Abstract
Purpose: There remains an unmet medical need for radioprotective and mitigative agents. BP-C2 is a novel lignin-derived polyphenolic composition with ammonium molybdate, developed as radioprotector/radiomitigator.
Objectives: The present study evaluated BP-C2 for the mitigation of acute radiation syndrome (ARS).
Methods: A total-body irradiation mouse model (TBI, 4.0–8.0 Gy) was used in the study.
Results: In a 30-day survival study, performed in CBA mice, BP-C2, at a dosage of 81.0 mg/kg, improved survival (dose reduction factor (DRF) = 1.1) and increased the formation of endogenous spleen colony-forming units (CFU). In C57BL/6 mice, BP-C2, when administered daily for 7 days, starting 24 hours after TBI, also improved survival. In animals irradiated with 5.0 Gy, BP-C2 increased the number of CFUs (6.7 ± 5.1) compared to the 5.0 Gy placebo group (2.3 ± 2.3, p = .0245). The number of surviving intestinal crypts was maintained in the 5.0 Gy BP-C2 group (133.7 ± 13.9), in contrast to the 5.0 Gy placebo group (124.2 ± 10.5, p < .0023). BP-C2 also increased the number of LGR5 + positive cells in intestinal crypts.
Conclusion: BP-C2 mitigates radiation-induced damage in mid-lethal range of radiation doses. Effects are mediated by enhancement of extramedullar hematopoiesis in the spleen and a protective effect on the intestinal epithelium.
Introduction
In recent decades, radiation accidents, the acceleration of space exploration programs, the increasing use of radiotherapy and radio-imaging techniques, and the growing threat of nuclear terrorism have given rise to global demand for radioprotective agents. Several radioprotectors, mitigators and treatment agents are under development and have made it through the nonclinical and early clinical phases; however, only a few hematopoietic colony-stimulating factors have obtained marketing authorization from the US FDA (Singh et al. Citation2015). Thus, there remains an unmet medical need for radioprotective and mitigative agents. Phenolic compounds (rosmarinic acid (Xu et al. Citation2016), lycopene (Gajowik and Dobrzynska Citation2014) and flavonoids (Grebeniuk et al. Citation2013; Vasin Citation2014)) are reported to exert radioprotective effects through various mechanisms. BP-C2 is a novel lignin-derived polyphenolic composition with ammonium molybdate. The polyphenolic part comprises low- and high-molecular fractions of highly functionalized conjugated benzenepolycarboxylic acids (Shipov et al. Citation2013) (e.g. 2-hydroxy-4-(3,5,7-trihydroxy-4-oxo-4H-chromen-2-yl)benzoic acid, 3-(benzyloxy)-4,5-dihydroxybenzoic acid, biphenyl-2,2',3,3'-tetracarboxylic acid, etc.). Molybdenum, an essential micronutrient, acts as a cofactor in multiple detoxification system enzymes (Ale-Ebrahim et al. Citation2015). Complex organic compounds, containing variable-valence metals, appear to be promising radioprotective and radiomitigative agents (Sorenson Citation1984; Miura et al. Citation1998). One possible mechanism of action of these plant-derived complexes of polyphenolic origin may involve the radical-scavenging properties of these compounds (Kuntić et al. Citation2013) and their ability to inhibit lipid oxidation, induced by γ-radiation (Zbikowska et al. Citation2016).
It is well known that radiation induces DNA damage, alters gene expression and causes mitotic arrest and apoptosis in dividing cells. Murine bone marrow cells were found to be the most sensitive of all mammalian cells (Dainiak Citation2002). The radiation affects both hematopoietic stem cells themselves and the stromal elements’ ability to serve hematopoiesis and leads to a dose-dependent decline in bone marrow production (Greenberger Citation1991).
The intestinal mucosa, due to its fast renewal and high mitotic rate, loses a portion of the cycling cells and undergoes crypt shrinkage in response to irradiation. Crypt regeneration depends on stem cell viability. Rapidly cycling Lgr5-positive stem cells, located at the crypt base among Paneth cells, are thought to make the most prominent contribution to crypt growth. These cells give rise to all the cell lineages in crypts and villi, cycle rapidly and are relatively radioresistant compared to transiently amplifying cells (Barker et al. Citation2012; Hua et al. Citation2012). Though early progenitors or quiescent stem cells can differentiate into crypt base cells (Lgr5+), maintenance of Lgr5 + cells was shown to be essential for crypt regeneration (Metcalfe et al. Citation2014). The efficacy of radioprotectors/radiomitigators is usually evaluated with hematological white blood cells assessment and crypt survival assays. As a rule, such agents are effective against hematopoietic or gastrointestinal radiation injury (Singh et al. Citation2014a). Gamma-tocotrienol (GT3) is an exception to this rule as it, when administered prior to radiation, is known to protect mice against both hematopoietic and gastrointestinal injury (Ghosh et al. Citation2009).
Radioprotective efficacy of oral BP-C2 was earlier demonstrated in the 30-day survival study in rats exposed to total body irradiation (Panchenko et al. Citation2017) and combined protective and therapeutic efficacy of topical BP-C2 was investigated by Fares et al. (Citation2015) in mouse models of radiation-induced dermatitis. The therapeutic window of BP-C2 is wide, as the LD50 of oral BP-C2 in mice is 8000 mg/kg (Meabco AS data on file).
The aim of this study was to assess the potential radioprotective and radiomitigative effects of BP-C2 in mouse TBI models.
Materials and methods
Materials
Two percent aqueous solution of BP-C2 was kindly provided by Meabco A/S (Copenhagen, Denmark). Purified water was used as a solvent and as the placebo.
Ethics statement
Experimental animals were handled in accordance with the Guide for the Care and Use of Laboratory Animals, 8th edition. Protocols were approved by the Local Ethics Committee of the N. N. Petrov Research Institute of Oncology (Protocol No. 1 dated 13 February 2014). Experimental animals found in moribund condition were euthanized by trained personnel (animals were considered moribund if they scored 8–9 points on decreased activity, squinted eyes and hunched posture, each weighed on the 1–3 scale, and a body weight loss of more than 30%), and the remaining animals were euthanized at the end of the studies by the CO2 inhalation method.
Animals
Male 10–12-week CBA and C57BL/6 mice weighing 18–22 g were purchased from the Rappolovo animal nursery (Rappolovo, Leningrad area, Russia). The animals were acclimated for at least 10 days prior to the start of the experiments. The animals were maintained on a 12:12 light/dark cycle at 21 ± 2 °C with 50 ± 20% average humidity and with free access to a standard rodent diet (Laboratorkorm Ltd., Moscow, Russia) and tap water. No concomitant supportive care was given to the experimental animals after irradiation. Irradiated animals were examined twice daily for signs of morbidity or mortality.
Radiation exposure
TBI (total body irradiation) was performed by external γ-irradiation from a cesium-137 source at a dose rate of 1.08 Gy/min. The mice were placed into plexiglas boxes, which each holds 24 animals. Each exposure was confirmed by placing an Inlight dot dosimeter inside a dummy paraffin mouse that was then irradiated together with the experimental animals. The dosimeters were read using a validated reader calibrated using standard dot dosimeters. The reproducibility of individual dots was 5 ± 1% with an accuracy of 4 ± 2%, well within the industry standard for experimental radiation dosimetry (±10%).
The animals were not sedated or anesthetized. Animals in the experimental and matching control groups were irradiated in the same box at the same time; each animal was placed in an individual compartment. Irradiation was performed in the morning between 9:00 and 11:00 (MSK time zone).
30-Day survival study and analysis of endogenous colony-forming units in CBA mice
Two hundred forty male CBA mice were randomly divided into four experimental groups. Three doses were used in the study: 1/650, 1/50 and 1/25 of LD10 established in male mice. 1/25 dose level corresponds to the limited volume of oral dose of BP-C2. Three experimental groups were given respectively 6.0 mg/kg, 81.0 mg/kg, and 156.0 mg/kg of BP-C2 by gavage in the amount of 0.2 ml per animal every second day, from day 10 before irradiation until day 10 after irradiation (10 times total). One group was given the same volume of placebo by the same route and on the same schedule as the BP-C2. Each experimental group was further divided into five subgroups (n = 12) exposed to 4.0, 5.0, 6.0, 7.0 and 8.0 Gy, respectively. Irradiation was performed after the 5th administration of BP-C2 or the placebo. An additional 96 male CBA mice were used in a second set of experiments run only for 6.0 and 7.0 Gy.
Mortality was recorded for 30 days after irradiation. Survival rates were calculated on day 30 after irradiation. Probit analysis was performed to calculate 16%, 50% and 84% lethal doses of radiation (LD). The DRF was calculated as the ratio of the LD50/30 of the BP-C2 group to that of the placebo group.
In an additional set of experiments, CBA male mice were divided into two groups (12 animals each) – a placebo group and a BP-C2 group (81 mg/kg) – and given the placebo or BP-C2 every second day (10 times total) until day 10 after irradiation (LD50/30 = 5.7 Gy on day 0). On day 10, the animals were euthanized and the endogenous spleen colony-forming units (CFUs) were analyzed.
Short-term study in C57BL/6 mice
Male C57BL/6 mice were randomized into nine groups (n = 10 each). One group (the control group) was ‘false-irradiated’ (the animals were placed inside boxes for radiation but were not irradiated) and did not receive any further interventions. Eight other groups received 5.0, 6.0, 7.0 and 8.0 Gy of total-body radiation, two groups per dose. Twenty-four hours postirradiation, BP-C2 was administered to animals from one of the two groups irradiated with equal doses, and the placebo was administered to animals from the other irradiated groups (placebo groups) by the same route, in the same dose, and on the same schedule as BP-C2. BP-C2 was administered via gavage (81.0 mg/kg, 0.2 ml per animal; the dose found effective on CBA mice was used for the study on C57BL/6 mice) daily for 7 days after irradiation. Because of the earlier reported low toxicity of BP-C2, no additional group, where false-irradiated animals would be treated with BP-C2, was included. Animals were examined twice daily for 7 days, and mortality dynamics and general condition were recorded. At day 8 post-irradiation, the surviving animals in all groups were euthanized, and spleen, bone marrow, jejunum and blood samples were collected.
The study was designed in accordance with FDA guidelines on testing efficacy of medical countermeasures against ARS in murine model developed by Plett et al. (Citation2012). In the study, the animals were euthanized on days 3, 8 and 17 after irradiation with 8 Gy TBI and underwent histopathological examination. By day 8, foci of myeloid cells were detectable in the bone marrow, small germinal centers with indistinct mantle zones were found in the spleen, and small intestines appeared without gross abnormalities, although villi blunting and modest crypt loss with signs of regeneration (Plett et al. Citation2012). Gastrointestinal damages, marked by villi shortening and apoptotic death in the small intestine, were registered by day 3 and lasted at least up to day 14 (Jeong et al. Citation2016). Thus to reduce the number of experimental animals in the spirit of 3Rs principle, day 8 was specifically chosen as the time point for collecting samples for the assessment of both hematological and intestinal changes.
Hematological analysis of peripheral blood
Peripheral blood samples were collected into K3-EDTA tubes (MiniCollect). Clinical blood analysis was performed using Abacus Junior/Arcus Hematology Analyzer. The analysis of peripheral blood was performed within 1–2 hours after blood sampling.
Bone marrow nucleated cell (BMNC) assay
Quantitative analysis of the bone marrow cellularity was performed in a bone marrow cell suspension prepared by mixing 0.02 ml of femoral punctate with 0.4 ml of a 3% acetic acid solution. BMNCs were counted with a hemocytometer (McCulloch and Till Citation1960). The number of nucleated bone marrow cells was used to calculate the cell survival dose–response relationship. This relationship can be described with a single-impact multitarget model and expressed by the equation:
Mathematical modelling was performed to calculate the D0 (average cellular lethal dose) and n (extrapolation coefficients, their errors and significance levels).
Endogenous spleen colony-forming unit (CFU) assay
CFUs were analyzed in the spleens of euthanized mice. The spleens were dissected and weighed, then fixed in Bouin’s solution (composed of picric acid, acetic acid and formaldehyde) for 24 h. Macroscopic colonies (CFUs) visible to the naked eye were scored in each spleen (Till et al. Citation1964).
Assay for surviving intestinal crypts (SIC)
The jejunum after dissection was immediately fixed in 10% buffered neutral formaldehyde (pH 7.4), and 10 transverse cross-cuts were then prepared and subjected to routine histological processing. Tissue sections (4 μm) were cut from paraffin blocks and stained with hematoxylin and eosin (H&E). The number of surviving crypts per jejunum cross-cut was counted using light microscopy. At least six jejunum cross-cuts were evaluated for each animal, and the average number of surviving crypts was calculated for each experimental group. A crypt was defined as viable if it contained a cluster of 10 or more cells with nuclear basophilic staining (Withers and Elkind Citation1970).
Crypt stem cell and proliferating cell assays
Additional paraffin-embedded tissue sections were made for immunohistochemical evaluation of Lgr5 stem cell marker and proliferating cell nuclear antigen (PCNA). Slides were deparaffinized and placed into epitope retrieval solution (Dako, pH 6.0) at 95 °C for 30 minutes. Endogenous peroxidase activity was blocked using 3% H2O2 solution for 15 minutes. The slides were further washed in PBS and immunostained with primary anti-Lgr5 (bs-1117r, Bioss Antibodies, Inc.) or anti-PCNA (bs-0754r, Bioss Antibodies, Inc.) rabbit polyclonal antibodies and further with an anti-rabbit HRP-conjugated detection system (DAKO EnVision + System, HRP). Binding was visualized using 3,3′-diaminobenzidine, which produces a brown precipitate at the antigen site. For intestinal stem cell assessment, Lgr5 + cells were counted in longitudinally cut crypts containing Paneth cells (300–350 crypts per group). The percentage of PCNA-expressing cells was counted up to position 20 and was estimated at an average of 100 crypts per group.
Statistical analysis
The experimental data were statistically analyzed (GraphPad Prizm 6.01, San Diego, CA). The log-rank test was used to perform the survival analysis and compare the survival curves (Kaplan–Meier curves). Fisher's exact test was used to compare survival rates at study termination (day 30 or day 8 postirradiation).
Secondary endpoints (e.g. BMNC count, spleen weight, number of CFUs and number of SIC) were analyzed using one-way ANOVA with the Tukey post-test and the Mann–Whitney U test, where appropriate, and data were summarized descriptively by mean (M) and standard deviation (SD), unless otherwise stated. Results were considered statistically significant at p < .05.
Results
Effect of BP-C2 on survival of irradiated CBA mice
The results presented in indicate that BP-C2 increases survival of CBA mice. BP-C2 demonstrated the most pronounced radioprotective effect when given at 81.0 mg/kg and was most effective in the median lethal radiation dose range. In this dose range, BP-C2 increased survival of animals exposed to 5.0 Gy and 6.0 Gy by 16.7% and 15.0%, respectively.
Table 1. Effect of BP-C2 on the outcome of acute radiation injuries in CBA mice.
Survival curves are presented in . At 5.0 Gy TBI (), no significant changes were observed in any of the BP-C2 treated groups as compared to the placebo group, but survival was higher at all tested doses of BP-C2. At 6.0 Gy (), only 81.0 mg/kg of BP-C2 caused a statistically significant increase in survival. No dose dependency was noted with regards to the BP-C2 effect. At 7.0 Gy (), differences in survival between the placebo group (0% survival) and the experimental groups treated with 6.0, 81.0 and 156.0 mg/kg of BP-C2 were not statistically significant and constituted 4.2%, 4.2% and 8.3%, respectively.
Figure 1. Effect of BP-C2 on survival of CBA mice after TBI. BP-C2 or Placebo were given by gavage 5 times every second day prior to, and 5 times every second day after, irradiation. n = 12 animals per group at 5.0 Gy and n = 24 animals per pooled group at 6.0 and 7.0 Gy from two sets of experiments.
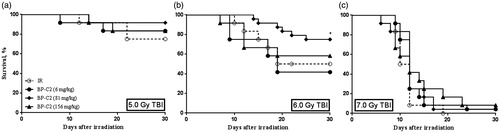
These results were used to calculate the lethal doses of radiation presented in . The highest LD50/30 was achieved with 81.0 mg/kg BP-C2. Lower (6.0 mg/kg) or higher (156.2 mg/kg) BP-C2 doses had a smaller effect on half-lethal doses of radiation. At 81.0 mg/kg, BP-C2 ensured a DRF of 6.2/5.7 = 1.1 in CBA mice.
Table 2. Effect of BP-C2 on LD50 doses in CBA mice.
The effect of a BP-C2 dose of 81.0 mg/kg on endogenous colony formation in the spleen was assessed (). Spleen weight and CFU count were significantly higher in animals treated with this dose of BP-C2. Based on this, 81.0 mg/kg was chosen as the optimal dose for the subsequent short-term survival experiment, in which the therapeutic efficacy of the compound was assessed in the C57BL/6 mice.
Figure 2. Effect of BP-C2 on weight of spleen and number of CFUs in CBA mice on day 10 after TBI. BP-C2 (81 mg/kg) was given by gavage 5 times every second day prior to, and 5 times every second day after, 5.7 Gy irradiation. (a) Weight of spleen. (b) CFUs. Data are presented as M ± SD. ****p < .0001. n = 12 animals per group.
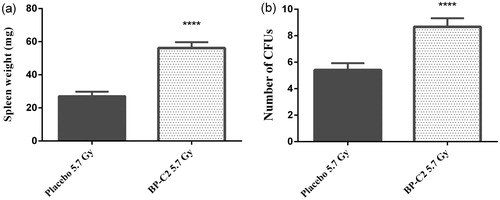
Effects of BP-C2 on C57BL/6 mice after TBI
Survival of irradiated C57BL/mice
On day 8, in the placebo groups irradiated with 5.0, 6.0, 7.0 and 8.0 Gy, animal survival was 100%, 60%, 10% and 0% (), respectively. Survival of animals treated with 81.0 mg/kg BP-C2, starting 24 h after irradiation, was 100%, 50%, 50% and 10% at 5.0, 6.0, 7.0 and 8.0 Gy, respectively (). Difference in the mean survival time was statistically significant only after 7.0 Gy and constituted 5.5 ± 0.4 days in the placebo group and 7.0 ± 0.4 days in the BP-C2 group (p < .05).
Table 3. Effect of BP-C2 on the survival of C57BL/6 mice on day 8 after total body irradiation.
Hematological observations
By day 8, the number of nucleated bone marrow cells in irradiated animals was significantly reduced compared to the false-irradiated animals (). In groups irradiated with 5.0 Gy and 6.0 Gy, the number of nucleated cells was reduced by a factor 5–6 and 9–10, respectively. Differences between the placebo and BP-C2 groups were not statistically significant.
Figure 3. Effect of BP-C2 on nucleated bone marrow cell count in C57BL/6 mice on day 8 after TBI. BP-C2 (81 mg/kg) was given by gavage daily 7 times starting 24 h after irradiation. Data are presented as M ± SD. ****p < .0001 versus control.
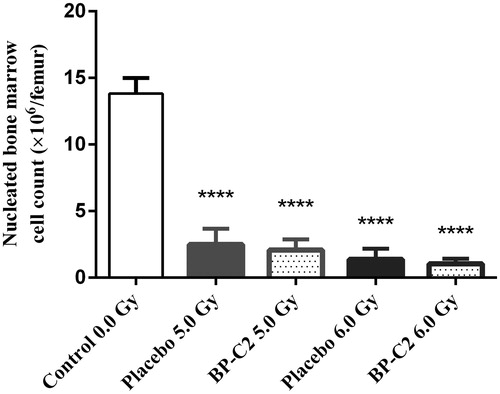
An approximately 10–15-fold reduction in whole blood count values was registered in the post-radiation period (data not presented). Differences between the placebo and BP-C2 groups were not statistically significant.
Curves, representing the relationship between radiation dose and nucleated bone marrow cell survival on day 8, were produced based on data from the analysis of ionizing radiation’s effect on bone marrow nucleated cell count (). In the placebo and BP-C2 groups, D0 – the dose at which the fraction of live cells is reduced e times compared to the initial value – did not differ significantly and was 1.32–1.86 Gy. At the same time, extrapolation coefficient n, which is the point of intersection of the ordinate axis with its exponential segment of the survival curve, was 2.5 times higher in animals treated with BP-C2 than in the placebo group animals: 7.0 and 2.8, respectively. This explains the higher quasi-threshold dose (Dq), representing cells’ self-repair capacity, in the BP-C2 group compared to the placebo group: 2.6 Gy and 1.9 Gy, respectively.
Figure 4. Survival curves for nucleated bone marrow cells. Effect of therapeutic administration of BP-C2 on survival of nucleated bone marrow cells in C57BL/6 mice on day 8 after irradiation. (a) Placebo groups, (b) BP-C2 (81 mg/kg) groups. Dq – quasi-threshold dose representing the cells’ self-repair capacity, n – extrapolation coefficient.
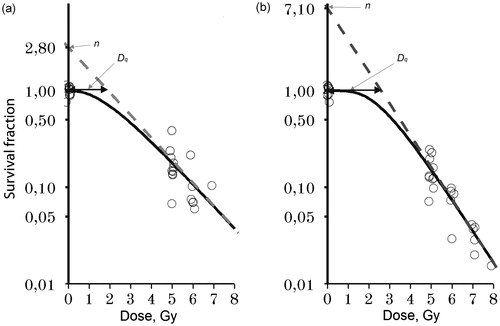
A reduction in spleen weight was recorded in irradiated animals on day 8 (). Spleen weight in false-irradiated animals was 94.0 ± 36.7 mg, while it was decreased by a factor of 2.5–3.4 in animals irradiated with 5.0–8.0 Gy. The differences in the change in spleen weight between BP-C2 and the placebo group did not reach the level of statistical significance. At the same time point, number of spleen colonies formed from surviving hematopoietic stem cells () in animals treated with BP-C2 was about twice that of the placebo group animals. After 5.0 Gy TBI, the number of CFUs was 6.7 ± 5.1 in the BP-C2 group and 2.3 ± 2.3 in the placebo group (p = .0245).
Figure 5. Effect of BP-C2 on weight of spleen and number of CFUs in C57BL/6 mice on day 8 after TBI. BP-C2 (81 mg/kg) was given by gavage daily 7 times starting 24 hours after irradiation. (a) Weight of spleen. (b) CFUs. Data are presented as M ± SD. Control group – 0.0 Gy n = 10; placebo groups – 5.0 Gy n = 10, 6.0 Gy n = 6; BP-C2 groups – 5.0 Gy n = 10, 6.0 Gy n = 5. CFUs: spleen colony-forming units; TBI: total body irradiation; n: number of animals. ****p < .0001 versus control. ***p = .0002 versus Control. #p = .0245 versus placebo 5.0 Gy.
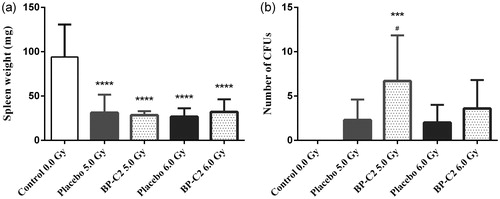
Effect on survival, cell proliferation and intestinal crypt stem cells
Radiation doses above 8.0 Gy are known to induce gastrointestinal radiation syndrome, the severity of which can be evaluated through morphologic analysis of surviving intestinal crypts (SIC). The results of the crypt survival analysis performed on samples collected on day 8 after irradiation of animals with 5.0–8.0 Gy are presented in . By this time point, a comparison of the false-irradiated animals and the irradiated animals can be used to evaluate the effect of the test agent on both, intestinal crypt survival and intestinal crypt repair (Booth et al. Citation2012).
Figure 6. Effect of BP-C2 on number of SIC in C57BL/6 mice on day 8 after TBI. BP-C2 (81 mg/kg) was given by gavage daily starting 24 h after irradiation 7 times. Data are presented as M ± SD. Control group – 0.0 Gy n = 10 (100), placebo groups – 5.0 Gy n = 10 (90), 6.0 Gy n = 6 (60), 7.0 Gy n = 1 (10), BP-C2 groups – 5.0 Gy n = 10 (83), 6.0 Gy n = 5 (48), 7.0 Gy n = 5 (46), 8.0 Gy n = 1 (10). n – number of animals (number of intestinal cross-sections scored). ***, ****p < .001, p < .0001 versus Control. ##p = .0023 BP-C2 5.0 Gy versus placebo 5.0 Gy, ####p < .0001 BP-C2 6.0 Gy versus. placebo 6.0 Gy.
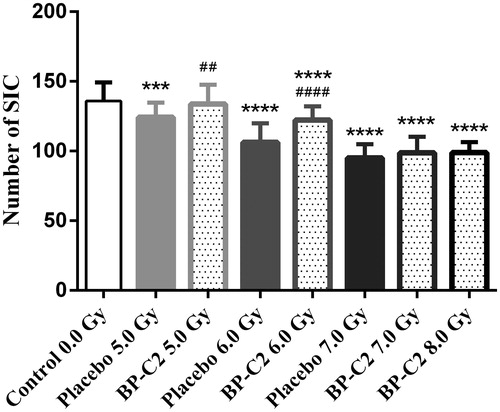
After 5.0 Gy TBI, the number of SIC in the BP-C2 and placebo groups was 133.7 ± 13.9 and 124.2 ± 10.5, p = .0023, respectively. At 6.0 Gy, BP-C2 also increased the number of SIC (122.2 ± 9.8 vs. 106.2 ± 13.7, p < .0001). At higher TBI doses, CFUS and SIC could not be evaluated because the experimental animals died prematurely.
Anti-Lgr5 staining revealed small cells in the base of crypts and, in some cases, at the +4 position. Their average number per crypt was gradually lower in groups irradiated with 5.0 Gy to 8.0 Gy, with a statistically significant difference between the control and BP-C2 groups at each irradiation dose (except for the 8.0 Gy group, since there were no surviving animals by day 8) ().
Figure 7. Effect of BP-C2 on Lgr5 + cells of intestinal crypts in C57BL/6 mice on day 8 after TBI. (a) Control 0.0 Gy; (b) Placebo 5.0 Gy; (c) BP-C2 (81 mg/kg) 5.0 Gy; (d) Placebo 7.0 Gy (note marked loss of intestinal crypts along with depletion of Lgr5 + cells); (e) BP-C2 (81 mg/kg) 7.0 Gy; (f) Number of Lgr5 + cells of intestinal crypts (data are presented as M ± SEM). **p < .01, ****p < .0001 versus Control, ###p < .001, ####p < .0001 BP-C2 group versus placebo group. Scale bar – 500 μm.
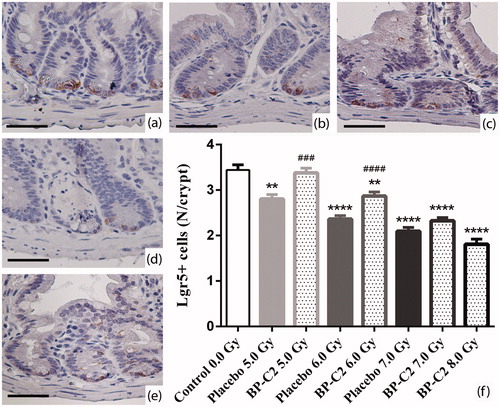
Cells positive for PCNA were found along the whole crypt, but predominately at its base and at positions 4–10. No significant change in the amount of PCNA + intestinal epithelial cells was observed by day 8 in any of the groups ().
Discussion
BP-C2 was tested in two TBI murine models (CBA and C57BL/6 mice) with intermediate sensitivity to radiation (Yuhas and Storer Citation1969). The effects in CBA strain were more evident in the mid-lethal than in the lethal radiation dose range (DRF = 1.1). Also, on day 8 after irradiation, BP-C2, administered to C57BL/6 mice 24 h after 7.0 Gy of irradiation, improved animal survival by 40% and mean survival time (MST) by 1.5 days. This type of pattern is common for radiomitigators, which in general have a low DRF and are efficient mostly in the mid-lethal range of radiation doses (Vasin Citation2014; Singh et al. Citation2014a; Rosen et al. Citation2014). Such agents exert a systemic effect, accelerating recovery of radiosensitive tissues after exposure to radiation. Radiomitigators are a diverse group of agents, comprising hormonal compounds (progesterones, estrogens) and similarly cytokines and immunomodulators. Many radiomitigators are of polyphenolic origin; for instance, BIO-300 or genistein (4′,5,7-trihydroxyisoflavone) demonstrates radioprotective efficacy in a CD2F1 murine model of ARS (DRF 1.16), protecting bone marrow progenitor cell populations (Davis et al. Citation2007; Grebeniuk et al. Citation2013) and exhibiting anti-inflammatory effects (Comalada et al. Citation2006). Some protective effects have been reported for compounds with structures similar to BP-C2: on the hematopoietic system, rosmarinic acid (Xu et al. Citation2016) and resveratrol (Ma et al. Citation2013); on γ-irradiated mouse skin, curcumin (Jagetia and Rajanikant Citation2015). An antioxidant effect is proposed as the key mechanism of action of such compounds.
Another key mechanism of action of radiomitigators may involve immune modulation, given that after radiation exposure the tissue suffers ongoing cell death, cytokine release and associated effects and hypoxia (Bentzen Citation2006). For instance, 5-AED (androstenediol) (Singh et al. Citation2005), CBLB502 (Entolimod) (Krivokrysenko et al. Citation2012) and gamma-tocotrienol (vitamin E isomer (Ghosh et al. Citation2009)) have been demonstrated to induce elevated levels of G-CSF and IL-6.
In studies performed in human PBMC culture, the polyphenolic ligand of BP-C2 was found to have some immunomodulatory effects (unpublished data) that may be related to the ameliorating effect of BP-C2 on radiation-induced hematological acute radiation syndromes. The results of our current study, namely the increased spleen weight and number of CFUs in animals treated with BP-C2, demonstrate the stimulatory effect of combined prophylactic/therapeutic as well as therapeutic administration of BP-C2 on extramedullar hematopoiesis in CBA and C57BL/6 murine strains.
BP-C2 activity was not associated with BP-C2’s effect on blood count or bone marrow cellularity, but with its ability to stimulate the repair of progenitor cells, as assessed by comparing the value of the quasi-threshold radiation dose (Dq) in the BP-C2 and the placebo groups. The Dq value, calculated based on curves representing the relationship of nucleated bone marrow cell mortality to radiation dose, was 1.4 times higher in the BP-C2 group than in the placebo group. This effect may be associated with mobilization of bone marrow progenitor cells in response to cytokine regulation by BP-C2. Many cytokines and growth factors, such as IL-6, IL-1m and GM-CSF, have been reported to affect the survival and differentiation of progenitor cells (Bodine et al. Citation1991; Singh et al. Citation2014b). The cytokines, reported to be involved in acquired radioresistance that do not affect hematopoiesis but do induce CFU formation, are IL-4 and IL-11 (Yonezawa et al. Citation2004).
In animal species exposed to radiation doses above 8.0 Gy, hematological radiation syndrome is usually accompanied by gastrointestinal (GI) syndrome, which is generally the factor that complicates treatment of damages induced by lethal radiation doses (Deng et al. Citation2015). In our study, the increased number of surviving intestinal crypts in animals treated with BP-C2 in the 5.0–8.0 Gy dose range appears to have resulted from the GI-protective effect of this compound. This effect may be a result of the protection of intestinal stem cells, as the number of lgr5 + mitotically active cells in irradiated animals treated with BP-C2 was maintained. However, this effect was not associated with a significant proliferation rate change, as measured by PCNA marker in intestinal crypts by day 8 after TBI. BP-C2 may act by inducing production of IL-22 by group 3 innate lymphoid cells (ILC3s) in response to IL-1β (Cella et al. Citation2010; Hughes et al. Citation2010) and subsequently stimulating the proliferation of intestinal epithelial cells. ILC3s, which are located in the immediate proximity of intestinal crypts and required for epithelial activation and proliferation, are also known to be responsible for controlling the development of secondary bacterial inflammations (Hazenberg and Spits Citation2014). These cells are considered to be the key mechanism of protection from cytotoxic damage and may be the target of compounds that reduce GI complications caused by antitumor chemotherapy (Aparicio-Domingo et al. Citation2015). A significant reduction in GI-associated side effects was previously reported in a case study of BP-C2 administered orally to a cancer patient as a supportive care drug in combination with FOLFIRINOX (Ibrahim et al. Citation2015)).
Our animal experiments have demonstrated that BP-C2, a novel lignin-derived polyphenolic composition with ammonium molybdate, exhibits a radiomitigating effect in the mid-lethal range of radiation doses in two mouse strains. The pharmacological activity of BP-C2 is apparently mediated through stimulation of the self-repair capacity of hematopoietic progenitor cells, the protective effect on the intestinal epithelium, and the enhancement of extramedullar hematopoiesis in the spleen.
An ideal radiation countermeasure should have several important properties: be effective, nontoxic, that is, not to induce side effects, be suitable for oral intake, be available and stable, i.e. providing good storage and easy transfer. Despite many candidates under different stages of the development no such countermeasure is currently available (Singh et al. Citation2014a). The BP-C2 is effective in mid-dose range of radiation, is safe with LD50 = 8000 mg/kg in mice, is intended for oral use, is easy to handle, can be stored at room temperature and have a shelf life over 2 years. Though the initial aim was to develop BP-C2 for treatment of acute radiation syndromes, scientific evidence gathered by us so far suggests that this agent may be more effective in the mid-lethal range of radiation doses. This fact, along with the convenient oral dosage form and low toxicity, merit development of this agent for people chronically exposed to low-mid doses of radiation. One of the indications BP-C2 is developed for is the supportive care of cancer patients receiving radio- or chemotherapy, associated with such side effects as nausea, vomiting and diarrhea. Amifostine is currently approved and used in clinical studies as a radioprotector and is claimed to reduce the risk of developing Grade 3-4 mucositis, but it is also known to induce some side effects, including nausea, emesis, hypotension and allergic reactions (Gu et al. Citation2014). Complementary studies to evaluate effect of BP-C2 on normal tissues and tumor radiosensitivity are underway to pursue its further development.
Notes on contributors
Prof. Vladimir N. Bykov, MD, PhD, Department of Carcinogenesis and Oncogerontology, PNMRCO, Saint-Petersburg, Russia.
Igor S. Drachev, MD, PhD, Department of Carcinogenesis and Oncogerontology, PNMRCO, Saint-Petersburg, Russia.
Sergey Yu. Kraev, PhD student, Laboratory of Carcinogenesis and Aging, PNMRCO, Saint-Petersburg, Russia.
Mikhail A. Maydin, researcher, laboratory for Carcinogenesis and Aging, PNMRCO, Saint-Petersburg, Russia.
Ekaterina A. Gubareva, researcher, laboratory for Carcinogenesis and Aging, PNMRCO, Saint-Petersburg, Russia.
Sergey E. Pigarev, CEO, Meabco A/S, Copenhagen, Denmark.
Prof. Vladimir N. Anisimov, MD, PhD, Head of the Department of Carcinogenesis and Oncogerontology, PNMRCO, Saint-Petersburg, Russia.
Prof. Irina A. Baldueva, MD, PhD, Head of the Department of Oncoimmunology, PNMRCO, Saint-Petersburg, Russia.
Elena I. Fedoros, PhD, Senior Researcher, Department of Carcinogenesis and Oncogerontology, PNMRCO, Saint-Petersburg, Russia and R&D Director Meabco A/S, Copenhagen, Denmark.
Andrey V. Panchenko, MD, PhD, Head of the Laboratory for Carcinogenesis and Aging, PNMRCO, Saint-Petersburg, Russia.
Disclosure statement
The authors report no conflicts of interest. The authors alone are responsible for the content and writing of the paper.
Additional information
Funding
References
- Ale-Ebrahim M, Eidi A, Mortazavi P, Tavangar SM, Tehrani DM. 2015. Hepatoprotective and antifibrotic effects of sodium molybdate in a rat model of bile duct ligation. J Trace Elem Med Biol. 29:242–248.
- Aparicio-Domingo P, Romera-Hernandez M, Karrich JJ, Cornelissen F, Papazian N, Lindenbergh-Kortleve DJ, Butler JA, Boon L, Coles MC, Samsom JN. 2015. Type 3 innate lymphoid cells maintain intestinal epithelial stem cells after tissue damage. J Exp Med. 212:1783–1791.
- Barker N, van Oudenaarden A, Clevers H. 2012. Identifying the stem cell of the intestinal crypt: strategies and pitfalls. Cell Stem Cell. 11:452–460.
- Bentzen SM. 2006. Preventing or reducing late side effects of radiation therapy: radiobiology meets molecular pathology. Nat Rev Cancer. 6:702–713.
- Bodine DM, Crosier PS, Clark SC. 1991. Effects of hematopoietic growth factors on the survival of primitive stem cells in liquid suspension culture. Blood. 78:914–920.
- Booth C, Tudor G, Tudor J, Katz BP, MacVittie TJ. 2012. Acute gastrointestinal syndrome in high-dose irradiated mice. Health Phys. 103:383–399.
- Cella M, Otero K, Colonna M. 2010. Expansion of human NK-22 cells with IL-7, IL-2, and IL-1beta reveals intrinsic functional plasticity. Proc Natl Acad Sci USA. 107:10961–10966.
- Comalada M, Ballester I, Bailón E, Sierra S, Xaus J, Gálvez J, de Medina FS, Zarzuelo A. 2006. Inhibition of pro-inflammatory markers in primary bone marrow-derived mouse macrophages by naturally occurring flavonoids: analysis of the structure-activity relationship. Biochem Pharmacol. 72:1010–1021.
- Dainiak N. 2002. Hematologic consequences of exposure to ionizing radiation. Exp Hematol. 30:513–528.
- Davis TA, Clarke TK, Mog SR, Landauer MR. 2007. Subcutaneous administration of genistein prior to lethal irradiation supports multilineage, hematopoietic progenitor cell recovery and survival. Int J Radiat Biol. 83:141–151.
- Deng W, Kimura Y, Gududuru V, Wu W, Balogh A, Szabo E, Thompson KE, Yates CR, Balazs L, Johnson LR, et al. 2015. Mitigation of the hematopoietic and gastrointestinal acute radiation syndrome by octadecenyl thiophosphate, a small molecule mimic of lysophosphatidic acid. Radiat Res. 183:465–475.
- Fares F, Fares B, Azzam N, Nashashibi M, Nevelsky A, Larsen S, Lindkaer-Jensen S. 2015. An innovative complex of benzene-poly-carboxylic acid and molybdenum, for prevention and treatment of radiation dermatitis. Med Chem. 5:447–451.
- Gajowik A, Dobrzyńska MM. 2014. Lycopene - antioxidant with radioprotective and anticancer properties. A review. Rocz Panstw Zakl Hig. 65:263–271.
- Ghosh SP, Kulkarni S, Hieber K, Toles R, Romanyukha L, Kao TC, Hauer-Jensen M, Kumar KS. 2009. Gamma-tocotrienol, a tocol antioxidant as a potent radioprotector. Int J Radiat Biol. 85:598–606.
- Grebeniuk AN, Basharin VA, Tarumov RA, Aksenova NV, Nazarov VB, Kovtun VIu, Chekunov IE. 2013. The experimental evaluation of antiradiation effectiveness of genistein by survival rates and bone marrow hemopoiesis of mice irradiated by X-rays. Radiats Biol Radioecol. 53:468–474.
- Greenberger J. 1991. Toxic effects on the hematopoietic microenvironment. Exp Hematol. 19:1101.
- Gu J, Zhu S, Li X, Wu H, Li Y, Hua F. 2014. Effect of amifostine in head and neck cancer patients treated with radiotherapy: a systematic review and meta-analysis based on randomized controlled trials. PLoS One. 9:e95968.
- Hazenberg MD, Spits H. 2014. Human innate lymphoid cells. Blood 124:700–709.
- Hua G, Thin TH, Feldman R, Haimovitz-Friedman A, Clevers H, Fuks Z, Kolesnick R. 2012. Crypt base columnar stem cells in small intestines of mice are radioresistant. Gastroenterology. 143:1266–1276.
- Hughes T, Becknell B, Freud AG, McClory S, Briercheck E, Yu J, Mao C, Giovenzana C, Nuovo G, Wei L, et al. 2010. Interleukin-1beta selectively expands and sustains interleukin-22+ immature human natural killer cells in secondary lymphoid tissue. Immunity 32:803–814.
- Ibrahim T, Larsen S, Lindkaer Jensen NH, Lindkaer Jensen S. 2015. BP-C2 improves functional status, quality of life and corrects biochemical imbalances as adjuvant therapy to FOLFIRINOX treatment: a case of advanced inoperable pancreatic cancer. J Clin Case Rep. 5:514.
- Jagetia GC, Rajanikant GK. 2015. Curcumin stimulates the antioxidant mechanisms in mouse skin exposed to fractionated γ-irradiation. Antioxidants (Basel). 4:25–41.
- Jeong BK, Song JH, Jeong H, Choi HS, Jung JH, Hahm JR, Woo SH, Jung MH, Choi BH, Kim JH, et al. 2016. Effect of alpha-lipoic acid on radiation-induced small intestine injury in mice. Oncotarget 7:15105–15117.
- Krivokrysenko VI, Shakhov AN, Singh VK, Bone F, Kononov Y, Shyshynova I, Cheney A, Maitra RK, Purmal A, Whitnall MH, et al. 2012. Identification of granulocyte colony-stimulating factor and interleukin-6 as candidate biomarkers of CBLB502 efficacy as a medical radiation countermeasure. J Pharmacol Exp Ther. 343:497–508.
- Kuntić VS, Stanković MB, Vujić ZB, Brborić JS, Uskoković-Marković SM. 2013. Radioprotectors - the evergreen topic. Chem Biodivers. 10:1791–1803.
- Ma Z, Huang H, Zhang Y, Yang Z. 2013. [Anti-radiation effect of resveratrol]. Zhong Nan Da Xue Xue Bao Yi Xue Ban. 38:597–601.
- McCulloch EA, Till JE. 1960. The radiation sensitivity of normal mouse bone marrow cells, determined by quantitative marrow transplantation into irradiated mice. Radiat Res. 13:115–125.
- Metcalfe C, Kljavin NM, Ybarra R, de Sauvage FJ. 2014. Lgr5+ stem cells are indispensable for radiation-induced intestinal regeneration. Cell Stem Cell. 14:149–159.
- Miura N, Satoh M, Imura N, Naganuma A. 1998. Protective effect of bismuth nitrate against injury to the bone marrow by gamma-irradiation in mice: possible involvement of induction of metallothionein synthesis. J Pharmacol Exp Ther. 286:1427–1430.
- Panchenko AV, Fedoros EI, Pigarev SE, Bykov VN, Drachev IS, Kraev SYu. 2017. [Experimental study of the radioprotective efficacy of a complex of polyphenolic polymeric lignin derivative with ammonium molybdate BP-C2]. Radiats Biol Radioecol. 57:505–511.
- Plett PA, Sampson CH, Chua HL, Joshi M, Booth C, Gough A, Johnson CS, Katz BP, Farese AM, Parker J, et al. 2012. Establishing a murine model of the hematopoietic syndrome of the acute radiation syndrome. Health Phys. 103:343–355.
- Rosen EM, Day R, Singh VK. 2014. New approaches to radiation protection. Front Oncol. 4:381.
- Shipov VP, Pigarev ES, Fedoros EI, inventors. 2013. RDInnovation APs, applicant. Oct 03. Benzene polycarboxylic acid compounds and their use as drug. WO2013143549 (A1).
- Singh VK, Newman VL, Romaine PL, Wise SY, Seed TM. 2014a. Radiation countermeasure agents: an update (2011–2014)). Expert Opin Ther Pat. 24:1229–1255.
- Singh VK, Romaine PL, Newman VL, Seed TM. 2014b. Tocols induce G-CSF and mobilise progenitors that mitigate radiation injury. Radiat Prot Dosimetry. 162:83–87.
- Singh VK, Romaine PL, Seed TM. 2015. Medical countermeasures for radiation exposure and related injuries: characterization of medicines, FDA-Approval Status and Inclusion into the Strategic National Stockpile. Health Phys. 108:607–630.
- Singh VK, Shafran RL, Inal CE, Jackson WE III, Whitnall MH. 2005. Effects of whole-body gamma irradiation and 5-androstenediol administration on serum G-CSF. Immunopharmacol Immunotoxicol. 27:521–534.
- Sorenson JR. 1984. Bis (3, 5-diisopropyl-salicylato) copper (II), a potent radioprotectant with syperoxide dismutase mimetic activity. J Med Chem. 27:1747–1749.
- Till JE, McCulloch EA, Siminovitch L. 1964. A stochastic model of stem cell proliferation, based on the growth of spleen colony-forming cells. Proc Natl Acad Sci USA. 51:29–36.
- Vasin MV. 2014. Comments on the mechanisms of action of radiation protective agents: basis components and their polyvalence. Springerplus. 3:414.
- Withers HR, Elkind MM. 1970. Microcolony survival assay for cells of mouse intestinal mucosa exposed to radiation. Int J Radiat Biol Relat Stud Phys Chem Med. 17:261–267.
- Xu W, Yang F, Zhang Y, Shen X. 2016. Protective effects of rosmarinic acid against radiation-induced damage to the hematopoietic system in mice. J Radiat Res. 57:356–362.
- Yonezawa M, Horie K, Kondo H, Kubo K. 2004. Increase in endogenous spleen colonies without recovery of blood cell counts in radioadaptive survival response in C57BL/6 mice. Radiat Res. 161:161–167.
- Yuhas JM, Storer JB. 1969. On mouse strain differences in radiation resistance: hematopoietic death and the endogenous colony-forming unit. Radiat Res. 39:608–622.
- Zbikowska HM, Szejk M, Saluk J, Pawlaczyk-Graja I, Gancarz R, Olejnik AK. 2016. Polyphenolic-polysaccharide conjugates from plants of Rosaceae/Asteraceae family as potential radioprotectors. Int J Biol Macromol. 86:329–337.