ABSTRACT
As a standard strain of American strains library widely used in scientific research, Escherichia coli ATCC 8739 was used in microarray analysis experiment to study the antimicrobial activity of cinnamaldehyde (CMA). The reverse transcription (RT)-PCR and western blot experiments were used to verify the results of microarray analysis. The microarray analysis showed that 126 genes expressed differentially, where the number of upregulated genes was 83 (65.9%) and downregulated genes was 43 (34.1%). These differentially expressed genes were divided into 16 classes including hypothetical protein (19.8%), metabolism of coenzymes, prosthetic groups (11.9%) and so on. The results of RT-PCR and western blot experiments coincide with the microarray analysis, so the microarray analysis study was effective. These results indicated that flagellin and cell membrane may be the preliminary antibacterial mechanism of CMA. CMA could affect the synthesis and aggregation of cytoplasmic protein and interfere with ATP metabolism. These effects of CMA could lead to bacterial metabolic disorder and autolysis.
1. Introduction
With the living standards improved, people have paid more attention to the food safety problems. Pathogenic bacteria contamination in foods is one of the major causes of foodborne illness and also the key factor that impacts food safety (Kong, Liu, Xing, Kuang, & Xu, Citation2015; Walker, Citation2005; Wang et al., Citation2015). Escherichia coli (E. coli) is one of the main factors which causes the food safety incidents, and it is an important parameter to determine the degree of food contamination (Feng et al., Citation2013; Walker, Steele, & Aguado, Citation2007). Currently, adding additives in foods is an important means to inhibit E. coli and ensure food safety (Svennerholm & Steele, Citation2004).
Internationally, the developmental tendency of food additives is natural, nutritional and functional. The food additives research and application has been gradually extended (Carocho, Morales, & Ferreira, Citation2015; Kim, Her, & Lee, Citation2015). In recent years, developing natural food preservatives has been the main direction to research for the safe, efficient, economical and nutritional food preservatives from natural plant source (Bearth, Cousin, & Siegrist, Citation2014). But, incomplete studies such as various kinds of antibacterial mechanism, antibacterial spectrum and the range of application restrict the utilization and development of natural antiseptic process.
Cinnamaldehyde (CMA) as a food preservative with non-toxic or low toxicity to human body shows good inhibitory effects on gram-negative (Moleyar & Narasimham, Citation1992) and gram-positive bacterias (Goñi et al., Citation2009) such as Staphylococcus aureus, E. coli and Salmonella. Besides, CMA has strong bacteriostatic effects on Aspergillus flavus, Aspergillus niger, yeast and so on. Since the nineteenth century, CMA has been isolated from cinnamon essential oils and was mainly used to impart cinnamon fragrance to cosmetics, medical products and perfumes. In recent years, CMA has been reported to have a broad spectrum of antimicrobial activity against a wide range of microorganism including bacteria, yeasts and molds, and to be more effective than other cinnamon essential oil constituents (Balaguer, Lopez-Carballo, Catala, Gavara, & Hernandez-Munoz, Citation2013). There have been few reports related to investigation of the mechanism of CMA treatment which motivated us to further study the mechanism of CMA treatment against E. coli. Shen et al. (Citation2015) found that the bacterial cell morphology, membrane integrity and permeability were damaged when the E. coli and S. aureus cells were exposed to the minimum inhibitory concentrations of CMA with 0.31 mg/mL.
The rapid development of microarray analysis technology in a few short years has caused wide attention around the world. DNA microarray technology has proven to be useful in rapid detection of viruses and bacteria and it can be used to discover gene’s functions to understand biochemical pathways and to discover drug targets (Thissen et al., Citation2014). Microarray analysis has a great advantage in studying the antibacterial mechanism and side effects evaluation of CMA. And this technology can also provide research foundation for the development and utilization of CMA and other natural preservative.
E. coli, gram-negative bacteria, is a kind of common food spoilage microorganisms. E. coli ATCC 8739, which is sensitive with CMA as reported in previous studies, is a standard strain of American strains library widely used in scientific research (Goh et al., Citation2012; Lee, Oon, Lee, & Ling, Citation2012). In this study, the synthetic microarray analysis was applied to detect the influence of the gene expression profile of E. coli ATCC 8739 exposed to CMA. In addition, the western blot technique and reverse transcription (RT)-PCR technology were used to validate the results of microarray analysis. Then, the target genes of CMA in E. coli ATCC 8739 were screened from the transcriptional level, and the antibacterial mechanism of CMA in E. coli was preliminarily explored.
2. Materials and methods
2.1. Microorganisms and materials
E. coli strain was obtained from Middlebrook 7H9 broth and OADC (oleic acid, albumin, dextrose and catalase) was purchased from BD Biosciences, Inc. (USA). Alamar Blue was obtained from Trek Diagnostic Systems (Westlake, OH, USA). TRIzol was purchased from Invitrogen (USA). Tween 20 and dimethyl sulfoxide (DMSO) were purchased from Sigma-Aldrich (USA). The E. coli (ATCC 8739) microarray was synthesized by Agilent Co. (USA). The analytical grade CMA was obtained from Sigma Chemicals Co. (USA). Anti-groEL and anti-Dnak were purchased from Shanghai Abcam Co. (China). Anti-reduced glyceraldehyde-phosphate dehydrogenase (GAPDH) was purchased from Jiangsu Beyotime Institute of Biotechnology (China). The horseradish peroxidase (HRP)-conjugated secondary antimouse IgG (H + L) from goat was purchased from Beijing ZSGB-BIO Co. (China).
2.2. Antifungal susceptibility testing
The minimum inhibitory concentration (MIC) of CMA against E. coli (ATCC 8739) was determined by broth microdilution using twofold serial dilutions in RPMI 1640 medium as described by NCCLS (M27-A) (Citation1997). Three rounds of experiments were performed, and the average MIC value was calculated.
2.3. RNA isolation
When E. coli strain was cultured to the logarithmic phase, CMA with liquid concentration for 1/2 of MIC was added to the bacterial liquid. The other culture containing 1% (vol/vol) DMSO lacking CMA was used as the control. Then the E. coli strain was cultured for 4 h sequentially. After the bacteria suspension was poured into a centrifuge tube, bacteria were centrifuged and the supernatant was discarded. One milliliter of TRIzol lysis solution was added to the bacteria precipitation (1 × 107), hitted for 10 min repeatedly until bacteria dissolved completely. RNA quantity and quality were measured by using NanoDrop ND-1000. The absorption value of RNA was measured at 260, 280 and 230 nm for the concentration calculation and purity assessment. RNA integrity was assessed by standard denaturing agarose gel electrophoresis.
2.4. DNA microarray
The E. coli ATCC 8739 microarray is well-known and predicted E. coli genes and transcripts. Coupled with NCBI’s gene prediction processes and Agilent’s probe selection, this design delivers increased data quality and less gene coverage redundancy.
Agilent Feature Extraction software (version 11.0.1.1) was used to analyze the acquired array images. Quantile normalization and subsequent data processing were performed with the GeneSpring GX v12.1 software package (Agilent Technologies). After quantile normalization of the raw data, genes that at least 4 out of 4 samples have flags in P or M (“All Targets Value”) were chosen for further data analysis. Differentially expressed genes were identified through Fold change filtering. Hierarchical clustering was performed using the Agilent GeneSpring GX software (version 12.1).
2.5. RNA labeling and array hybridization
Sample labeling and array hybridization were performed according to the Agilent One-Color Microarray-Based Gene Expression Analysis protocol from Agilent Technology (USA). Briefly, total RNA from each sample was linearly amplified and labeled with Cy3-UTP. The labeled cRNAs were purified by RNeasy Mini Kit from Qiagen (Germany). The concentration and specific activity of the labeled cRNAs (pmol Cy3/μg cRNA) were measured by using NanoDrop ND-1000. One microgram of each labeled cRNA was fragmented by adding 11 μL of 10× blocking agent and 2.2 μL of 25× fragmentation buffer, then heated at 60°C for 30 min, and finally 55 μL of 2× GE hybridization buffer was added to dilute the labeled cRNA. One hundred microliters of hybridization solution was dispensed into the gasket slide and assembled to the gene expression microarray slide. The slides were incubated for 17 h at 65°C in an agilent hybridization oven. The hybridized arrays were washed, fixed and scanned with the Agilent DNA Microarray Scanner (part number G2505C).
2.6. Western blot analysis
The bacterial proteins were subjected to 10% SDS-PAGE and electrophoretically transferred onto polyvinylidene difluoride (PVDF) membrane. The membrane was immersed in blocking solution (5% skim milk in TBST) for 2 h and then immunoblotted with primary antibodies (anti-groEL, anti-Dnak at 1:5000 dilutions and anti-GAPDH as internal control) for 2 h at room temperature. The membrane was washed with TBST buffer (2% Tris-HCL, 0.88% NaCl, 0.05% Tween 20) three times followed by a 2-h incubation with HRP-conjugated secondary antimouse IgG (H + L) from goat (1:3000 dilution). The molecular mass of the protein was calculated using a calibration curve of log molecular weight versus relative mobility of standard molecular weight markers. And the gray level of the protein expression was analyzed by using the software, ImageJ.
2.7. RT-PCR assays
The microarray results were verified by RT-PCR. Aliquots of the RNA preparations from the untreated and treated samples used in the microarray experiments were retained for RT-PCR follow-up studies. The RT-PCR was performed in triplicate using the 7000 Sequence Detection System from Applied Biosystems (USA) according to a previously described procedure. The primers are listed in . And the gray level of the RT-PCR results was analyzed by using the software, ImageJ.
Table 1. The primers used in RT-PCR.
3. Results
3.1. Gene transcription responses to CMA exposure
By the antifungal susceptibility testing, the MIC value of CMA against E. coli (ATCC 8739) was 0.31 mg/mL in this work. Whole-genome microarrays were employed to profile gene expression in E. coli in response to exposure to a subinhibitory (1/2 × MIC) concentration of CMA for 4 h, while the control group contained 1% (vol/vol) DMSO lacking CMA. The genes which were differentially expressed in response to CMA and their biological roles according to their functional class are shown in . Overall, there were 126 genes differentially regulated by CMA. Among these, 83 exhibited a significant increase in transcription and 43 exhibited a significant decrease in transcription. These differentially expressed genes were classified as involved in hypothetical protein (19.8%), metabolism of coenzymes and prosthetic groups (11.9%), transport/binding proteins and lipoproteins (10.3%), RNA synthesis (7.9%), protein synthesis (7.9%), metabolism of carbohydrates and related molecules (7.1%), metabolism of amino acids and related molecules (7.1%), adaption to atypical conditions (7.1%), pathogenic factors (6.3%), DNA packaging and segregation (4.0%), tRNA (3.2%), DNA modification and repair (2.4%), cell wall (1.6%), phage-related functions (0.8%), metabolism of nucleotides and nucleic acids (0.8%), and unknown function (1.6%) as shown in . A similar research reported that 40 genes required for cell viability of Saccharomyces cerevisiae were significantly regulated by treatment with p-anisaldehyde; of these, 5 showed an increase in expression and 35 showed a decrease in expression. This result was not the same as in our study, and it may be because of the different bacteria and antimicrobial additives (Yu et al., Citation2010).
Figure 1. CMA-responsive genes grouped by functional classification. The genes were significantly regulated more than 2-fold after taking logarithm. The differentially regulated genes were divided into 16 functional categories. The number of genes upregulated and downregulated for each functional group is represented.
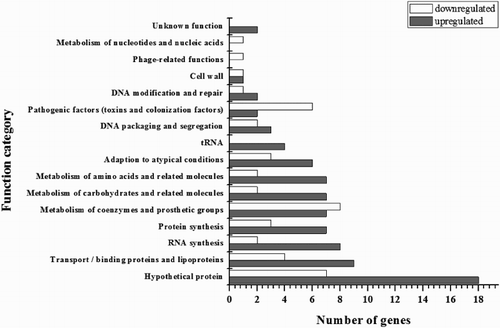
Table 2. Genes with expression changes of at least 2.0 fold in E. coli (ATCC 8739) exposed to CMA.
3.2. Validating microarray data by western blot
The results of western blot experiments are shown in . The gene expression level of dnak and groEL was significantly (P < .05) increased compared with the control group (labeling 1). The protein expression content of treatment groups 1/2 MIC (labeling 2) and 2 MIC (labeling 4) CMA was more than one time that of blank control group and the protein expression content of treatment groups by 1 MIC (labeling 3) CMA was more than two times. In addition, the results that the dnak gene increased 2.92 times and groEL gene increased 2.49 times were verified, and the effectiveness of gene microarray was shown. It was found that with the linear increasing of CMA concentration, the relative content of proteins was not linear increase, even the protein expression content of 2 MIC treatment group was slightly decreased. It is supposed that when the CMA reached a certain bacteriostasis concentration, the protein expression content of E. coli-regulated gene will be no obvious change because of the limit of bacterium liquid. However, the bacteria number decreased sharply when the relative concentration of CMA was high, and this phenomenon may lead to the E. coli-controlled genes’ protein expression slightly decreased with the highest concentration of CMA.
Figure 2. The results of western blot. (A) indicates the group of gene groEL. (B) the analysis of gray level of the GroEL protein expression. (C) indicates the group of gene dnaK. (D) the analysis of gray level of the DnaK protein expression. The labeling 1, 2, 3, 4 indicate that E. coli was inhibited by the 0, 0.16, 0.31, 0.62 mg/mL (0, 1/2, 1, 2 MIC) cinnamic aldehyde, respectively. The different letters (a, b, c) mean that variance of two samples is significant (P < .05).
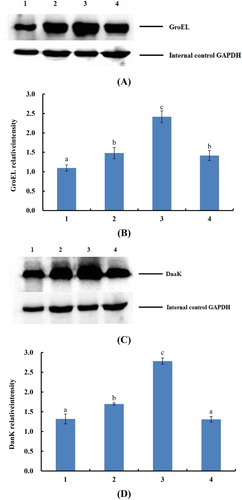
3.3. Validating microarray data by RT-PCR
After microarray analysis, five genes (dkgA, iraD, zraP, flip, argC) were detected. In the process of experiments, every experiment was divided into four groups (): E. coli, control group (labeling 1), 1/2 MIC treatment group (labeling 2), MIC treatment group (labeling 3) and 2 MIC treatment group (labeling 4). In the regulated direction (upregulated or downregulated), the RT-PCR results of these genes were totally the same with the microarray results. Gene flip (−4.73) and argC (−2.45) were downregulated, and with the increase of CMA concentration of the experimental group, the gel stripe gradually became narrow and dark. Moreover, gene dkgA (+6.37), zraP (+2.97) and iraD (+2.51) were upregulated and with the increase of CMA concentration of the experimental groups, the gel stripe gradually became wide and bright. By regulated multiples, only the RT-PCR results of gene argC were relatively consistent, and this may be caused by the reason of large-range RT-PCR analyses. In conclusion, the RT-PCR results agreed with the microarray analysis. However, comparing the RT-PCR and western blot, the microarray can reflect the influenced genes more accurately by up/downregulated numbers not by sensorial pictures.
Figure 3. The results of RT-PCR. M indicates marker. The labeling 1, 2, 3, 4 indicate that E. coli was inhibited by the 0, 0.16, 0.31, 0.62 mg/mL (0, 1/2, 1, 2 MIC) cinnamaldehyde, respectively. (A) The results of RT-PCR of gene flip. (a) Analysis of gray level of gene flip. (B) The results of RT-PCR of gene dkgA. (b) Analysis of gray level of gene dkgA. (C) The results of RT-PCR of gene zraP. (c) Analysis of gray level of gene zraP. (D) The results of RT-PCR of gene iraD. (d) Analysis of gray level of gene iraD. (E) The results of RT-PCR of gene argC. (e) Analysis of gray level of gene argC. The different letters (a, b, c, d) mean that variance of two samples is significant (P < .05).
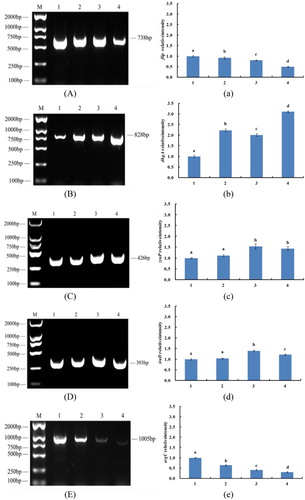
4. Discussion
4.1. Induction of genes involved in chaperonins by CMA
Molecular chaperones are intracellular proteins that promote correctly folding of proteins leading to active three-dimensional structures (Zmijewski, Macario, & Lipinska, Citation2004). They are thought to assist in the folding of their target proteins by providing a sequestered environment which is conducive to correct folding. In this environment, the extended proteins can fold and shield from nonproductive interactions with other proteins (Grigoroudis, McInnes, Premnathc, & Kontopidis, Citation2015). In this study, two dna genes encoding chaperonins, dnaK and groEL, were induced by CMA. The dnaK gene, which encodes the molecular chaperone DnaK, was significantly upregulated by factors of 2.9. DnaK, the major bacterial Hsp70, is one of the most abundant constitutively expressed and stress-inducible chaperones in the E. coli cytosol, and the protein chaperone DnaK was identified as a Cu-induced protein (Grimshaw et al., Citation2005). In E. coli, DnaK is required to chaperone the multicopper oxidase CueR to the TatAB secretion system (Graubner, Schierhorn, & Bruser, Citation2006), which then exports it into the periplasm. Another dna gene (groEL), which encodes the molecular chaperone GroEL, was also significantly upregulated by factors of 2.5. GroEL is required for bacterial growth under all conditions, mediating folding assistance, via its central cavity, to a diverse set of cytosolic proteins; yet, the subcellular localization of GroEL remains unresolved (Charbon et al., Citation2011). Calloni et al. (Citation2012) used E. coli as a model to understand the cooperation of the DnaK system with the upstream chaperone Trigger factor (TF) and the downstream GroEL. In this study, Giulia Calloni found that DnaK functions prominently in stabilizing proteins for subsequent folding by GroEL. Combined loss of DnaK and TF causes proteostasis collapse with disruption of GroEL function, defective ribosomal biogenesis and extensive aggregation of large proteins. The chaperonin GroEL participates in the 2–7% facilitated folding of newly synthesized proteins in E. coli (Ellis & Hartl, Citation1996). A previous study suggested that at high concentrations, either GroEL or DnaK prevents the aggregation of newly synthesized proteins, while at physiological concentrations, the expression of all four hsps is required (Gragerov et al., Citation1992). Ye et al. (Citation2011) suggest that the aggregation of upregulated GroEL and DnaK was prevented in Mycobacterium tuberculosis H37Rv in the presence of plumbagin, while in our study, these two genes were all upregulated and we infer that the aggregation of newly synthesized proteins may be prevented in E. coli by CMA.
4.2. Inhibition of flagellar genes by CMA
Bacterial flagellum is the unique structure for motility powered by an ion-driven rotary motor that allows bacteria to swim in liquid environments. Cell motility (swimming) of enterobacteria like E. coli, Proteus mirabilis and Salmonella typhimurium is mediated by surface flagella (Claret & Hughes, Citation2002). The flagellum is divided into three substructures: the filament, the hook and the basal body (Mizuno, Amida, Kobayashi, Aizawa, & Tate, Citation2011). It assembles from a large number of different proteins. The E. coli flagellar gene regulation and assembly have been extensively characterized. Construction of the flagellar organelle of a bacterium is an exquisitely ordered process involving the temporal expression of genes. These genes are arranged in three classes including class I, class II and class III which are coordinately regulated and expressed in a transcriptional hierarchy (Pallen, Penn, & Chaudhuri, Citation2005). The component export of the flagellum across the cytoplasmic membrane requires a specialized secretion apparatus at its base, which shares homology to the type III secretion apparatus of the bacterial needle used by some gram-negative bacteria in pathogenesis (Chaban, Hughes, & Beeby, Citation2015). For the flagella systems of E. coli, the flagellar export apparatus consists of six integral membrane proteins (FlhA, FlhB, FliO, FliP, FliQ and FliR) and three cytoplasmic proteins (FliH, FliI and FliJ) (Sajó et al., Citation2014). These proteins are called general export components because they are required for rod-type (FlgB, FlgC, FlgF and FlgG), hook-type (FlgD and FlgE) and filament-type (FlgM, FlgK, FlgL, FliC and FliD) export substrates (Terashima, Kojima, & Homma, Citation2008).
In our study, the genes flgD, flhB, fliP and fliR were significantly downregulated more than 2-fold after taking logarithm when exposed to CMA. The flgD gene, which encodes the flagellar basal body rod modification protein FlgD, was significantly downregulated by factors of 5.3. FlgD is an essential scaffolding protein which caps the hook during its assembly. Though it has been reported that the transport of FlgD is accomplished by type III secretion system in E. coli, its immune protection has not been fully elucidated (Weber-Sparenberg et al., Citation2006). In addition to flgD, three genes fliP, flhB and fliR were also downregulated by approximately 4.7-fold, 3.8-fold and 3.7-fold, respectively. All of these genes encode flagellar biosynthesis proteins which are related to the protein export. The fliP gene encodes flagellar biosynthesis protein FliP, flhB gene encodes FlhB, while fliR gene encodes FliR. It is reported that the bacterial flagellar export apparatus consists of about five membrane proteins (FlhA, FlhB, FliP, FliQ and FliR) and three soluble proteins (Minamino, Citation2014). The membrane proteins are highly conserved with a high sequence identity from bacterial species to species, and to the export apparatus of the needle complex (Barker & Samatey, Citation2012). All in all, observation from these results suggests that the downregulation of the genes may affect the flagellar biosynthesis in the presence of CMA, and then the cell motility (swimming) of E. coli is limited.
4.3. Effects of transport/binding proteins and lipoproteins by CMA
The results of this experiment showed that 13 gene-related transport/binding proteins and lipoproteins were impacted by CMA including 9 upregulated genes (EcolC_3132, EcolC_4087, EcolC_4244, EcolC_2029, EcolC_0110, EcolC_1541, EcolC_4101, EcolC_1903 and EcolC_1254) and 4 downregulated genes (EcolC_0537, EcolC_2144, EcolC_2964 and EcolC_0295). The function of EcolC_3132 was encoding copper exporting ATPase, EcolC_4087 was encoding ATP-dependent protease ATP-binding subunit HslU, EcolC_4244 was encoding ribose ABC transporter permease, EcolC_2029 was encoding putative transport protein, EcolC_0110 was encoding putative cytoplasmic protein, EcolC_1541 was encoding nickel/cobalt efflux protein RcnA, EcolC_4101 was encoding sulfate transporter subunit, EcolC_1903 was encoding sodium:dicarboxylate symporter, EcolC_1254 was encoding thiosulfate transporter subunit, EcolC_0537 was encoding tryptophan permease, EcolC_2144 was encoding monosaccharide-transporting ATPase, EcolC_2964 was encoding putrescine transporter, and EcolC_0295 was encoding transcriptional regulator MalT. In addition, two genes related to the ATPase are cause for concern. One of the two related genes is hslU (2.18, System name EcolC4087) participate in encoding HslVU protein an ATP-dependent protease consisting of two multimeric components: the HslU ATPase and the HslV peptidase (Shin et al., Citation1996). HslU promotes the ability of SulA to block cell growth through its chaperone function in cell growth inhibition by preventing SulA aggregation and as the regulatory component for elimination of SulA by supporting the HslV-mediated degradation (Seong, Oh, Leea, Tanaka, & Chung, Citation2000). Thus, the HslU protein expression was enhanced by the upregulated hslU (2.18). Moreover, the growth of E. coli was inhibited indirectly by HslU through its chaperone function. The other gene (−2.84, System name EcolC2144) encodes a transmembrane ATPase: monosaccharide-transporting ATPase which can keep the balance of monosaccharide metabolism inside and outside of cells (Sun-Wada & Wada, Citation2015). Transmembrane ATPase can input many metabolic substances for cells and output poison, metabolic wastes and other materials that may hinder cellular processes (Chingaté, Delgado, Salaza, & Soto, Citation2015). In addition, ATP-dependent proteolysis plays an essential role in protein quality control and gene regulation by removing misfolded proteins and short-lived regulatory protein factors through regulating certain cellular protein levels (Wang et al., Citation2001). Under the effects of CMA, the synthesis of monosaccharide transfer ATPase of E. coli was inhibited. Thus, it can be speculated that the bacterial metabolism was affected by CMA through the inhibition of ATPase. Some studies show that the binding activity of bacterial cell membranes and ATPase may be inhibited by CMA, so it has a significant impact on bacteriostatic effect of sublethal concentrations of CMA (Denyer, Citation1990; Shen et al., Citation2015). This study clearly supports the conclusion that CMA has inhibitory effects on the ATPase.
5. Conclusions
The microarray analysis showed that 126 genes expressed differentially, 2-fold difference in expression as a significant criterion, where the number of upregulated genes was 83 (65.9%) and the number of downregulated genes was 43 (34.1%). Among these genes, chaperonins, flagellar and ATP metabolism-related genes were regulated expression. Because of the inhibition of some chaperone protein genes, the normal protein synthesis and aggregation of E. coli may be prevented by CMA. And the downregulation of the flagellar genes may affect the flagellar biosynthesis in the presence of CMA through limiting the cell motility of E. coli. At last, the bacterial ATP metabolism-related genes were affected by CMA, so it may also be an antibacterial mechanism through ATPase inhibition of bacterial metabolism.
Disclosure statement
No potential conflict of interest was reported by the authors.
Notes on contributors
Songyi Lin is a full professor of Food science and technology of Dalian Polytechnic University engineering. She got her Ph.D of engineering in 2005. Her research interests are food science and agriculture products processing.
Rong Liang is a student reading for doctor degree in College of Food science and technology of Jilin University. Her research interests are food quality control technology and functional foods.
Tiehua Zhang is a full professor of College of food science and technology of Jilin University. He got his Ph.D of engineering in 2008. His research interest is food science.
Yuan Yuan is a full professor of College of food science and technology of Jilin University. She got her Ph.D of engineering in 2007. Her research interests are food safety and food control.
Suxia Shen is a master of engineering. She studied in College of food science and technology of Jilin University and got her master degree in 2013. Her research interest is food safty.
Haiqing Ye is a professor of College of food science and technology of Jilin University. He got his Ph.D in 2013. His research interest are food safety and food control.
Additional information
Funding
References
- Balaguer, M. P., Lopez-Carballo, G., Catala, R., Gavara, R., & Hernandez-Munoz, P. (2013). Antifungal properties of gliadin films incorporating cinnamaldehyde and application in active food packaging of bread and cheese spread foodstuffs. International Journal of Food Microbiology, 166(3), 369–377. doi:10.1016/j.ijfoodmicro.2013.08.012
- Barker, C. S., & Samatey, F. A. (2012). Cross-complementation study of the flagellar type III export apparatus membrane protein FlhB. PLos ONE, 7(8), e44030. doi:10.1371/journal.pone.0044030
- Bearth, A., Cousin, M. E., & Siegrist, M. (2014). The consumer’s perception of artificial food additives: Influences on acceptance, risk and benefit perceptions. Food Quality and Preference, 38, 14–23. doi:10.1016/j.foodqual.2014.05.008
- Calloni, G., Chen, T., Schermann, S. M., Chang, H., Genevaux, P., Agostini, F., … Hartl, F. U. (2012). DnaK functions as a central hub in the E. coli chaperone network. Cell Reports, 1(3), 251–264. doi:10.1016/j.celrep.2011.12.007
- Carocho, M., Morales, P., & Ferreira, I. C. F. R. (2015). Natural food additives: Quo vadis? Trends in Food Science & Technology, 45(2), 284–295. doi:10.1016/j.tifs.2015.06.007
- Chaban, B., Hughes, H. V., & Beeby, M. (2015). The flagellum in bacterial pathogens: For motility and a whole lot more. Seminars in Cell & Developmental Biology, 46, 91–103. doi:10.1016/j.semcdb.2015.10.032
- Charbon, G., Wang, J., Brustad, E., Schultz, P. G., Horwich, A. L., Jacobs-Wagner, C., & Chapman, E. (2011). Localization of GroEL determined by in vivo incorporation of a fluorescent amino acid. Bioorganic & Medicinal Chemistry Letters, 21(20), 6067–6070. doi:10.1016/j.bmcl.2011.08.057
- Chingaté, S., Delgado, G., Salaza, L. M., & Soto, C. Y. (2015). The ATPase activity of the mycobacterial plasma membrane is inhibited by the LL37-analogous peptide LLAP. Peptides, 71, 222–228. doi:10.1016/j.peptides.2015.07.021
- Claret, L., & Hughes, C. (2002). Interaction of the atypical prokaryotic transcription activator FlhD2C2 with early promoters of the flagellar gene hierarchy. Journal of Molecular Biology, 321(2), 185–199. doi:10.1016/S0022-2836(02)00600-9
- Denyer, S. P. (1990). Mechanisms of action of antibacterial biocides. International Biodeterioration & Biodegradation, 36(3), 227–245. doi:10.1016/0964-8305(96)00015-7
- Ellis, R. J., & Hartl, F. U. (1996). Protein folding in the cell: Competing models of chaperonin function. Faseb Journal, 10, 20–26.
- Feng, M., Yong, Q., Wang, W., Kuang, H., Wang, L., & Xu, C. (2013). Development of a monoclonal antibody-based ELISA to detect Escherichia coli O157:H7. Food and Agricultural Immunology, 24(4), 481–487. doi:10.1080/09540105.2012.716026
- Goh, D. J. W., How, J. A., Lim, J. Z. R., Ng, W. C., Oon, J. S. H., Lee, K. C., … Ling, M. H. T. (2012). Gradual and step-wise halophilization enables Escherichia coli ATCC 8739 to adapt to 11% NaCl. Electronic Physician, 4(3), 527–535.
- Goñi, P., López, P., Sánchez, C., Gómez-Lusa, R., Becerrila, R., & Nerín, C. (2009). Antimicrobial activity in the vapour phase of a combination of cinnamon and clove essential oils. Food Chemistry, 116(4), 982–989. doi:10.1016/j.foodchem.2009.03.058
- Gragerov, A., Nudler, E., Komissarova, N., Gaitanaris, G. A., Gottesman, M. E., & Nikiforov, V. (1992). Cooperation of GroEL/GroES and DnaK/DnaJ heat shock proteins in preventing protein misfolding in Escherichia coli. Proceedings of the National Academy of Sciences of the United States of America, 89, 10341–10344. doi: 10.1073/pnas.89.21.10341
- Graubner, W., Schierhorn, A., & Bruser, T. (2006). DnaK plays a pivotal role in Tat targeting of CueO and functions beside SlyD as a general Tat signal binding chaperone. Journal of Biological Chemistry, 282(10), 7116–7124. doi:10.1074/jbc.M608235200
- Grigoroudis, A. I., McInnes, C., Premnathc, P. N., & Kontopidis, G. (2015). Efficient soluble expression of active recombinant human cyclin A2 mediated by E. coli molecular chaperones. Protein Expression and Purification, 113, 8–16. doi:10.1016/j.pep.2015.01.013
- Grimshaw, J. P. A., Siegenthaler, R. K., Züger S., Schonfeld, H.- J., Z’graggen, B. R., & Christen, P. (2005). The heat-sensitive Escherichia coli grpE280 phenotype: Impaired interaction of GrpE(G122D) with DnaK. Journal of Molecular Biology, 353(4), 888–896. doi:10.1016/j.jmb.2005.08.069
- Kim, J. S., Her, J. Y., & Lee, K. G. (2015). Formation and reduction of carcinogenic furan in various model systems containing food additives. Food Chemistry, 189, 108–113. doi:10.1016/j.foodchem.2014.10.128
- Kong, D., Liu, L., Xing, C., Kuang, H., & Xu, C. (2015). Sensitive and highly specific detection of Cronobacter sakazakii based on monoclonal sandwich ELISA. Food and Agricultural Immunology, 26(4), 566–576. doi:10.1080/09540105.2014.998634
- Lee, C. H., Oon, J. S. H., Lee, K. C., & Ling, M. H. T. (2012). Escherichia coli ATCC 8739 adapts to the presence of sodium chloride, monosodium glutamate, and benzoic acid after extended culture. ISRN Microbiology. doi:10.5402/2012/965356
- Minamino, T. (2014). Protein export through the bacterial flagellar type III export pathway. Biochimica et Biophysica Acta, 1843, 1642–1648. doi:10.1016/j.bbamcr.2013.09.005
- Mizuno, S., Amida, H., Kobayashi, N., Aizawa, S., & Tate, S. (2011). The NMR structure of FliK, the trigger for the switch of substrate specificity in the flagellar Type III secretion apparatus. Journal of Molecular Biology, 409(4), 558–573. doi:10.1016/j.jmb.2011.04.008
- Moleyar, V., & Narasimham, P. (1992). Antibacterial activity of essential oil components. International Journal of Food Microbiology, 16(4), 337–342. doi: 10.1016/0168-1605(92)90035-2
- National Committee for Clinical Laboratory Standards (NCCLS). (1997). Reference method for broth dilution antifungal susceptibility testing of yeasts. Proposed standard M27-A. National Committee for Clinical Laboratory Standards, Villanova.
- Pallen, M. J., Penn, C. W., & Chaudhuri, R. R. (2005). Bacterial flagellar diversity in the post-genomic era. Trends in Microbiology, 13(4), 143–149. doi:10.1016/j.tim.2005.02.008
- Sajó, R., Liliom, K., Muskotál, A., Klein, Á., Závodszky, P., Vonderviszt, F., & Dobó, J. (2014). Soluble components of the flagellar export apparatus, FliI, FliJ, and FliH, do not deliver flagellin, the major filament protein, from the cytosol to the export gate. Biochimca et Biophysica Acta, 1843, 2414–2423. doi:10.1016/j.bbamcr.2014.07.004
- Seong, I. S., Oh, J. Y., Leea, J. W., Tanaka, K., & Chung, C. H. (2000). The HslU ATPase acts as a molecular chaperone in prevention of aggregation of SulA, an inhibitor of cell division in Escherichia coli. FEBS Letters, 477, 224–229. doi:10.1016/S0014-5793(00)01808-1
- Shen, S., Zhang, T., Yuan, Y., Lin, S., Xu, J., & Ye, H. (2015). Effects of cinnamaldehyde on Escherichia coli and Staphylococcus aureus membrane. Food Control, 47, 196–202. doi:10.1016/j.foodcont.2014.07.003
- Shin, D. H., Yoo, S. J., Shim, Y. K., Seol, J. H., Kang, M., & Chung, C. H. (1996). Mutational analysis of the ATP-binding site in HslU, the ATPase component of HslVU protease in Escherichia coli. FEBS Letters, 398, 151–154. doi:10.1016/S0014-5793(96)01223-9
- Sun-Wada, G. H., & Wada, Y. (2015). Role of vacuolar-type proton ATPase in signal transduction. Biochimca et Biophysica Acta, 1847(10), 1166–1172. doi:10.1016/j.bbabio.2015.06.010
- Svennerholm, A. M., & Steele, D. (2004). Progress in enteric vaccine development. Best Practice Research Clinical Gastroenterology, 18(2), 421–445. doi:10.1053/ybega.2004.451
- Terashima, H., Kojima, S., & Homma, M. (2008). Chapter 2 flagellar motility in bacteria: Structure and function of flagellar motor. International Review of Cell and Molecular Biology, 270, 39–85. doi:10.1016/S1937-6448(08)01402-0
- Thissen, J. B., McLoughlin, K., Gardner, S., Gu, P., Mabery, S., Slezak, T., & Jaing, C. (2014). Analysis of sensitivity and rapid hybridization of a multiplexed microbial detection microarray. Journal of Virological Methods, 201, 73–78. doi:10.1016/j.jviromet.2014.01.024
- Walker, R. I. (2005). Considerations for development of whole cell bacterial vaccines to prevent diarrheal diseases in children in developing countries. Vaccine, 23(26), 3369–3385. doi:10.1016/j.vaccine.2004.12.029
- Walker, R. I., Steele, D., & Aguado, T. (2007). Analysis of strategies to successfully vaccinate infants in developing countries against enterotoxigenic E. coli (ETEC) disease. Vaccine, 25(14), 2545–2566. doi:10.1016/j.vaccine.2006.12.028
- Wang, W., Feng, M., Kong, D., Liu, L., Song, S., & Xu, C. (2015). Development of an immunochromatographic strip for the rapid detection of Pseudomonas syringae pv. maculicola in broccoli and radish seeds. Food and Agricultural Immunology, 26(5), 738–745. doi:10.1080/09540105.2015.1023266
- Wang, J., Song, J. J., Seong, I. S., Franklin, M. C., Kamtekar, S., Eom, S. H., & Chung, C. H. (2001). Nucleotide-dependent conformational changes in a protease-associated ATPase HslU. Structure, 9(11), 1107–1116. doi:10.1016/S0969-2126(01)00670-0
- Weber-Sparenberg, C., Pöplau, P., Brookman, H., Rochón, M., Möckel, C., Nietschke, M., & Jung, H. (2006). Characterization of the type III export signal of the flagellar hook scaffolding protein FlgD of Escherichia coli. Archives of Microbiology, 186(4), 307–316. doi:10.1007/s00203-006-0146-0
- Ye, H., Liu, Z., Guo, A., Liang, J., Guo, N., Zeng, F., … Yu, L. (2011). Global transcriptional profiles of Mycobacterium tuberculosis treated with plumbagin. World Journal of Microbiology and Biotechnology, 27, 2261–2269. doi:10.1007/s11274-011-0689-3
- Yu, L., Guo, N., Yang, Y., Wu, X., Meng, R., Fan, J., … Deng, X. (2010). Microarray analysis of p-anisaldehyde-induced transcriptome of Saccharomyces cerevisiae. Journal of Industrial Microbiology & Biotechnology, 37, 313–322. doi:10.1007/s10295-009-0676-y
- Zmijewski, M. A., Macario, A. J. L., & Lipinska, B. (2004). Functional similarities and differences of an archaeal Hsp70(DnaK) stress protein compared with its homologue from the bacterium Escherichia coli. Journal of Molecular Biology, 336(2), 539–549. doi:10.1016/j.jmb.2003.12.053