminary study on immunoassay techniques for transgenic EPSPS soybean. Beijing: China Agricultural University.
- McCafferty, J., Griffiths, A. D., Winter, G., & Chiswell, D. J. (1990). Phage antibodies: Filamentous phage displaying antibody variable domains. Nature, 348, 552–554. doi:10.1038/348552a0
- McRobb, F. M., Capuano, B., Crosby, I. T., Chalmers, D. K., & Yuriev, E. (2010). Homology modeling and docking evaluation of aminergic G protein-coupled receptors. Journal of Chemical Information and Modeling, 50, 626–637. doi:10.1021/ci900444q
- Mertens, R., Deus-Neumann, B., & Weiler, E. W. (1983). Monoclonal antibodies for the detection and quantitation of the endogenous plant growth regulator, abscisic acid. FEBS Letters, 160, 269–272. doi:10.1016/0014-5793(83)80980-6
- Messih, M. A., Lepore, R., Marcatili, P., & Tramontano, A. (2014). Improving the accuracy of the structure prediction of the third hypervariable loop of the heavy chains of antibodies. Bioinformatics, 30, 2733–2740. doi:10.1093/bioinformatics/btu194
- Morea, V., Lesk, A. M., & Tramontano, A. (2000). Antibody modeling: Implications for engineering and design. Methods, 20, 267–279. doi:10.1006/meth.1999.0921
- Noguchi, H. K., & Tanaka, Y. (2008). Effect of ABA-β-d-glucopyranosyl ester and activity of ABA-β-d-glucosidase in Arabidopsis thaliana. Journal of Plant Physiology, 165, 788–790. doi:10.1016/j.jplph.2007.04.005
- Pearce, B. C., Langley, D. R., Kang, J., Huang, H., & Kulkarni, A. (2009). E-Nove: An automated workflow for efficient structure-based lead optimization. Journal of Chemical Information and Modeling, 49, 1797–1809. doi:10.1021/ci900073k
- Perata, P., Vernieri, P., Armellini, D., Bugnoli, A., Presentini, R., Picciarelli, P., … Tognoni, F. (1990). A monoclonal antibody for the detection of conjugated forms of abscisic acid in plant tissues. Journal of Plant Growth Regulation, 9, 1–6. doi:10.1007/BF02041934
- Sambrook, J., & Russell, D. W. (2005). Molecular cloning: A laboratory manual (3rd ed.). Beijing: Science Press.
- Sartorius, R., Apice1, L. D., Trovato, M., Cuccaro, F., Costa, V., Leo, M. G., … Berardinis, P. (2015). Antigen delivery by filamentous bacteriophage fd displaying an anti-DEC-205 single-chain variable fragment confers adjuvanticity by triggering a TLR9-mediated immune response. EMBO Molecular Medicine, 7, 973–988. doi:10.15252/emmm.201404525
- Singh, P. K., Agrawal, R., Kamboj, D. V., Gupta, G., Boopathi, M., Goel, A. K., & Singh, L. (2010). Construction of a single-chain variable-fragment antibody against the superantigen staphylococcal enterotoxin B. Applied and Environmental Microbiology, 76, 8184–8191. doi:10.1128/AEM.01441-10
- Sircar, A., Kim, E. T., & Gray, J. J. (2009). RosettaAntibody: Antibody variable region homology modeling server. Nucleic Acids Research, 37, W474–W479. doi:10.1093/nar/gkp387
- Suzuki, Y., Ito, S., Otsuka, K., Iwasawa, E., Nakajima, M., & Yamaguchi, I. (2005). Preparation of functional single-chain antibodies against bioactive gibberellins by utilizing randomly mutagenized phage-display libraries. Bioscience, Biotechnology and Biochemistry, 69, 610–619. doi:10.1271/bbb.69.610
- Suzuki, Y., Mizuno, T., Urakami, E., Yamaguchi, I., & Asami, T. (2008). Immunomodulation of bioactive gibberellin confers gibberellin-deficient phenotypes in plants. Plant Biotechnology Journal, 6, 355–367. doi:10.1111/j.1467-7652.2008.00325.x
- Vaks, L., & Benhar, I. (2014). Production of stabilized scFv antibody fragments in the E. coli bacterial cytoplasm. Methods in Molecular Biology, 1060, 171–184. doi:10.1007/978-1-62703-586-6_10
- Wang, Z., Raifu, M., Howard, M., Smith, L., Hansen, D., Golds, R., & Ratner, D. (2000). Universal PCR amplification of mouse immunoglobulin gene variable regions: The design of degenerate primers and an assessment of the effect of DNA polymerase 3’ to 5’ exonuclease activity. Journal of Immunological Methods, 233, 167–177. doi:10.1016/S0022-1759(99)00184-2
- Weiler, E. W. (1980). Radioimmunoassays for the differential and direct analysis of free and conjugated abscisic acid in plant extracts. Planta, 148, 262–272. doi:10.1007/BF00380037
- Wigger, J., Phillips, J., Peisker, M., Hartung, W., Nieden, U., Artsaenko, O., … Conrad, U. (2002). Prevention of stomatal closure by immunomodulation of endogenous abscisic acid and its reversion by abscisic acid treatment: Physiological behaviour and morphological features of tobacco stomata. Planta, 215, 413–423. doi:10.1007/s00425-002-0771-z
- Wu, G., Robertson, D. H., Brooks, C. L., & Vieth, M. (2003). Detailed analysis of grid-based molecular docking: A case study of CDOCKER-A CHARMm-based MD docking algorithm. Journal of Computational Chemistry, 24, 1549–1562. doi:10.1002/jcc.10306
- Xu, Z. W., Escamilla-Treviño, L., Zeng, L. H., Lalgondar, M., Bevan, D., Winkel, B., & Esen, A. (2004). Functional genomic analysis of Arabidopsis thaliana glycoside hydrolase family 1. Plant Molecular Biology, 55, 343–367. doi: 10.1007/s11103-004-0790-1
- Xu, Z. Y., Lee, K. H., Dong, T., Jeong, J. C., Jin, J. B., Kanno, Y., & Hwang, I. (2012). A vacuolar β-glucosidase homolog that possesses glucose-conjugated abscisic acid hydrolyzing activity plays an important role in osmotic stress responses in Arabidopsis. Plant Cell, 24, 2184–2199. doi:10.1105/tpc.112.095935
- Yang, Y., Dib-Hajj, S. D., Zhang, J., Zhang, Y., Tyrrell, L., Estacion, M., & Waxman, S. G. (2012). Structural homology modeling and mutant cycle analysis predict pharmacoresponsiveness of a NaV1.7 mutant channel. Nature Communications, 3, 1186–1197. doi:10.1038/ncomms2184
- Yoshikawa, Y., Oishi, S., Kubo, T., Tanahara, N., Fujii, N., & Furuya, T. (2013). Optimized method of G-protein-coupled receptor homology modeling: Its application to the discovery of novel CXCR7 ligands. Journal of Medicinal Chemistry, 56, 4236–4251. doi:10.1021/jm400307y
- Zhang, Y., Yang, H. Q., Fang, F., Song, L. L., Jiao, Y. Y., Wang, H., … Hung, T. (2015). Single chain variable fragment against Aβ expressed in baculovirus inhibits abeta fibril elongation and promotes its disaggregation. PLoS ONE, 10, 10–26. doi:10.1371/journal.pone.0124736
- Zhao, M., Cascio, D., Sawaya, M. R., & Eisenberg, D. (2011). Structures of segments of asynuclein fused to maltose-binding protein suggest intermediate states during amyloid formation. Protein Science, 20, 996–1004. doi:10.1002/pro.630
- Zhou, G., Liu, Y., Luo, M., Xu, Q. F., Ji, X., & He Z. K. (2012). Peptide-capped gold nanoparticle for colorimetric immunoassay of conjugated abscisic acid. ACS Applied Materials & Interfaces, 4, 5010–5015. doi:10.1021/am301380q