Abstract
Background
High dose ionizing radiation exposure is associated with myelo-depression leading to pancytopenia and the expected clinical manifestations of acute radiation syndrome (ARS). Herein, we evaluated the efficacy of sargramostim (Leukine®, yeast-derived rhu GM-CSF), with regimens delivered at 48, 72, 96, or 120 h after radiation exposure.
Methods
A randomized and blinded nonhuman primate (NHP) study was conducted to assess the effects of sargramostim treatment on ARS. NHPs were exposed to total body radiation (LD83/60 or lethal dose 83% by Day 60) and were randomized to groups receiving daily subcutaneous dosing of sargramostim starting from either 48, 72, 96, or 120 h post-irradiation. Additionally, separate groups receiving sargramostim treatment at 48 h post-irradiation also received prophylactic treatment with azithromycin. Sargramostim treatment of each animal continued until the preliminary absolute neutrophil count (ANC) returned to ≥1000/μL post-nadir for three consecutive days or the preliminary ANC exceeded 10,000/μL, which amounted to be an average of 15.95 days for all treatment groups. Prophylactic administration of enrofloxacin was included in the supportive care given to all animals in all groups. All animals were monitored for 60 days post-irradiation for mortality, hematological parameters, and sepsis.
Results
Delayed sargramostim treatment at 48 h post-irradiation significantly reduced mortality (p = .0032) and improved hematological parameters including neutrophil but also lymphocyte and platelet counts. Additional delays in sargramostim administration at 72, 96, and 120 h post-irradiation were also similarly effective at enhancing the recovery of lymphocyte, neutrophil, and platelet counts compared to control. Sargramostim treatment also improved the survival of the animals when administered at up to 96 h post-irradiation. While sargramostim treatment at 48 h significantly reduced mortality associated with sepsis (p ≤ .01), the additional prophylactic treatment with azithromycin did not have clinically significant effects.
Conclusion
In a NHP ARS model, sargramostim administered starting at 48 h post-radiation was effective to improve survival, while beneficial hematological effects were observed with sargramostim initiated up to 120 h post exposure.
Introduction
While accidental exposure to high radiation doses is infrequent, the potential to elicit large-scale adverse events makes the strategic preparedness planning for the medical management of radiation exposure a critical concern for health professionals and governmental agencies (DiCarlo et al. Citation2011). Individuals exposed to total body irradiation (TBI) or significant partial-body irradiation at exposures greater than 1 Gy may develop acute radiation syndrome (ARS) with significant hematopoietic consequences (Weinstock et al. Citation2008; Dorr and Meineke Citation2011). Radiation induced pancytopenia are resultant of the relative high radiosensitivity of the committed hematopoietic progenitors in the maturation pool (Inoue et al. Citation1995; Goans et al. Citation1997; Dainiak Citation2002; Waselenko et al. Citation2004). Myelosuppression after radiation exposure can lead to hemorrhages, an immunocompromized state, and subsequent infections. As such, the reconstitution or protection of the hematopoietic cells is a priority during patient management (Gianni et al. Citation1989; Laterveer et al. Citation1996; Singh and Seed Citation2017). One of the primary and recommended approaches for the treatment of ARS is the prompt administration of leukocyte growth factors (LGFs) (Smith et al. Citation2006), which shorten the duration of neutropenia after irradiation and thus reduce the risks for opportunistic infections with the goal of potentially improving survival. However, until recently, there were limited data to inform use of LGFs following a nuclear incident and no U.S. Food and Drug Administration (FDA) approved LGF medical countermeasures (MCM) (Singh et al. Citation2015). Nonhuman primate (NHP) models have been validated for use in evaluation of potential treatments of hematopoietic-ARS (H-ARS) in accordance with the U.S. FDA ‘Animal Rule’ guidance (US FDA Citation2019). NHP mortality rate at day 60 was established as the primary endpoint of interest, since this is clearly related to the desired benefit in humans, while shortening time to absolute neutrophil count (ANC) recovery is a useful supportive endpoint.
There are now three ARS MCMs that have been approved by the U.S. FDA – the recombinant human granulocyte colony-stimulating factor (rhu G-CSF) filgrastim (Neupogen®) (Amgen Inc. Citation2018) and pegfilgrastim (Neulasta®) (Amgen Inc. Citation2019) were both approved in 2015, and the most recently approved granulocyte macrophage colony stimulating factor (GM-CSF) sargramostim (Leukine®) (Sanofi-Aventis Citation2018). The studies supporting these approvals have varied in the use of supportive care and timing of administration following irradiation. Only studies of sargramostim have included both male and female animals. Both filgrastim and pegfilgrastim have demonstrated efficacy in the NHP ARS model, showing improved survival rates compared to controls when administered 24 h after radiation with full supportive care including blood transfusions and individualized antibiotics (Farese et al. Citation2012, Citation2013; Hankey et al. Citation2015). MacVittie et al. also showed that delayed administration of filgrastim at up to 120 h had beneficial effects on shortening the duration of neutropenia and improving ANC nadirs in NHPs (MacVittie et al. Citation2015). However, subsequent studies showed that delayed administration of filgrastim and pegfilgrastim at 48 h post-irradiation did not significantly improve survival even with the same levels of supportive care (Farese et al. Citation2014; Gluzman-Poltorak et al. Citation2014). Since the availability of immediate supportive care is likely to be varied (especially in the administration of LGFs) in the aftermath of a catastrophic nuclear event, this lack of efficacy in delayed treatments raises concerns regarding the practical utility of the compounds.
Sargramostim, yeast derived rhu GM-CSF, is a potent cytokine that stimulates myeloid cell growth and maturation as well as immunologic reconstitution (Waller Citation2007). Sargramostim has been previously approved for treatment associated with neutropenia during cancer therapy to shorten the time to neutrophil and myeloid recovery (Mehta et al. Citation2015) and has been shown to accelerate leukocyte recovery in combination chemo/radiation therapies (Farese et al. Citation1993). More recently, sargramostim has been shown to significantly reduce the mortality rate in NHP models of ARS at a delayed administration time of 48 h post-irradiation exposure (Clayton et al. Citation2016). As well, incidences of secondary bacterial infections are also reduced with sargramostim treatment. This improvement was accompanied by neutrophil and lymphocyte recovery as well as enhanced platelet and reticulocyte counts following radiation exposure (Farese et al. Citation1993; Clayton et al. Citation2016). Herein, we report the evaluation of delayed sargramostim treatment at up to 120 h after irradiation to fully investigate its robustness as an ARS MCM.
Furthermore, the addition of a broad-spectrum antibiotic supplement along with medical treatment and countermeasures has been considered to be beneficial in reducing the incidence of infections and improving survival rates in the medical management of ARS. Irradiation studies using murine models have shown that the addition of broad-spectrum antibiotics can increase both the mean and total survival times in this species (Booth et al. Citation2012; Plett et al. Citation2012). Other studies in NHPs have also shown that the prophylactic use of antibiotics, combined with intensive medical management including whole blood transfusions to restore neutrophil recovery, worked to improve survival in the animals after exposure to lethal doses of radiation (Farese et al. Citation2013, Citation2014). However, the exact contribution of the use of prophylactic antibiotics to the reduction in mortality has not been empirically studied.
In this study, we evaluated the effects of sargramostim treatment, in a NHP model of ARS in a blinded and randomized good laboratory practice (GLP)-compliant study. We also evaluated the effect of azithromycin, a broad-spectrum antibiotic, when given with sargramostim. We presented results describing the effects of sargramostim treatment compared to vehicle treatment on survival, hematology, and microbiological analyses. We also characterized bacterial species that were identified and analyzed their antimicrobial resistance to help establish treatment strategies for future studies.
Materials and methods
Ethics statement
All experimental procedures were performed in compliance with the Good Laboratory Practice (GLP; 21, Code of Federal Regulations Part 58) and in accordance with Institutional Animal Care and Use Committee (IACUC) of Citoxlab North America, and the Canadian Council on Animal Care (CCAC) guidelines for use of experimental animals. All protocols included humane euthanasia criteria and were reviewed and approved by the IACUC. All animals were monitored continuously (i.e. technical staff and veterinarians were available on-site 24 h a day) for development of any untoward clinical signs.
Test and control items
Sargramostim (rhu GM-CSF, Leukine®) (manufactured by Sanofi-Aventis, Boston, MA at time of study) was stored from 2 to 8 °C. The vehicle control was sterile water for injection, USP (SWFI) (Baxter Healthcare Corporation, Deerfield, IL) purchased commercially and stored at room temperature. Sargramostim (250 µg/vial) was reconstituted fresh on each dosing occasion in SWFI to obtain a nominal concentration of 250 µg/mL. The solution was gently swirled at room temperature until complete dissolution of the test item and was placed on wet ice pending use.
Azithromycin (Zithromax®) (manufactured by Sandoz) was stored at room temperature. The azithromycin solution was prepared by mixing a sufficient volume of reverse osmosis water with the required amount of azithromycin as specified by the manufacturer in order to achieve a concentration of 40 mg/mL. The azithromycin solution was stored at room temperature without the use of preservatives and used within 7 days.
Animals
The animal room environment was maintained at a temperature of 21 ± 3 °C with a relative humidity of 50 ± 20%, a light dark cycle of 12 h light/12 h dark, and 10–15 air changes per hour. Temperature and relative humidity were monitored continuously.
A total of 308 (154 males/154 females) rhesus monkey (Macaca mulatta) NHPs, aged 2.7–5.8 years and weighing 3.1–7.0 kg and 2.9–6.6 kg for males and females, respectively, were included in the study. NHPs were fed a standard certified commercial chow (ENVIGO Teklad Certified Hi-Fiber Primate Diet #7195 C in form of cookies) twice daily. Treats or fruits/vegetables were provided as part of the animal enrichment program. Water (which had been exposed to ultraviolet light and purified by reverse osmosis) was provided to the animals ad libitum. This study was conducted outside of the breeding season for Rhesus monkeys.
Radiation exposure
To minimize stress due to handling procedures, animals were acclimated to the radiotherapy restraining apparatus by pre-exposure training periods. Animals were transferred to the treatment facility in a transport vehicle with controlled temperature. Prior to transportation to the radiation facility, animals were acclimated on at least three occasions to the restraining apparatus and at least one occasion to transportation. Positive reinforcement was used to facilitate acclimation, and animals were not sedated during transport or irradiation. Animals were fasted overnight prior to whole-body irradiation, and fed after irradiation at the irradiation facility (i.e. a banana was given after irradiation to each animal) and upon returning to the housing unit to reduce stress.
NHPs were irradiated using a Cobalt-60 gamma source (Theratron® 1000; Best Theratronics; Ottawa, Ontario, Canada). Prior to irradiation (Day 1), the radiation conditions were calibrated using an acrylic phantom placed in the same experimental conditions as those to be used. Animals were irradiated in a random order (i.e. not in ascending group number) and in staggered cohorts over 18 days, with individual radiations taking place between the hours of 10 am and 4 pm.
During radiation, animals were placed in a chair in a symmetrical vertical position. Positioning was confirmed with linear laser markers. In order to produce homogenous dose distribution, treatment was divided in two fractions (i.e. antero-posterior followed by postero-anterior). Two dosimeters (Landauer, Inc. Model nanoDot) were placed on each animal during whole body irradiation to quantify the dose. The skin was shaved and one dosimeter was placed on the midplane approximately at the level of the xiphoid process and a second dosimeter was placed at the corresponding level in the dorsal area (below the interscapular area) on the day of irradiation. Dosimeters were placed under a gel bolus build-up of approximately 5 mm (superflab) in thickness and secured with bandaging and surgical glue. The dosimetry results (i.e. the average of frontal and dorsal) obtained from the Farmer electrode chamber were within +0.01% to +2.33% of the targeted radiation dose. NHPs were exposed to 713 cGy at 0.5 Gy min−1. The exposure time for each animal was calculated individually based on body dimensions as per facility standard operating procedures.
The irradiation dose level was selected based on available historical data (Farese et al. Citation2012) and Citoxlab North America studies to analyze the benefits of MCM administration on animals receiving a lethal dose of radiation. The irradiation dose level was also used during a previous study where an LD83/60 was obtained (unpublished data).
Supportive care
Prophylactic supportive care for all animals included sucralfate (1 g/day (0.5 g BID, PO) daily from days 5 to 30 (inclusive)), enrofloxacin during predicted periods of neutropenia (10 ± 0.5 mg/kg, subcutaneous, SID from Day 5 to Day 27 (inclusive)), ondansetron (1 mg/kg IM 30–90 min prior to irradiation and 30–45 min following irradiation to suppress emesis), and buprenorphine (approximately 0.01 mg/kg/dose BID, subcutaneous from Day 3 to 30).
Symptomatic supportive care based on individual animal evaluation consisted of analgesics (buprenorphine and/or bupivacaine), parenteral fluids (Ringer’s lactate with or without 5% dextrose and/or Pedialyte®/Gastrolyte®), anti-emetics (ondansetron), nutritional support (e.g. crushed cookies, fruit, juice, etc.), and cutaneous care (hydrotherapy, iodine, and/or sterile water flush). Symptomatic supportive care was provided to all NHPs as indicated by cage-side and clinical observations per the approved IACUC protocol.
Experimental design
The study was designed with reference to the following test methods and/or guidelines: U.S. FDA Guidance for Industry October 2015: Product Development Under the Animal Rule Guidance for Industry; and ICH S6 (R1): Preclinical Safety Evaluation of Biotechnology-Derived Pharmaceuticals.
Animal assignment
Male and female animals were separately assigned to dose groups by a randomized stratification system based on body weights and the animal providers, as applicable. A total of 308 NHPs (154 males/154 females) were randomized to the vehicle and sargramostim delayed treatment groups: 44 NHPs (22 M/22 F) per group were assigned to the reference item/vehicle (Group 1), 48 h (Group 2), 72 h (Group 3), 96 h (Group 4), and 120 h (Group 5) treatment groups. An additional 44 NHPs (22 M/22 F) per group were assigned to the reference item/vehicle + azithromycin (Group 6) and sargramostim 48 h + azithromycin (Group 7) groups.
Blinding
All study personnel were blinded to treatment assignment, with exception of the staff responsible for randomization, preparation of the test item, and dosing. The unblinded personnel were not involved in clinical evaluation or euthanasia decisions. The pathologist was blinded at the time of necropsy and initial macro- and microscopic evaluation.
Treatment and route of administration
The sargramostim (7 µg/kg/day) and reference item/vehicle were administered daily by subcutaneous injection between the scapulas. The first injection was performed 48, 72, 96, or 120 h ± 1 h post-end of irradiation and subsequent injections were administered at approximately the same time as the first treatment injection (± 3 h). For groups with treatments starting later than 48 h after irradiation (Groups 3, 4, and 5), the control was administered on days before the start of sargramostim to have the same level of handling procedures in all groups. Treatment of each animal continued until the preliminary ANC returned to ≥1000/μL post-nadir for three consecutive days or the preliminary ANC exceeded 10,000/μL. In the event that the ANC could not be obtained for a given day due to clotted sample (or any other reason), dosing was stopped when three consecutive results of preliminary ANC were ≥1000/μL. Interim analysis was not performed during this study to assess efficacy and/or futility.
Azithromycin was administered PO from Day 8 to Day 21 (inclusive) to Groups 6 and 7.
Survival
NHPs were evaluated at least twice daily during all phases of the study. At 60 days post-radiation exposure, surviving animals were humanely terminated and necropsies were completed. Animals in unrelievable pain or distress before Day 60 were humanely euthanized. Animals were euthanized if one of the following criteria was observed: respiratory distress, complete anorexia for 3 days with deteriorating conditions, loss of weight >20% of baseline for more than 3 days, hemorrhage in excess of 20% estimated blood volume, severe dehydration with hypothermia or hyperthermia, recumbent in the cage with decreased or absent responsiveness to touch, or in severe pain which could not be significantly alleviated with analgesia.
Hematology
Blood samples (300–500 µL) were obtained from a peripheral vein (avoiding the femoral vein between Days 6 and 24) in tubes containing K3-EDTA. Hematology evaluations (including neutrophil, platelet, WBC, RBC, and reticulocyte counts) were performed on all surviving animals twice prior to irradiation, and on Days 1–30 (inclusive), 35, 40, 45, 50, 55, and 60 after irradiation and prior to unscheduled euthanasia, when possible. Animals were manually restrained but not anesthetized for blood collection.
Blood culture
Blood samples (300–500 µL) were obtained from a peripheral vein (avoiding the femoral vein between Days 6 and 24) in tubes containing K3-EDTA. Hemocultures were performed on all animals when febrile neutropenia was identified (ANC <0.5 × 109/L, rectal temperature ≥ at 103 °F/39.4 °C) and at euthanasia; however, animals were not sampled more than 2 times/week (excluding at euthanasia).
Bacteriology
The following tissues were collected at necropsy from all animals (including unscheduled terminations or moribund animals) for microbiological analysis: liver (medial lobe), lungs (right caudal lobe and left cranial lobe separately), spleen (part of remaining tissues), kidney (right), heart (apex), and brain (left hemisphere). Organ samples were placed on a sterile plate for manipulations. A selected area at the surface of the tissue sample was burned to eliminate possible surface contaminant. The burning of the area was performed by using a spatula soaked in 70% ethanol and heated by the gas burner flame until the spatula is red. A sterile culture swab was inserted in the tissue sample through the burned surface and inserted into a sterile tube for isolation and identification of aerobic and anaerobic bacteria and antibiogram. Culture swabs were stored refrigerated (2–8 °C) or kept on wet ice pending processing.
All microbiological analyses on blood and tissues collected were performed by IDEXX Laboratories (IDEXX Laboratories; Markham Ontario, Canada).
Clinical observations, body weight, and body temperature
Cage-side clinical signs were recorded on all animals at least twice daily throughout the study. A detailed clinical examination was performed on each animal prior to animal assignment, the day prior to irradiation, every 6 days thereafter, and prior to necropsy. Body weights were recorded for all animals prior to animal assignment, the day prior irradiation, every 3 days thereafter, and prior to necropsy. Body temperature (auricular) was taken on all animals the day prior to irradiation and every 3 days after irradiation until the end of the study, or when clinically justified and authorized by the clinical veterinarian.
Statistical design
The primary objective of the study was to evaluate the efficacy of sargramostim treatment initiated at 48, 72, 96, or 120 h post-irradiation versus the vehicle control in NHPs, as assessed by survival rate 60 days after TBI at an LD83/60 dose; additional endpoints of this study included hematology parameters and incidence of infection. The survival rate at Day 60 was defined as the proportion of animals that survived to Day 60 scheduled euthanasia. Secondary objectives were to evaluate the efficacy of the azithromycin and sargramostim combination on overall survival, hematology parameters, and incidence of infection.
Using 44 rhesus animals per group, the trial was expected to provide 80% power at a 2-sided alpha level of 5% to demonstrate a decreased mortality rate at Day 60 of 40% in the Leukine arm and 70% in the reference item/vehicle 48 h arm using a Log-Rank test and was expected to provide 80% power at a 1-sided alpha level of 2.5% to demonstrate a decreased mortality rate at Day 60 of 50% in the Leukine arm and 80% in the reference item/vehicle 48 h arm using a Fisher Exact test. The number of animals used in the study was approved by IACUC.
Plots of time to event (Kaplan-Meier) were presented (e.g. survival and for time to event data such as time to ANC recovery). Plots of the treatment means across time were presented for continuous numerical parameters when including all animals. For each dataset with more than two groups to compare, a one-way analysis of variance (ANOVA) was performed and the residuals were tested for normality using a Shapiro–Wilk test. When significant differences among the group means were indicated by the ANOVA overall F-test (p ≤ .05), a Dunnett test was used to perform the group mean comparisons between the reference item/vehicle 48 h group and each test item treated group.
When the Shapiro–Wilk test on the residuals of the second ANOVA was significant or when heterogeneous group variances (p ≤ .05) were revealed by the Levene test, then the ANOVA results were discarded and the groups were compared using a non-parametric Kruskal–Wallis test. When the Kruskal–Wallis test was significant (p ≤ .05), a Dunn test was used to perform the pairwise group comparisons between the reference item/vehicle 48 h group and each test item treated group.
For datasets with only two groups to compare (including the reference item/vehicle 48 h Group 1), a Shapiro–Wilk test and a Levene test were performed as described above but a two-sample t-test replaced the one-way ANOVA F-test, and a Wilcoxon rank-sum test replaced the Kruskal–Wallis test, while Dunnett and Dunn tests were not performed. Analyses were performed using version 9.2 of the SAS (1) system running on Windows 7.
Results
Sargramostim administration at 48 h post-irradiation significantly increased survival in NHPs
The logistic regression model for comparing the mortality frequencies at Day 60 showed an overall statistically significant dose effect (t-test, p = .0032), indicating that a sargramostim regimen starting at 48 h post-irradiation significantly reduced mortality (). The mortality rate observed on day 60 was 86% in Group 1 (reference item/vehicle 48 h) and 68% for Group 2 (sargramostim 48 h), showing a reduction of 18% (. This result corroborates the outcomes observed in previous studies (Clayton et al. Citation2016).
Figure 1. Survival curves and cause of death in NHPs after TBI and treatment with sargramostim and/or azithromycin. (A) Survival curve comparisons of Groups 1 (reference item/vehicle 48 h) and 2 (sargramostim 48 h). (B) Survival curve comparisons of Groups 6 (reference item/vehicle 48 h + azithromycin) and 7 (sargramostim 48 h + azithromycin). Logistic regression model for comparing the mortality frequencies at Day 60 showed an overall statistically significant dose effect (t-test, p = .0032). (C) Most likely cause of death of moribund animals in each group.
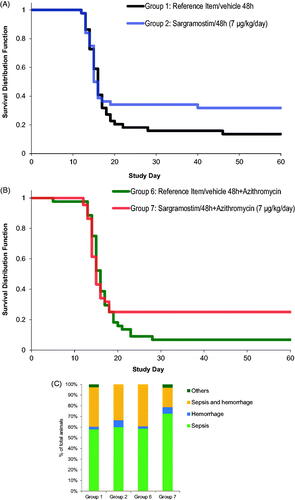
Additionally, the mortality rate at Day 60 was 93% for Group 6 (reference item/vehicle 48 h + azithromycin) and 75% for Group 7 (sargramostim 48 h + azithromycin), a reduction of 18% (. Although the animals dosed with sargramostim at 48 h with the addition of azithromycin (Group 7, 75%) had higher survival compared to animals dosed with only sargramostim (Group 2, 68%), this difference between groups (7%) did not reach statistical significance. The addition of azithromycin therefore had no statistically significant effect and there was no interaction between sargramostim and azithromycin. The most common cause of death in all moribund animals across groups was sepsis as determined by bacteriological analysis of organ and hemocultures (. The prophylactic administration of azithromycin added to enrofloxacin, which was provided prophylactically to all animals, had no significant effect on the incidences of sepsis across all groups under the conditions that prevailed in this study. This indicates that there may be preexisting resistance to the administered antibiotherapies in the commensal bacteria population of the NHPs in this study.
48 h post-irradiation sargramostim treatment improved hematological parameters compared to controls
A summary of the hematological parameters related to neutrophil and platelet data can be found in . Following irradiation, the neutrophil count in the animals decreased rapidly reaching an ANC <500/μL by a mean of 5.5–6.5 days, and ANC < 100/μL by a mean of 8.7–10.1 days after radiation exposure (. The recovery was initiated earlier in sargramostim-treated groups with or without azithromycin compared to controls (Groups 2 and 7, compared to Groups 1 and 6). Return to values similar or higher than baseline was achieved on Days 19 or 20 for all sargramostim-treated groups (Groups 2 and 7) and later on Day 22 for both reference item/vehicle 48 h groups (Groups 1 and 6, p ≤ .0001).
Figure 2. Changes in hematology in NHPs after exposure to radiation and treatment with sargramostim and/or azithromycin. (A) Mean (±SEM) absolute neutrophil (ANC) counts in NHPs after irradiation. ANC nadir was significantly lower in Group 7 compared to Group 2 (p≤.05). (B) Platelet count in NHPs after irradiation. Shown are the treatment means (±SEM) of platelet count × 109 cells/L. The log-rank test for comparing the recovery curves indicated a significant earlier recovery for the sargramostim group at 48 h compared to the reference item/vehicle 48 h (p ≤ .05). (C) White blood cell count in NHPs after irradiation. Shown are the treatment means (±SEM) of white blood cell count × 109 cells/L. (D) Erythrocytes absolute count in NHPs after irradiation. Shown are the treatment means (±SEM) of Erythrocytes absolute count × 109 cells/L. (E) Reticulocyte absolute count in NHPs after irradiation. Shown are the treatment means (±SEM) of reticulocyte absolute count × 109 cells/L. All analysis were performed using peripheral blood collected from NHPs with sargramostim and/or azithromycin administration (n = 44 per group; 22 males and 22 females). PI: prior to irradiation.
Table 1. Summary of neutrophil- and platelet-related hematology parameters.
Further analysis of neutrophil-related parameters revealed that, as expected, given the mechanism of action of the drug (Farese et al. Citation1993; Mehta et al. Citation2015; Clayton et al. Citation2016), the mean duration of neutropenia (ANC < 500/μL) was significantly shorter in sargramostim-treated animals (Group 2, 10.1 ± 1.8 days, p ≤ .001) compared to reference item/vehicle 48 h (Group 1, 13.3 ± 1.3 days) without azithromycin treatment. Mean duration of severe neutropenia (ANC < 100/μL) was also slightly shorter with sargramostim at 48 h (Group 2, 4.6 ± 2.9 days) compared to reference item/vehicle 48 h (Group 1, 6.0 ± 2.3 days), although this result did not reach statistical significance. Overall, there was no statistically significant effect of the addition of azithromycin on neutropenia (ANC < 500/μL) and severe neutropenia (ANC < 100/μL) duration.
Platelet count started to decrease for all groups on Days 5–7 (. The mean duration of platelet count ˂20,000/μL ranged from 4.1 to 6.3 days and was similar in all groups. The platelet nadir was slightly higher in Group 2 (sargramostim 48 h) compared to Group 1 (reference item/vehicle 48 h), and the recovery onset was noted earlier overall in sargramostim treated groups (Groups 2 and 7, p ≤ .05 and p ≤ .001, respectively). There was no clinically significant effect of azithromycin administration on platelet count. Platelet nadir and mean duration of severe thrombocytopenia were similar in both sargramostim treated groups with or without azithromycin (Groups 2 and 7).
As well, leukocyte counts decreased similarly in all groups (. Decreases started on Day 2 and values reached nadir on Day 15 for both reference item/vehicle 48 h groups (Groups 1 and 6) and one or two days earlier on Days 13 or 14 for sargramostim-treated groups (Groups 2 and 7). Afterward, recovery was initiated earlier in all sargramostim-treated groups. Recovery to values similar or higher than baseline was achieved on Day 20 or 21 for all sargramostim-treted groups and later on Day 23 for both reference item/vehicle 48 h groups.
Decreases in erythrocytes were noted starting on Day 6 or 7 in all groups (. Given the long biological half-life of red blood cells (Shrestha et al. Citation2016), this loss is likely associated with hemorrhages as an effect from irradiation. Mean erythrocyte counts were lower from approximately Days 8 to 26 and the nadir was observed slightly earlier in Group 2 (sargramostim at 48 h) compared to Group 1 (reference item/vehicle 48 h). No consistent differences in erythrocyte count were noted with the addition of azithromycin. Mean reticulocyte counts began to decline the day after irradiation reaching similar nadir on Days 7–9 in all groups (. This was followed by a compensatory increase up to approximately Day 12. The magnitude of the increase was greater in all sargramostim-treated groups (Groups 2 and 7), reaching statistical significance (p ≤ .05) on days 3, 6, 10–12, 17–25, and 30 (two-way ANOVA). A second moderate decline in reticulocyte count was observed in all groups afterward up to approximately Day 16. Afterward a marked increase (approximately 5 folds from baseline levels) was noted reaching mean reticulocyte counts above 400 × 109/L (baseline values were between approximately 66 and 81 × 109/L) in all sargramostim-treated groups (Groups 2 and 7) and much lower (between 115 and 290 × 109/L) in reference item/vehicle 48 h groups (Groups 1 and 6) by Day 24. Again, no consistent differences in reticulocyte count were noted with the addition of azithromycin.
Delayed sargramostim treatment up to 120 h after irradiation was still efficacious against radiation-induced leukopenia and neutropenia
To investigate the efficacy of delayed sargramostim administration, sargramostim was administered at 48, 72, 96, and 120 h post-irradiation in NHPs and hematology was monitored throughout the 60 day study period. Dosing was continued until the preliminary ANC returned to ≥1000/μL post-nadir for three consecutive days or the preliminary ANC exceeded 10,000/μL. Mean overall treatment times were found to be quite similar between the groups, with 16.34 ± 0.53 days for Group 1, 15.89 ± 0.47 days for Group 2, 16.02 ± 0.48 days for Group 3, 16.41 ± 0.51 days for Group 4, 15.68 ± 0.44 days for Group 5, 15.77 ± 0.52 days for Group 6, and 15.52 ± 0.45 days for Group 7. Lymphocyte counts declined rapidly immediately post-radiation followed by a sustained but less severe decrease, reaching lowest mean levels on Day 14 for all sargramostim-treated groups (Groups 2–5) and on Day 15 for the reference item/vehicle 48 h group (Group 1) (. Lymphocyte recovery was initiated earlier in all sargramostim-treated groups (Groups 2–5) compared to the reference item/vehicle 48 h group (Group 1), and there were no difference between the delayed sargramostim treatment groups in terms of overall changes in lymphocyte count.
Figure 3. Changes in white blood cell (WBC) count, neutrophil count, and survival after exposure to radiation and delayed treatment of 48 h, 72 h, 96 h, and 120 h with sargramostim. (A) Lymphocyte absolute count in NHPs after irradiation. Shown are the treatment means (±SEM) of lymphocyte count × 109 cells/L. (B) Mean (±SEM) ANC in NHPs after irradiation. (n = 44 per group; 22 males and 22 females). (C) Platelet count in NHPs after irradiation. Shown are the treatment means (±SEM) of platelet count × 109 cells/L (n = 44 per group; 22 males and 22 females). (D) Survival curve comparisons of Groups 1 (reference item/vehicle 48 h), 2 (sargramostim 48 h), 3 (sargramostim 72 h), 4 (sargramostim 96 h), and 5 (sargramostim 120 h).
As observed with the leukocyte count, neutrophil recovery was initiated earlier in sargramostim-treated groups (Groups 2–5) (. In Group 2 (sargramostim 48 h), the ANC nadir was also higher compared to Group 1 (reference item/vehicle 48 h), but did not reach statistical significance. This was not observed in all other sargramostim treatment groups (Groups 3–5). Additionally, the platelet nadir was slightly higher in Group 2 (sargramostim 48 h) compared to Group 1 (reference item/vehicle 48 h) but slightly lower in Groups 3, 4, and 5 (sargramostim 72, 96, and 120 h); however, no statistical significances were detected (. Similar to neutrophils, platelet recovery was also initiated earlier in all sargramostim treated groups. Sargramostim also appeared to improved survival when administer at up to 96 h post TBI (), although the effects did not reach statistical significance except for the 48 h treatment as presented previously (. Overall, delayed sargramostim treatment up to 120 h after irradiation was efficacious in improving leukocyte, neutrophil, and platelet recovery, with results that are comparable to those achieved by the earlier 48 h post-irradiation sargramostim treatment.
Sargramostim has significant beneficial effects on reducing the rate of sepsis post-irradiation
The vast majority (93%; 225/242 animals) of moribund animals presented with at least two positive results for bacteriology in organ and/or blood culture which was required for diagnosis of sepsis (. Treatment with sargramostim reduced the total number of deaths attributed to infection, reaching statistical significance when comparing pooled Groups 2 and 7 (sargramostim 48 h with and without azithromycin) with pooled Groups 1 and 6 (reference item/vehicle 48 h with and without azithromycin) (p ≤ .01) (. The number of animals with signs of sepsis was also significantly lower in the group treated with sargramostim at 48 h and azithromycin (Group 7; 30 animals) compared to the reference item/vehicle 48 h group with azithromycin (Group 6; 40 animals). The results were also similar to the sargramostim group without azithromycin (Group 2; 29 animals), indicating that administration of azithromycin had no significant beneficial effects on the rate of sepsis.
Figure 4. Percentage of animals with sepsis following irradiation and treatment with sargramostim and/or azithromycin. (A) Percentage of total animals across all groups, both moribund and surviving, presenting as septic or non-septic (n = 308). Septic is defined as animals presenting with at least two positive bacteriology results in organ and blood culture. (B) Percentage of total animals within each group, both moribund and surviving, presenting as septic or non-septic (n = 44 per group; 22 males and 22 females). Group 1 (reference item/vehicle 48 h); Group 2 (sargramostim 48 h); Group 6 (reference item/vehicle 48 h + azithromycin); Group 7 (sargramostim 48 h + azithromycin). **p≤.01.
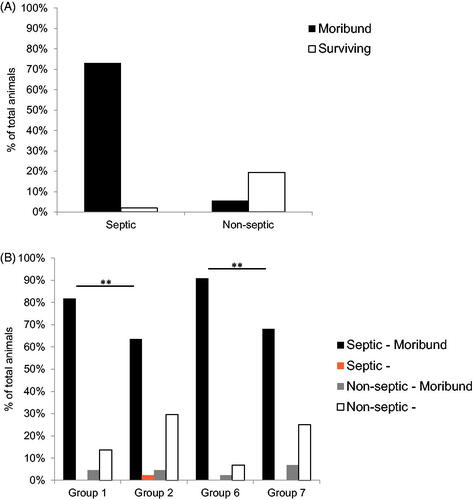
Characterization of bacteriological agents detected in organ and hemoculture of animals post-irradiation
Microbiological evaluations of organs and hemoculture analyses were performed on both moribund and surviving animals post mortem. A large majority of the moribund animals in all groups (from 55% to 91% of total animals in each group) had positive bacteriology results in the major organs, of which the liver and lungs (left and right caudal lobes) appeared to be slightly more susceptible compared to the brain, heart, spleen, and kidneys (. Of the isolated bacterial strains from organ and hemoculture, gram-positive species were the most frequently identified followed by a combination of gram-positive and -negative, and finally gram-negative only (. A list of all identified bacterial strains and their frequency can be found in .
Figure 5. Bacteriology of study animals post irradiation. (A) Percentage of total animals with at least one positive bacterial identification in the brain, heart, liver, lung (left and right caudal lobes), spleen, and kidneys post mortem. Moribund is defined as animals which were removed before endpoint of 60 days. (B) Categorization of the species of bacteria found in both organ and hemocultures of study animals (both moribund and surviving to termination) from all groups. n = 308.
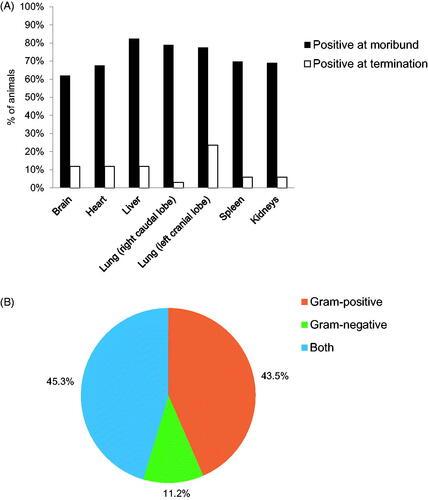
Table 2. Gram-positive and -negative bacteria identified in organ and hemocultures.
Results from this study suggest that the addition of azithromycin to the prophylactic supportive care, which included enrofloxacin given to all groups, had no significant effect on mitigating the incidences of sepsis and bacteremia in NHPs post-irradiation. This may be possibly due to preexisting resistance of the commensal flora to both enrofloxacin and azithromycin. To further investigate this phenomenon, we compiled antibiograms of the top five most common bacterial strains identified in organ and hemoculture against a panel of commonly used broad-spectrum antibiotics (). Based on the antibiogram, frequent resistance across the isolated bacterial strains was found against azithromycin, enrofloxacin, and gentamicin, which corroborated the inefficacy of enrofloxacin/azithromycin antibiotherapy observed in our study. Intermediate to low levels of resistance were present against four other antibiotics (amoxicillin/K clavulanate, cefepime, cefotaxime, ceftriaxone, and imipenem-cilastatin). Finally, there appeared to be minimal resistance among bacterial strains against ceftazidime, a broad-spectrum beta-lactam antibiotic.
Figure 6. Summary of the antibiogram of the top five most common bacterial strains identified in organ and hemocultures. The cumulative resistance of the identified bacterial strains against a panel of commonly used broad-spectrum antibiotics. Results are pooled from animals of all groups (n = 308), both moribund and surviving.
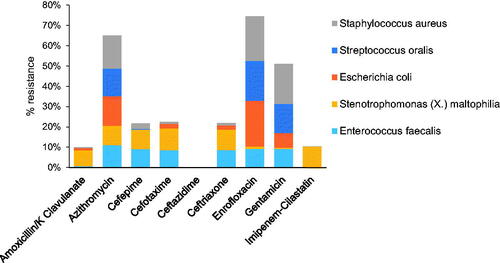
Discussion
The administration of LGFs such as G-CSF and GM-CSF has now been approved by the U.S. FDA as MCMs after a radiological and/or nuclear incident (US FDA Citation2018). While the administration of G-CSFs such as filgrastim and pegfilgrastim post-irradiation have been shown to improve survival and neutrophil recovery in NHP models if given with full supportive care, delays in treatment (i.e. exceeding 24 h post-irradiation) appeared to negatively affect the efficacy of these drugs (Farese et al. Citation2013; Hankey et al. Citation2015). We developed a nonclinical program to evaluate the effects of delayed sargramostim (rhu GM-CSF) administration in a NHP model of ARS under the criteria of the U.S. FDA ‘Animal Rule’ (US FDA Citation2019).
We showed that a delayed sargramostim regimen (i.e. started at 48 h post-radiation) enhanced survival of the animals post-irradiation (t-test, p = .0032). The magnitude of the increase in hematological parameters such as reticulocyte counts was also greater in all sargramostim-treated groups, which may have increased oxygenation and reduced oxidative cell death in the treated animals (Kuhn et al. Citation2017), thus potentially improving overall survival. A second, more moderate decline in reticulocyte count observed in all groups up to approximately Day 16 may have resulted from iron sequestration typically observed with acute inflammation (Ganz and Nemeth Citation2009).
Additionally, sargramostim treatment at 48, 72, 96, and 120 h post-irradiation significantly improved neutrophil, lymphocyte, and platelet recovery in the NHP ARS model. Animals treated with sargramostim 48 h after irradiation had earlier recoveries and significantly shorter mean durations of neutropenia (ANC < 500/μL) compared to untreated controls (10.1 days compared to 13.3 days, p ≤ .001). Interestingly, G-CSFs such as filgrastim and pegfilgrastim have not been previously shown to effectively increase survival when treatment is delayed – although the administration of filgrastim shortened the duration of neutropenia and time to recovery of neutrophils in NHPs after TBI, it did not induce a significant increase in survival compared to controls when given at 48 h post irradiation (Farese et al. Citation2014), and pegfilgrastim has only been studied starting at 24 h post irradiation (Hankey et al. Citation2015). In the event of a large nuclear or radiation emergency, prompt dispersal of time-sensitive therapeutics (i.e. treatment within 24 h of incident) such as G-CSFs may present logistical challenges. As such, MCMs that are efficacious with delayed administration may be advantageous in such a scenario.
This disparity in the observed survival efficacy of delayed treatments of G-CSF and GM-CSF may be due to the different downstream effects induced by each LGF. GM-CSF, such as sargramostim, has the ability to stimulate multiple cell lineages from progenitors, including megakaryocytes which are responsible for platelet production, as well as erythrocytes and a host of immune cells including monocytes and neutrophils (Ushach and Zlotnik Citation2016). G-CSFs, however, are considered to be more selective for the enhancement of neutrophil production and function (Basu et al. Citation2002), and have been previously proposed to actually suppress the maturation and development of megakaryocytes in murine models (Saito et al. Citation1996; Metcalf et al. Citation2005). The implications of these divergent functions of GM-CSF and G-CSF can be seen in NHP ARS studies, where all but one surviving animals who received filgrastim (rhu G-CSF) required anywhere from 0.5 to 5.5 blood transfusions during the study (Farese et al. Citation2013). In a separate study, filgrastim treatment at 24 h post irradiation without transfusions and additional support resulted in no significant survival benefits compared to controls (31–36%, respectively) (Gluzman-Poltorak et al. Citation2014). Based on these results, blood transfusions may be important with G-CSF therapies post-irradiation. In contrast, the current study along with similar, previously unpublished data suggests that the beneficial effects of sargramostim (rhu GM-CSF) on survival did not require transfusions, which may also translate well into clinical settings.
As well, the effects of G-CSF on other measures of bone marrow function and immune reconstitution in NHPs have not been reported. However, GM-CSF is shown in our study to improve multiple parameters, of which lymphocyte counts appeared to be very well correlated with mortality following radiation exposure. The effect of GM-CSF on lymphocytes was observed in prior studies and was an unexpected finding since GM-CSF is thought to affect predominantly myeloid lineage. However, the replication of this finding in this study suggests this effect is real and may contribute to the different outcomes observed. As such, further understanding of the relationship between hematological changes and the risk of infection and mortality may help to elucidate both the appropriate assessment and management of patients exposed to lethal doses of radiation.
Decline in neutrophil and lymphocyte counts after radiation can lead to immunosuppression and can result in the systemic dissemination of bacterial infections, leading to sepsis which can contribute to mortalities (Brook et al. Citation1988; Kumar et al. Citation2002; Dainiak et al. Citation2003). Sargramostim treatment at 48 h post-irradiation significantly reduced the incidence of sepsis (, p ≤ .01). This effect could result from the faster ANC recovery and/or the higher leukocyte count at nadir observed in sargramostim-treated animals compared to reference item/vehicle 48 h-treated animals. Additionally, prophylactic treatments with broad-spectrum antibiotic have been suggested to also reduce the risk of infection due to immunosuppression after irradiation and has previous been shown to improve survival in neutropenic patients undergoing cytotoxic therapy for cancer (Gafter-Gvili et al. Citation2005). However, prophylactic administration of azithromycin and enrofloxacin in combination or either agent alone did not reduce incidence of morality and sepsis in our model, possibly due to high frequencies of resistant infections (). Additionally, as the LD values were above 80 (LD83/60) in the reference item/vehicle 48 h with or without azithromycin control groups (Groups 1 and 6), it is difficult to linearize the responses and claim a survival advantage to accurately assess the true effects of azithromycin administration on survival.
Further investigation revealed that Staphylococcus aureus, Streptococcus oralis, Escherichia coli, Stenotrophomonas (X.) maltophilia, and Enterococcus faecalis were the most commonly isolated bacterial strains in the organ cultures of all animals in the study. Most of these strains are commonly found in the microbiota of both wild and laboratory NHPs, which suggests that they are likely opportunistic infections resulting from immunosuppression post-irradiation (van den Berg et al. Citation2011; Clayton et al. Citation2014; Lebreton et al. Citation2014; Denapaite et al. Citation2016). All five strains have also been previously identified as commensal strains in the human mucosal microbiota as well (Hamada and Slade Citation1980; Conway et al. Citation2004; Mason et al. Citation2011; Adegoke et al. Citation2017; Young et al. Citation2017). Antibiograms obtained from these five strains against nine different antibiotics showed wide spread resistance to azithromycin and enrofloxacin among all strains, which likely accounts for the ineffectiveness of azithromycin treatment added to the prophylactic administration of enrofloxacin. Most isolated strains were also resistant against gentamicin and showed mid to low levels of resistance against a variety of other antibiotics such as cephalosporin and penicillin. Remarkably, there appeared to be little resistance to ceftazidime (a beta-lactam antibiotic), suggesting that prophylactic treatments with ceftazidime may be more effective at preventing opportunistic infections in this model that included enrofloxacin prophylactically in all groups.
In conclusion, delayed sargramostim treatment after irradiation significantly improved hematological parameters, as well as decreased the incidences of sepsis in an NHP model of ARS. Sargramostim appeared to present beneficial hematological effects, but limited improvements on survival, when administered at a delayed time of up to 120 h post irradiation with minimal supportive care, which would extend the time-of-treatment window of efficacy post-irradiation and may yield deployment advantages after a nuclear incident where resources are likely to be limited and delayed. The addition of prophylactic azithromycin treatment did not appear to have beneficial effects in the presence of enrofloxacin, as high frequency of resistance to both enrofloxacin and azithromycin may have limited their efficacy as a component of supportive care. Nevertheless, antibiotherapy is still expected to be a pivotal component of the countermeasure strategy – with the caveat that careful selection of the appropriate antibiotherapy is likely needed to circumvent resistance. Overall, sargramostim can be considered as an effective treatment for ARS with survival or hematological benefits observed up to 120 h post-exposure.
Acknowledgements
The authors would like to thank the staff at BARDA (Biomedical Advanced Research and Development Authority, DC, USA) for their contribution to the design and analysis of the study.
Disclosure statement
None of the authors have any conflicts of interest, other than their employment in commercial pharmaceutical companies or contract research organizations.
Additional information
Funding
Notes on contributors
Yifei Zhong
Yifei Zhong is a study director at Citoxlab, a Charles River company. She holds a B.Sc. in Microbiology and Immunology and a HBA from the University of Western Ontario, and a Ph.D. in Pharmacology and Therapeutics from McGill University.
Mylene Pouliot
Mylene Pouliot is a study director at Citoxlab North America. She holds a B.Sc. and a Ph.D. in Physiology from Universite de Montreal and is a Diplomate of Safety Pharmacology (DSP).
Anne-Marie Downey
Anne-Marie Downey is a research fellow and study director at Citoxlab, a Charles River company. She holds a B.Sc. in Biopharmaceutical Sciences (medicinal chemistry) from the University of Ottawa and a Ph.D. in Pharmacology and Therapeutics from McGill University.
Colleen Mockbee
Colleen Mockbee is the chief development officer and head of regulatory and quality at Partner Therapeutics. She graduated from Butler University with degrees in chemistry and pharmacy in 1990 and 1994, and received her MBA from Purdue’s Krannert School of Business in 2016.
Debasish Roychowdhury
Debasish Roychowdhury is a hematologist/oncologist and the co-founder of Partner Therapeutics and serves as its chief medical officer. He graduated from India and competed his fellowship from UCSF and practiced in Cincinnati before joining the pharmaceutical industry. He served in various leadership roles in Lilly, GSK and Sanofi and since 2013 has been a serial enterpreneur.
Wieslaw Wierzbicki
Wieslaw Wierzbicki is a certified radiophysicist with a Ph.D. in biophysics from Universite de Montreal and a post-doctoral fellowship from McGill University.
Simon Authier
Simon Authier is senior director of scientific operations and veterinary science at Citoxlab, a Charles River company. He holds a DVM from Universite de Montreal, an MBA from HEC and a Ph.D. in Pharmacology. He is a Diplomate of Safety Pharmacology (DSP).
References
- Adegoke AA, Stenstrom TA, Okoh AI. 2017. Stenotrophomonas maltophilia as an emerging ubiquitous pathogen: looking beyond contemporary antibiotic therapy. Front Microbiol. 8:2276.
- Amgen Inc. 2018. Neupogen (filgrastim) – US prescribing information. [accessed 2019 Oct 2]. https://www.pi.amgen.com/∼/media/amgen/repositorysites/pi-amgen-com/neupogen/neupogen_pi_hcp_english.pdf
- Amgen Inc. 2019. Neulasta (pegfilgrastim) – US prescribing information. [accessed 2019 Oct 2]. https://www.pi.amgen.com/∼/media/amgen/repositorysites/pi-amgen-com/neulasta/neulasta_pi_hcp_english.pdf
- Basu S, Hodgson G, Katz M, Dunn AR. 2002. Evaluation of role of G-CSF in the production, survival, and release of neutrophils from bone marrow into circulation. Blood 100(3):854–861.
- Booth C, Tudor G, Tudor J, Katz BP, MacVittie TJ. 2012. Acute gastrointestinal syndrome in high-dose irradiated mice. Health Phys. 103(4):383–399.
- Brook I, Walker RI, MacVittie TJ. 1988. Effect of antimicrobial therapy on bowel flora and bacterial infection in irradiated mice. Int J Radiat Biol Relat Stud Phys Chem Med. 53(5):709–716.
- Clayton J, Danzeisen J, Trent A, Murphy T, Johnson T. 2014. Longitudinal characterization of Escherichia coli in healthy captive non-human primates. Front Vet Sci. 1:24.
- Clayton NP, Charpentier E, LaCasse ER, Khan-Malek RC, Keutzer JM. 2016. Sargramostim accelerates leukocyte recovery and improves mortality rate at day 60 in a non-human primate model of hematopoietic acute radiation syndrome when administered 48 h after total body irradiation. Blood 128:2512.
- Conway T, Krogfelt KA, Cohen PS. 2004. The life of commensal Escherichia coli in the mammalian intestine. EcoSal Plus. 1(1). https://doi.org/https://doi.org/10.1128/ecosalplus.8.3.1.2
- Dainiak N. 2002. Hematologic consequences of exposure to ionizing radiation. Exp Hematol. 30(6):513–528.
- Dainiak N, Waselenko JK, Armitage JO, MacVittie TJ, Farese AM. 2003. The hematologist and radiation casualties. Hematology Am Soc Hematol Educ Program. 2003(1):473–496.
- Denapaite D, Rieger M, Kondgen S, Bruckner R, Ochigava I, Kappeler P, Matz-Rensing K, Leendertz F, Hakenbeck R. 2016. Highly variable Streptococcus oralis strains are common among Viridans Streptococci isolated from primates. mSphere 1(2):pii: e00041-15.
- DiCarlo AL, Maher C, Hick JL, Hanfling D, Dainiak N, Chao N, Bader JL, Coleman CN, Weinstock DM. 2011. Radiation injury after a nuclear detonation: medical consequences and the need for scarce resources allocation. Disaster Med Public Health Prep. 5(S1):S32–S44.
- Dorr H, Meineke V. 2011. Acute radiation syndrome caused by accidental radiation exposure – therapeutic principles. BMC Med. 9:126.
- Farese AM, Brown CR, Smith CP, Gibbs AM, Katz BP, Johnson CS, Prado KL, MacVittie TJ. 2014. The ability of filgrastim to mitigate mortality following LD50/60 total-body irradiation is administration time-dependent. Health Phys. 106(1):39–47.
- Farese AM, Cohen MV, Katz BP, Smith CP, Gibbs A, Cohen DM, MacVittie TJ. 2013. Filgrastim improves survival in lethally irradiated nonhuman primates. Radiat Res. 179(1):89–100.
- Farese AM, Cohen MV, Stead RB, Jackson W, MacVittie TJ. 2012. Pegfilgrastim administered in an abbreviated schedule, significantly improved neutrophil recovery after high-dose radiation-induced myelosuppression in rhesus macaques. Radiat Res. 178(5):403–413.
- Farese AM, Williams DE, Seiler FR, MacVittie TJ. 1993. Combination protocols of cytokine therapy with interleukin-3 and granulocyte-macrophage colony-stimulating factor in a primate model of radiation-induced marrow aplasia. Blood 82(10):3012–3018.
- Gafter-Gvili A, Fraser A, Paul M, Leibovici L. 2005. Meta-analysis: antibiotic prophylaxis reduces mortality in neutropenic patients. Ann Intern Med. 142(12_Part_1):979–995.
- Ganz T, Nemeth E. 2009. Iron sequestration and anemia of inflammation. Semin Hematol. 46(4):387–393.
- Gianni AM, Bregni M, Siena S, Villa S, Sciorelli GA, Ravagnani F, Pellegris G, Bonadonna G. 1989. Rapid and complete hemopoietic reconstitution following combined transplantation of autologous blood and bone marrow cells. A changing role for high dose chemo-radiotherapy? Hematol Oncol. 7(2):139–148.
- Gluzman-Poltorak Z, Vainstein V, Basile LA. 2014. Recombinant interleukin-12, but not granulocyte-colony stimulating factor, improves survival in lethally irradiated nonhuman primates in the absence of supportive care: evidence for the development of a frontline radiation medical countermeasure. Am J Hematol. 89(9):868–873.
- Goans RE, Holloway EC, Berger ME, Ricks RC. 1997. Early dose assessment following severe radiation accidents. Health Phys. 72(4):513–518.
- Hamada S, Slade HD. 1980. Biology, immunology, and cariogenicity of Streptococcus mutans. Microbiol Rev. 44(2):331–384.
- Hankey KG, Farese AM, Blaauw EC, Gibbs AM, Smith CP, Katz BP, Tong Y, Prado KL, MacVittie TJ. 2015. Pegfilgrastim improves survival of lethally irradiated nonhuman primates. Radiat Res. 183(6):643–655.
- Inoue T, Hirabayashi Y, Mitsui H, Sasaki H, Cronkite EP, Bullis JE, Jr., Bond VP, Yoshida K. 1995. Survival of spleen colony-forming units (CFU-S) of irradiated bone marrow cells in mice: evidence for the existence of a radioresistant subfraction. Exp Hematol. 23(12):1296–1300.
- Kuhn V, Diederich L, Keller TCS, Kramer CM, Lückstädt W, Panknin C, Suvorava T, Isakson BE, Kelm M, Cortese-Krott MM. 2017. Red blood cell function and dysfunction: redox regulation, nitric oxide metabolism, anemia. Antioxid Redox Signal. 26(13):718–742.
- Kumar KS, Srinivasan V, Toles RE, Miner VL, Jackson WE, Seed TM. 2002. High-dose antibiotic therapy is superior to a 3-drug combination of prostanoids and lipid A derivative in protecting irradiated canines. J Radiat Res. 43(4):361–370.
- Laterveer L, Zijlmans JM, Liehl E, Willemze R, Fibbe WE. 1996. Accelerated platelet reconstitution following transplantation of bone marrow cells derived from IL-6-treated donor mice. Ann Hematol. 73(5):239–245.
- Lebreton F, Willems RJL, Gilmore MS. 2014. Enterococcus diversity, origins in nature, and gut colonization. In: Gilmore MS, Clewell DB, Ike Y, editors. Enterococci: from commensals to leading causes of drug resistant infection. Boston: Massachusetts Eye and Ear Infirmary.
- MacVittie TJ, Bennett AW, Farese AM, Taylor-Howell C, Smith CP, Gibbs AM, Prado K, Jackson W. 3rd. 2015. The effect of radiation dose and variation in neupogen(R) initiation schedule on the mitigation of myelosuppression during the concomitant GI-ARS and H-ARS in a nonhuman primate model of high-dose exposure with marrow sparing. Health Phys. 109(5):427–439.
- Mason KL, Stepien TA, Blum JE, Holt JF, Labbe NH, Rush JS, Raffa KF, Handelsman J. 2011. From commensal to pathogen: translocation of Enterococcus faecalis from the midgut to the hemocoel of Manduca sexta. MBio 2(3):e00065–00011.
- Mehta HM, Malandra M, Corey SJ. 2015. G-CSF and GM-CSF in neutropenia. J Immunol. 195(4):1341–1349.
- Metcalf D, Mifsud S, Di Rago L. 2005. Murine megakaryocyte progenitor cells and their susceptibility to suppression by G-CSF. Stem Cells 23(1):55–62.
- Plett PA, Sampson CH, Chua HL, Joshi M, Booth C, Gough A, Johnson CS, Katz BP, Farese AM, Parker J, et al. 2012. Establishing a murine model of the hematopoietic syndrome of the acute radiation syndrome. Health Phys. 103(4):343–355.
- Saito M, Takada K, Yamada T, Fujimoto J. 1996. Overexpression of granulocyte colony-stimulating factor in vivo decreases the level of polyploidization of mouse bone marrow megakaryocytes. Stem Cells 14(1):124–131.
- Sanofi-Aventis US LLC. 2018. Leukine (sargramostim) – US prescribing information. [accessed 2019 May 3]. https://www.accessdata.fda.gov/drugsatfda_docs/label/2017/103362s5237lbl.pdf
- Shrestha RP, Horowitz J, Hollot CV, Germain MJ, Widness JA, Mock DM, Veng-Pedersen P, Chait Y. 2016. Models for the red blood cell lifespan. J Pharmacokinet Pharmacodyn. 43(3):259–274.
- Singh VK, Newman VL, Seed TM. 2015. Colony-stimulating factors for the treatment of the hematopoietic component of the acute radiation syndrome (H-ARS): a review. Cytokine 71(1):22–37.
- Singh VK, Seed TM. 2017. A review of radiation countermeasures focusing on injury-specific medicinals and regulatory approval status: part I. Radiation sub-syndromes, animal models and FDA-approved countermeasures. Int J Radiat Biol. 93(9):851–869.
- Smith TJ, Khatcheressian J, Lyman GH, Ozer H, Armitage JO, Balducci L, Bennett CL, Cantor SB, Crawford J, Cross SJ, et al. 2006. 2006 update of recommendations for the use of white blood cell growth factors: an evidence-based clinical practice guideline. J Clin Oncol 24(19):3187–3205.
- US FDA. 2018. Radiological and nuclear emergency preparedness information from FDA. https://www.fda.gov/emergency-preparedness-and-response/mcm-issues/radiological-and-nuclear-emergency-preparedness-information-fda.
- US FDA. 2019. Animal rule information. https://www.fda.gov/emergency-preparedness-and-response/mcm-regulatory-science/animal-rule-information.
- Ushach I, Zlotnik A. 2016. Biological role of granulocyte macrophage colony-stimulating factor (GM-CSF) and macrophage colony-stimulating factor (M-CSF) on cells of the myeloid lineage. J Leukoc Biol. 100(3):481–489.
- van den Berg S, van Wamel WJ, Snijders SV, Ouwerling B, de Vogel CP, Boelens HA, Willems RJ, Huijsdens XW, Verreck FA, Kondova I, et al. 2011. Rhesus macaques (Macaca mulatta) are natural hosts of specific Staphylococcus aureus lineages. PLoS One 6(10):e26170.
- Waller EK. 2007. The role of sargramostim (rhGM-CSF) as immunotherapy. Oncologist. 12(Suppl. 2):22–26.
- Waselenko JK, MacVittie TJ, Blakely WF, Pesik N, Wiley AL, Dickerson WE, Tsu H, Confer DL, Coleman CN, Seed T, et al. 2004. Medical management of the acute radiation syndrome: recommendations of the Strategic National Stockpile Radiation Working Group. Ann Intern Med. 140(12):1037–1051.
- Weinstock DM, Case C, Jr., Bader JL, Chao NJ, Coleman CN, Hatchett RJ, Weisdorf DJ, Confer DL. 2008. Radiologic and nuclear events: contingency planning for hematologists/oncologists. Blood 111(12):5440–5445.
- Young BC, Wu CH, Gordon NC, Cole K, Price JR, Liu E, Sheppard AE, Perera S, Charlesworth J, Golubchik T, et al. 2017. Severe infections emerge from commensal bacteria by adaptive evolution. Elife 6:e30637.