Abstract
Purpose
Previous research has highlighted the impact of radiation damage, with cancer patients developing acute disorders including radiation induced pneumonitis or chronic disorders including pulmonary fibrosis months after radiation therapy ends. We sought to discover biomarkers that predict these injuries and develop treatments that mitigate this damage and improve quality of life.
Materials and methods
Six- to eight-week-old female C57BL/6 mice received 1, 2, 4, 8, 12 Gy or sham whole body irradiation. Animals were euthanized 48 h post exposure and lungs removed, snap frozen and underwent RNA isolation. Microarray analysis was performed to determine dysregulation of messenger RNA (mRNA), microRNA (miRNA), and long non-coding RNA (lncRNA) after radiation injury.
Results
We observed sustained dysregulation of specific RNA markers including: mRNAs, lncRNAs, and miRNAs across all doses. We also identified significantly upregulated genes that can indicate high dose exposure, including Cpt1c, Pdk4, Gdf15, and Eda2r, which are markers of senescence and fibrosis. Only three miRNAs were significantly dysregulated across all radiation doses: miRNA-142-3p and miRNA-142-5p were downregulated and miRNA-34a-5p was upregulated. IPA analysis predicted inhibition of several molecular pathways with increasing doses of radiation, including: T cell development, Quantity of leukocytes, Quantity of lymphocytes, and Cell viability.
Conclusions
These RNA biomarkers might be highly relevant in the development of treatments and in predicting normal tissue injury in patients undergoing radiation treatment. We are conducting further experiments in our laboratory, which includes a human lung-on-a-chip model, to develop a decision tree model using RNA biomarkers.
Introduction
Discovering early biomarkers for radiation injury of normal tissue is necessary for protecting both cancer patients receiving radiotherapy and civilians who may be accidentally or intentionally exposed to a nuclear event. Radiation injury of lung tissue occurs within seconds of exposure through direct damage of DNA or through the production of reactive oxygen species (ROS). Thoracic radiation in murine models can result in incomplete repair of cellular DNA and organelles that can compromise organ integrity and produce cell death, senescence and inflammation. These changes have been observed in lung tissue several weeks after radiation injury (Malaviya et al. Citation2015; Giuranno et al. Citation2019).
The respiratory system consists of a large surface area continuously exposed to viruses and bacteria (Kato et al. Citation2013). Higher glucose concentration along the airway surface can increase bacterial growth and infection; this concentration is impacted by the permeability of tight junctions, and inflammation which is known to be increased by radiation injury (Baker and Baines Citation2018). The risk of death from bacterial pneumonia and other diseases is elevated in cancer patients both due to the disease and the impact of treatment (Wong and Evans Citation2017; Mei et al. Citation2022). To protect the lung, T cells and B cells are present in the lung parenchyma. Radiation can cause both lymphocyte cell death and lymphocyte activation which causes an inflammatory cascade that leads to radiation pneumonitis if unchecked (Wirsdörfer and Jendrossek Citation2016). Further, radiation of lung tissue produces structural damage which may include endothelial and epithelial death, disruption of microvasculature and fibrosis (Jain and Berman Citation2018; Giuranno et al. Citation2019). Endothelial damage includes both vascular endothelial cell death leading to decreased gas exchange at alveoli and lymphatic endothelial cell death leading to failure to transport antigens and antigen-presenting cells to lymph nodes with subsequent buildup in the lungs (Stump et al. Citation2017). Death of lymphatic endothelial cells and decreased lymphatic vessels have previously been linked to the development of lung fibrosis (Cui et al. Citation2014). This damage occurs shortly after radiation injury and can lead to chronic disability such as decreased lung function, hypoxemia, and, ultimately, early death (Torre-Bouscoulet et al. Citation2018).
Radiation therapy is commonly used to treat cancer patients. However, the usefulness of radiation therapy to the thorax for lung cancer, breast cancer, lymphoma or esophageal cancer is limited by normal tissue damage (Pinnix et al. Citation2015; Hanania et al. Citation2019). Radiation-induced activation of an inflammatory response acts as a double-edged sword, improving the antitumor impact of radiation while also impairing the quality of life by increasing the destruction of normal tissue (Shaverdian et al. Citation2016).
Radiation biomarkers could serve a twofold purpose. These markers would aid in triaging of civilians after a nuclear event, allowing separation of the ‘concerned citizens’ from those needing treatment. They could also aid in therapeutic decision-making for cancer patients, enabling doctors to offer treatment for radiation side effects to patients prior to developing symptoms. Several biomarker assays for whole body dosimetry exist, including the dicentric chromosome assay, γ-H2AX assay, the cytokinesis block micronucleus assay, and gene profiling. The strengths and weaknesses of these assays have previously been discussed (Hall et al. Citation2017). Recent evidence has reinforced the potential for RNA to be used as biomarkers of radiation (Kultova et al. Citation2020; Małachowska et al. Citation2020).
Prior research has highlighted the role of both coding and non-coding RNA in radiation injury (Macaeva et al. Citation2016; Singh and Pollard Citation2017; May et al. Citation2021). The noncoding RNA of interest include microRNA (miRNA) which are small non-coding nucleotides (19–22), and long non-coding RNA (lncRNA) which are over 200 nucleotides in length. Both are known to regulate protein-coding through interaction with mRNA or each other (Wang et al. Citation2019; Jakubik et al. Citation2021). These interactions can impact diverse functions within the cell including disease pathology, metabolism, radiation resistance and cell proliferation (Volovat et al. Citation2020; Jakubik et al. Citation2021). Many miRNA are conserved across species and are known to be stable both within the body and after blood draw of patients, making it a good potential biomarker (Acharya et al. Citation2015; Tomasik et al. Citation2018; Mori et al. Citation2019). Similarly, lncRNA from human serum could differentiate patients with bladder cancer compared to healthy control volunteers, indicating the stability of lncRNA in serum (Zhang et al. Citation2019).
This study used multiple doses of radiation at an early time point (48 h post-radiation) to determine early RNA biomarkers. This information in conjunction with previously published long-term literature, may be useful in understanding pathway dysfunction after different doses of radiation exposure. We employed a whole- transcriptome approach to discover radiation-induced biomarkers in normal mouse lung tissue. A thorough understanding of gene alterations in cell pathways may provide information on the appropriate methods for the mitigation of normal tissue damage. It is necessary to further develop strategies that mitigate radiation damage to normal lung tissue through early detection of injury.
Methods
Whole body irradiation of mice and sample collection
Six- to eight-week-old female C57BL/6 mice were given whole-body irradiation (WBI) with x-rays using the Small Animal Radiation Research Platform (SARRP Xstrahl Ltd.). Mice were placed in plastic containers and exposed to a single surface dose of 1, 2, 4, 8, or 12 Gy at a dose rate of 1.05 Gy/min. SARRP machinery was calibrated using SARRP software 3.3.6 which includes Rob2CT Transformation and Beam Calibration. Irradiator was checked for correct calibration and preventative maintenance was performed within 2 months prior to experiment start. X-ray tube potential was 225 kV and inherent Filtration: 0.8 mm ± 0.1 mm Be (Beryllium). Control mice (0 Gy) were placed in the same type of plastic container and sham irradiated. Three animals per dose were included in the study. Lungs of irradiated and control animals were harvested 48 hours after WBI. Organs were snap-frozen in liquid nitrogen and stored at −80 °C until processed for RNA isolation, no perfusion was performed for either C57BL/6 or C3H/HeJ. All animal experiments were performed at the Department of Pathology at New York University (NYU) Langone Medical Center under an approved IACUC protocol as part of a collaborative study. All animal experiments were approved by the animal welfare committee, the Institutional Animal Care and Use Committees (IACUC) of the New York University School of Medicine.
C3H/HeJ tissue collection validation studies was performed at the National Cancer Institute, Radiation Oncology branch. C3H/HeJ mice (8–14 weeks of age) were irradiated with a Precision X-ray source at a dose rate of 2.28 Gy/min. All mice used in this study were of the same age and commensurate weight. The dose rate was calibrated based upon the procedures described in the American Association of Physicist in Medicine (AAPM) Task Group Report 61 (TG-61) with regard to the following conditions: X-ray tube potential was 300 kV, half value layer (HVL) is 0.8 mm Copper (Cu), source to surface distance (SSD) was 50 cm. The dose rate was measured at 2 cm depth in a solid water phantom using a PTW model: N23342 ion chamber and Inovision, model 35,040 electrometer. This research was conducted in accordance with the principles and procedures outlined in the NIH Guide for the Care and Use of Animals and procedures. These methods are reported in accordance with ARRIVE guidelines (https://arriveguidelines.org).
RNA isolation
Samples were bathed in liquid nitrogen and pulverized into a fine powder using a mortar and pestle. Approximately 100 µg of powdered sample was lysed with 700 µl of QIAzol lysis buffer (Cat # 79306, QIAGEN) and homogenized by passing the solution through QIAshredder spin columns (Cat # 79654, QIAGEN). RNA isolation was performed using a standard miRNeasy mini kit (Cat # 217004, QIAGEN) according to the manufacturer’s protocol. The quality and quantity of the RNA samples were assessed using a DeNovix DS-11 nanodrop spectrophotometer (DeNovix, DE, US) and Agilent Bioanalyzer with the RNA6000 Nano Lab Chip (Agilent Technologies, Santa Clara, CA).
Microarray analysis
Microarray analysis was performed for sham animals (0 Gy) and 1 Gy, 2 Gy, 4 Gy, 8 Gy, and 12 Gy irradiated animals. Only C57BL/6 mice were analyzed by microarray. Quality assessments and microarray experiments were completed as previously reported (Aryankalayil, Chopra, Makinde, et al. Citation2018). Samples were hybridized to Agilent Mouse GE 8x60K v2 arrays for mRNA expression analysis and to Agilent Mouse miRNA 8x60K v21.0 arrays (Design ID 070155) for miRNA expression analysis. Slides were washed and scanned on an Agilent SureScan Microarray Scanner. Expression values were extracted using Agilent Feature Extraction software.
Real time RT-PCR analysis of RNAs
Individual qRT-PCR reactions using RT2 qPCR primer assays along with RT2 First Strand Synthesis kit and RT2 SYBR Green qPCR Master Mix (QIAGEN) were performed. The following RNA primers were purchased from Qiagen, gene globe IDs are included for mRNA and assay IDs for non-coding RNA: Cdkn1a (PPM02901B-200), Eda2r (PPM32677A-200), Phlda3 (PPM28194A-200), Hba-a2 (PPM69448A-200), miR-34a (YP00204486), miR-142-3p (YP00204291), miR-142-5p (YP00204722) Trp53cor1 (LPM12776A), Dino (Schmitt et al. Citation2016)(FP-GCAATGGTGTGCCTGACTAT; RP- TTCTGGCTTCCCAGAG), and Rplp0 (PPM03561B). QRT-PCR analysis was performed on select miRNA, lncRNA, and mRNA to validate results and determine cross-strain accuracy as C3H/HeJ mouse liver lung was also tested for RNA expression. C3H/HeJ organ RNA extraction and qRT-PCR analysis were the same as explained above. Relative expression was calculated as 2−dCt where dCt = Ct [test gene] − Ct Rplp0 (Aryankalayil et al. Citation2018).
Statistical analysis
Analysis of mRNA and miRNA data was performed using R statistical software and the Bioconductor Linear Model for Microarray Analysis (LIMMA) package in R (Ritchie et al. Citation2015). Background correction and normalization were performed in R using the normal-exponential correction method and quantile normalization between arrays (Ritchie et al. Citation2007). Only probes with intensities above background on at least one array were kept in the dataset for analysis. Transcripts with multiple probes were averaged such that the final set reflected the best estimates of transcript-level expression. A linear model was fit to each probe to assess differential expression for pair-wise dose comparisons within the lung-tissue samples. This method employed an empirical Bayes smoothing approach that results in more stable model estimates by using the information on variance from the whole probe set, despite the small number of arrays. Models were developed for each of the pair-wise comparisons between each dose (1, 2, 4, 8, and 12 Gy) and the control probes (0 Gy), and resulting probes were filtered using log2 fold change and adjusted p-value thresholds (|log2FC| > 1, adjusted p-value <.05) (Smyth Citation2004). Additionally, a nested interaction model was fit for each probe to examine dose within tissue as a linear (continuous) trend. Each model yielded main effects for the lung tissue and dose within the lung tissue. Probes were filtered using the nested dose coefficients with log fold change and adjusted p-value thresholds (|log2FC| > 1, adjusted p-value < .05). The color spectrum on each plot is the log2fold change between the dose group and the 0 Gy control group.
To identify potential interactions, paired analysis was conducted to evaluate correlative relationships between pairs of differentially expressed mRNA and miRNA probes. mRNA and miRNA probes were paired using shared target transcript Ensembl IDs (Aken et al. Citation2016). Probes that could not be mapped or paired were excluded. Transcripts for miRNA probes were identified using an Agilent microarray gene dataset and the TargetScan database; transcripts for mRNA probes were identified using an Agilent microarray gene dataset (Agarwal et al. Citation2015). Transcript-miRNA pairs with a TargetScan context++ score above −1 were excluded. Probe pairs with differentially expressed miRNA and mRNA probes were identified within the lung tissue for continuous dose contrast models. Pearson correlation coefficients of miRNA and mRNA expression across all experiments were calculated and plotted for the differentially expressed probe pairs.
Ingenuity pathway analysis
Both core and comparison analyses were performed in IPA (QIAGEN Inc., https://www.qiagenbioinformatics.com/products/ingenuitypathway-analysis). Pathways and function terms that satisfied [log2 fold change (FC)] > 1 and Benajmini-Hochberg adjusted (B-H) p-value < .05 were predicted to be altered based on the gene expression data.
Results
Increasing doses of radiation produced increased transcriptional dysregulation
Microarray analysis of C57BL/6 mouse lung samples indicated that 3688 probes were dysregulated (|log2FC| > 1; p-value <.05) in at least one dose of WBI mice compared to unirradiated mice. Overall, these probes showed a general downregulation compared to controls (). Across all doses, 49 probes were expressed, while 12, 5, 71, 119, and 1800 probes were exclusively expressed at 1, 2, 4, 8, and 12 Gy, respectively (). No genes were shared at only low dose (1 and 2 Gy) while 374 genes were shared only between high doses (8 and 12 Gy). Fold changes and p-values of all differentially expressed genes by dose are presented (Supplemental Table S1). To determine the greatest continuous dysregulation of genes across all doses we analyzed each gene by fitting a probe to a linear model. The most significantly upregulated genes were Gdf15, Celf5, and Eda2r (), while the most significantly downregulated genes were Hbb-b1, Alas2, and Zdhhc22. Genes relevant to mitochondrial function, oxygen transport and hemoglobin synthesis were downregulated, and genes relevant to cell cycle arrest and apoptosis were upregulated () (Sándor et al. Citation2015; Himburg et al. Citation2016; Li et al. Citation2017; Chen et al. Citation2019; Nie et al. Citation2019; Peng et al. Citation2021; Pervin et al. Citation2021). Supplemental Table S2 presents fold change and p-value for the top 20 most highly induced and 20 most highly repressed genes with a linear trend.qRT-PCR validation of mRNA and nc-RNA are provided in C57BL/6 and C3H/HeJ mouse strains after 1, 2, 4, and 8 Gy radiation treatments at 48 h (Supplemental Figure 1 (mRNA) and Supplemental Figure 2 (ncRNA). Cdkn1a showed significant upregulation at both low and high doses in C3H/HeJ mice but only at 8 and 12 Gy in C57BL/6 (Supplemental FIgure 1). Both Phlda3 and Eda2r were significantly increased for C3H/HeJ and C57BL/6 at 8 and 12 Gy. In contrast, Hba-a2 was downregulated for C3H/HeJ and C57BL/6 at 2, 4, 8, and 12 Gy. For non-coding RNA, miR-142-5p and miR-34a both showed significant expression changes at higher doses. Across strains, both Trp53cor1 and Dino showed similar upregulation starting from 2 Gy for Trp53cor1, and 4 Gy for Dino.
Figure 1. Radiation-induced gene expression profiles in C57BL/6 mouse lung tissue. Whole genome microarray analysis was performed on all samples. The heatmaps were created by calculating the log2 fold change between the mean of each dose group compared to the 0 Gy control group. There was no normalization done per row. The color spectrum on each plot is the log2fold change between the dose group and the 0 Gy control group. Criteria of |log2 fold change (FC)| > 1 and Benajmini-Hochberg adjusted (B-H) p-value < .05 relative to controls were used to determine significance and differential expression. (A) Heatmap displays expression patterns, represented by z-score, of top 40 most differentially expressed mRNAs across all doses and controls. Red indicates upregulation, blue indicates down regulation. (B) The number of down-regulated versus up-regulated mRNAs at each dose are shown in the table. (C) Venn diagram shows dose distribution and overlap of differentially expressed mRNAs across all doses. (D) Examples of significant linearly up- and down-regulated mRNAs are shown to display the dose-response to radiation in lung tissue samples. Asterisk (*) indicates statistical significance.
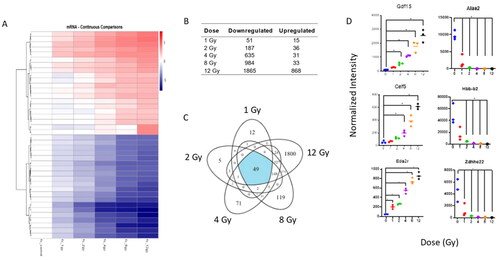
Table 1. Biological roles of most significantly dose-responsive mRNAs.
Pathways relevant to immune response and cell survival were dysregulated
Canonical pathway analysis and function analysis using differentially expressed mRNA was performed using IPA with significant deactivation (|log2FC| > 1; p-value <.05) of most pathways ( (canonical pathways) 2B (function)). While immune response pathways were significantly decreased in the lung after radiation, including the Th1 pathway (), we chose to curate our list to display other pathways of interest. Notably, pathways relevant to cell adhesion including the FAK pathway and pathways relevant to glucose metabolism, Diabetes Mellitis pathway were significantly downregulated starting at 2 Gy. The senescence pathway was upregulated between 2-8 Gy but was downregulated at 12 Gy. PI3K/AKT signaling showed downregulation from 4-12 Gy. Within the functional pathways, gene dysregulation indicated decreases of Lymphopoiesis and Quantity of leukocytes starting at 1 Gy. Cell viability and Cell survival showed decreased expression starting at 2 Gy ().
Figure 2. Predicted pathway dysregulation in mouse lung samples based on all differentially expressed mRNAs and functional dysregulation. IPA was used to perform pathway analysis on all differentially expressed mRNAs to predict pathway involvement (A) Heatmap displays significantly dysregulated canonical pathways (B-H p-value <.05). Function heatmap (B) displays significantly dysregulated functions (B-H p value <.05). A positive z-score indicates predicted activation of the pathway based on gene expression and a negative z-score indicates predicted deactivation of the pathway based on gene expression. Pathways are hierarchically clustered by z-score. Blue indicates downregulation, red indicates upregulation. Arrows indicate specific pathways of interest.
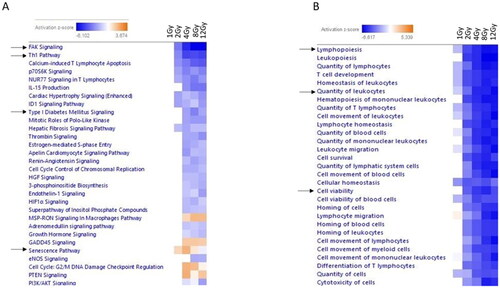
To further elucidate alterations in gene expression across low (1 and 2 Gy) medium (4 Gy) and high (8 and 12 Gy) doses we next studied which pathways were most activated at each point. Apoptosis of lung cancer cell lines was the only pathway significantly activated (z-score > 2) after 1 Gy radiation (data not shown). Since we were focused on normal tissue injury, we chose the Inflammation of joint pathway to display as this was one of the most upregulated pathways by 2 Gy (z-score = 2.22) (). Interestingly, in our data we observed downregulation of Hba and Hbb family members (Hba-a1, Hba-a2, Hbb-b1, Hbb-bt) and Alas2. Through IPA we observed these downregulations were linked to inflammation. However, this may be due to blood cells still present in the lung since no perfusion was performed prior to freezing. Both the Apoptosis of the B lymphocyte pathway and the Hypoplasia of the lymphoid organ pathway were significantly activated (z-score > 2) by 4 Gy. The Apoptosis of the B lymphocyte pathway was the most upregulated pathway at 4 Gy (). The Hypoplasia of the lymphoid organ pathway was most upregulated at 8 and 12 Gy (). In sum, the pathways which were most dysregulated at 1, 2, and 4 Gy, remain dysregulated at higher doses.
Figure 3. Dose impacts the upregulation of genes relevant to Inflammation, Apoptosis and Hyperplasia pathways. IPA was used to discover the most significantly dysregulated pathways based on z-score at low (1 and 2 Gy), middle (4 Gy) and high (8 and 12 Gy) doses in an attempt to highlight potential genes biomarkers for each dose and to determine pathophysiology changes as dose changed. Pathways highlighted include Inflammation of Joint (), Apoptosis of B Lymphocytes (), and Hyperplasia of Lymphoid Organ (). Genes are depicted as fold change of irradiated over control. Red indicated increased expression while blue indicates decreased expression. Genes depicted are ones considered statistically significant a noted at 2 Gy (3A), 4 Gy (3B) and 12 Gy (3C) dose.
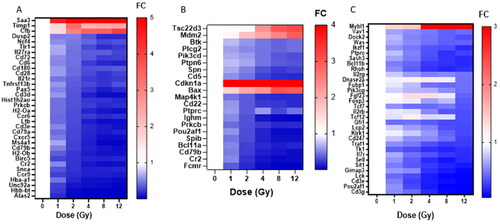
Genes relevant to glucose metabolism become dysregulated as radiation dose increased
Recent publications have linked glucose metabolism disorders such as diabetes, with fibrosis pathways in the heart, lung and liver (Kopf et al. Citation2021; Tuleta and Frangogiannis Citation2021). Further, diabetes and obesity are predisposing factors to radiation pneumonitis (Kong et al. Citation2019; Dong et al. Citation2021; Katsui et al. Citation2021). Therefore, we decided to study genes relevant to glucose metabolism disorder using IPA and prior research to generate a list () and their impact on glucose uptake or resistance are noted in the image (). Slc19a2, Adrb2, ApoD, Aldh1a1, Cfb, Hmgcs2, and Cpt1c show increased expression as the radiation dose increased. Pkib showed decreased expression with increased radiation dose. Interestingly Pdk4 and Fkbp5 show a trend toward decreased expression at 1 Gy, then show increased expression by 12 Gy compared to the control. Sphk1 is elevated at 4 Gy and 12 Gy but is only significant (FC > 2) at 8 Gy. No genes were significantly dysregulated (FC > 2) at 1 Gy. By 4 Gy Slc19a2, Ardb2, ApoD, Aldh1a1, Cfb, Hmgcs2, and Pdk4 were dysregulated (FC > 2). Cpt1c and Pkib only showed significance at 8 and 12 Gy.
Figure 4. Radiation injury dysregulated glucose metabolism. IPA and previous literature were utilized to decide which genes are relevant to glucose tolerance or utilization. These genes are displayed in the heatmap in (A). The impact of each gene’s expression is identified in (B) as to whether they encourage glucose sensitivity or insensitivity. Blue indicates downregulation while red indicates upregulation. Image made with Biorender.
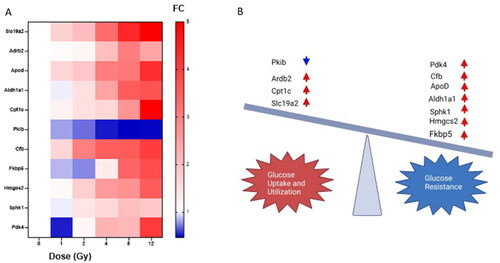
Genes relevant to fibrosis are downregulated in mice at 48 h post radiation
Using both FibROAD (https://www.fibroad.org/browse.html) and FibroAtlas (http://biokb.ncpsb.org.cn/fibroatlas/index.php), we list genes relevant to fibrosis which are significantly altered in our samples in at least one dose after radiation compared to unirradiated mice. Chemokine (Ccl), chemokine receptor (Ccr), CXC motif chomeokine (Cxcl), and CXC motif chemokine receptor (Cxcr) genes were downregulated as the dose increased. Forkhead/winged helix box gene (Fox), genes, except Foxo3, show decreased expression compared to 0 Gy samples, particularly as the dose increased. As shown in , Mmp16 and Mmp8 are upregulated by 12 Gy though Mmp10 is downregulated as the dose increases. The tissue inhibitor of metalloproteinase (Timp) genes and Gdf15 showed increased expression as the dose increased. Integrins (Itg) and Interleukins (Il) were mostly downregulated as the dose increased with the exception of Il-1b. An infographic to summarize our results within broader research is included for clarity ().
Figure 5. Genes relevant to lung fibrosis are mostly downregulated at 48h. FibROAD and FibroAtlas were used to determine which genes dysregulated in our microarray are relevant to lung fibrosis. (A) shows a heatmap of differentially expressed genes in which at least one dose showed |log2FC| > 1 and B-H p-value < .01. (B) A visual summary of dysregulated genes and pathways they are expected to impact. Arrows indicate anticipated activation when genes are upregulated. Blue indicates that genes are downregulated and subsequent impact on pathways and functions would be downregulated. Red indicates that genes are activated and red arros indicate anticipated activation. Image made with Biorender.
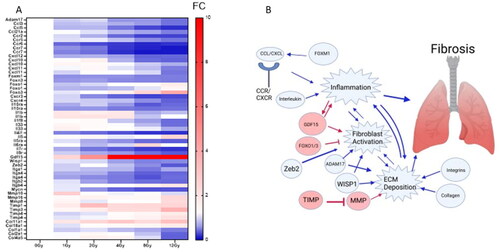
Only three miRNAs were significantly altered in mouse lung after WBI
Whole-genome analysis of miRNA expression indicated that 6 miRNA probes were significantly altered in the lung tissue of WBI mice compared to controls. These 6 probes matched to 3 miRNAs: miR-142-3p, miR-142-5p and miR-34a-5p. Both miRNA 142-5p and 142-3p were downregulated after radiation injury while miR-34a-5p was upregulated (). No miRNAs were dysregulated at 1 Gy. Both probes for miRNA-142-3p were decreased at 2 Gy and continued to remain low for increased doses while both probes for miR-142-5p became significantly decreased at 4 Gy and remained low for higher doses (). One probe for miR-34a-5p was significantly upregulated by 8 Gy and both probes were significantly upregulated at 12 Gy ().
Figure 6. Radiation-induced microRNA expression profiles in mouse lung tissue. Microarray analysis was performed for all samples, and a linear model was fit to each miRNA probe to assess the differential expression of irradiated samples compared to controls. Criteria of |log2FC| > 1 and B-H p-value < .05 relative to controls were used to determine significance and differential expression. (A) The heatmap displays expression patterns, represented by z-score, of all differentially expressed miRNAs across all doses and controls. (B) The number of down-regulated versus up-regulated miRNAs at each dose are shown in the table. (C) Venn diagram shows dose distribution and overlap of differentially expressed miRNAs across all doses. (D) Examples of significant linearly up- and down-regulated miRNAs are shown to display the dose-response to radiation in lung tissue samples. Asterisk (*) indicates statistical significance.
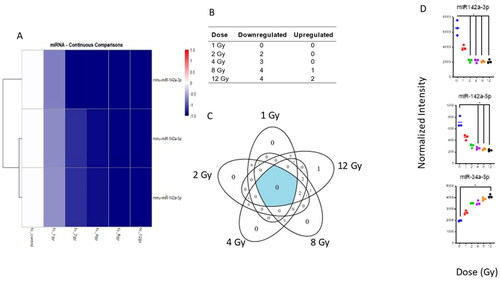
Radiation dysregulates lncRNA expression
Whole genome microarray data was filtered to locate lncRNAs dysregulated by radiation injury. Overall, lncRNA expression increased with increasing doses (). Several lncRNAs were significantly altered across the doses of radiation exposure (Supplemental Table S3). Due to a lack of research in this field, some of the lncRNA remain unnamed. Thus, their systematic IDs are substituted when no other name is available. Among these were Trp53cor1 (chr17:29183003-29217681_R), NR_045989, and Abhd11os (NR_026688) which were significantly upregulated. No lncRNA were shared at low doses (1, 2 Gy) while 6 lncRNA probes were shared at high doses (8, 12 Gy) (). These lncRNA showed a decreasing linear trend with significance only at 12 Gy: AW112010 (NR_102366), NR_045742, and chr7:140081171-140123058_R ().
Figure 7. Radiation-induced long non-coding RNA expression profiles in mouse lungtissue. Whole genome microarray data was filtered to include only probes that correspond to transcripts of lncRNAs. A linear model was fit to each lncRNA probe to assess differential expression of irradiated compared to control samples using criteria of |log2FC| > 1 and B-H p-value < .05. (A) The heatmap displays expression patterns, represented by z-score, of all differentially expressed lncRNAs across all doses and controls. (B) Venn diagram shows dose distribution and overlap of differentially expressed lncRNAs across all doses. (C) The table shows the number of down- versus up-regulated lncRNAs at each dose. (D) Examples of significant linearly up- and down-regulated lncRNAs are shown to display the dose response of lncRNAs to radiation in lung tissue samples. Asterisk (*) indicates statistical significance.
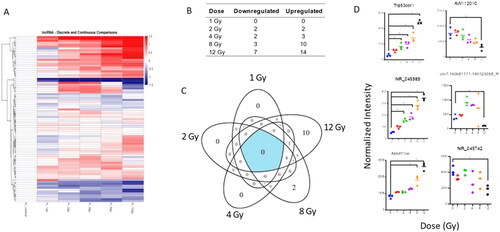
Discussion
Lung fibrosis is a critical dose-limiting factor for patients with thoracic tumors treated with radiation where lung damage can seriously compromise the quality of life. In the present study we identified mRNA, miRNA, lncRNA and pathways which are dysregulated by varying doses of radiation at 48 hours post-WBI. This organ-specific data is useful in discovering and developing new RNA biomarker panels to determine the dose of radiation post-exposure and predict potential pathophysiology in both a clinical and mass casualty setting. For this study we used lung tissue biopsies to determine gene changes after radiation, the next step is to determine if we can observe similar gene or pathway changes using a more accessible sample such as blood. The goal is to develop a noninvasive, possibly high throughput, method to determine radiation biomarkers to indicate both dose of radiation received and anticipate the progression or recovery of an injury.
We used a WBI model to study gene expression changes, however most cancer patients will receive partial body irradiation that limits tissue exposed, one factor in decreasing long-term risks of radiation therapy (Jarzebska et al. Citation2021). Prior data in non-human primates indicates some overlap in gene pathway changes in the lung after whole-body radiation and partial-body radiation at 4 and 7 d after 12 Gy cobalt exposure (Vellichirammal et al. Citation2022). Interestingly, they also observed downregulation of the FAK Signaling, Th1, and Hepatic Fibrosis Signaling among others, which matches our mouse data (Vellichirammal et al. Citation2022). We acknowledge our model has limitations when applied to most cancer patients receiving radiation, however it remains relevant to individuals in a mass casualty scenario and those accidentally exposed. Understanding the course of injury early on can then direct and determine medical triage and shape the discovery of radiation mitigation mechanisms.
No animal model is a perfect replica of human pathophysiology; we acknowledge our main mouse strain C57BL/6 displays pleural effusions and little lung fibrosis which is not frequently observed in humans (Jackson et al. Citation2010). We believe this mouse model in conjunction with C3H/HeJ can highlight shared early biomarkers which may nevertheless be relevant to the development of radiation mitigators for human lung. We observed similar gene changes in C57BL/6 and C3H/HeJ mice after similar doses of x-ray radiation despite differences in dose rate. Prior studies comparing the impact of dose rate on gene expression have focused on dose rate differences of 10-fold or greater (Goudarzi et al. Citation2014; Ghandhi et al. Citation2015). It is unclear how the 2-fold difference in dose rate impacted gene expression changes. Further research to understand how clinically relevant dose rate differences impact gene expression may be useful.
While we do not have long-term data on the impact of radiation doses for this experiment, prior research allows us to anticipate outcomes. The LD50/60 for C57BL/6 mice is approximately 7.69–7.81 Gy (Plett et al. Citation2012). The morbidity of mice is associated with increased airflow resistance and respiratory dysfunction after radiation injury (Jackson et al. Citation2012). Similarly, Andrographolide, a radiation mitigator which protected mouse lung from inflammasome-mediated pyroptosis, immune cell invasion and collagen deposition significantly improved survival; highlighting the importance of protecting lung tissue (Gao et al. Citation2019). The purpose of this paper is to highlight potential early biomarkers which may indicate when mitigators are needed in populations exposed in radiation incidents with high possible exposures (> ∼6–8 Gy).
Pathways relevant to cell cycle arrest, apoptosis, inflammation and tumorigenesis are dysregulated by WBI
Both Gdf15 and Eda2r have been associated with lung fibrosis and radiation injury in our research and others (Wu et al. Citation2012; Himburg et al. Citation2016; de Vries et al. Citation2018; Mukherjee et al. Citation2019; Zhang et al. Citation2019; Jeong et al. Citation2020; Aryankalayil et al. Citation2021). In contrast, there are genes dysregulated by radiation, including Celf5 and Zdhhc22 where no prior research has linked them to normal tissue injury after radiation. This highlights the importance of continued research in this area.
Li et al investigated the impact of 12-Gy thoracic radiation on gene expression 3 d post-radiation in female BALB/c mice (Li et al. Citation2019). Notably, genes observed in both the lungs of their BALB/c and our C57BL/6 mice included: Cdkn1a, Col1a1, Col2a1, Galnt15, Hif3a, and Doc2b (Li et al. Citation2019) (Supplemental Table S1). Further, we also observe dysregulation of the Th1 and Th2 pathways and alterations to the immune response after radiation. A second group highlighted the role of DNA damage and inflammatory response genes in a C57BL/6 mouse model who received 12.5 Gy radiation to the whole thorax (Beach et al. Citation2018). Of interest, both their study and ours observed dysregulation of Cxcr3, Cxcl12, Mmp8, Nhp2, Lck and Mapt; all genes involved in lung fibrosis (Beach et al. Citation2018) (Supplemental Table S1). While our data highlights the Joint inflammation pathway, we suspect these genes are not specific to joint inflammation, rather there is no IPA algorithm to discern inflammation specific to the lung.
Multiple genes relevant to inflammation, mitochondrial function and hemoglobin synthesis were significantly downregulated after radiation, including Hbb-b1, Hbb-b2, Hbb-bt, Hba-a1, Hba-a2, and Alas2. While the role of hemoglobin in oxygen transport is well known, these hemoglobin genes perform other functions as well. As noted by IPA, a decrease in expression of these Hba and Hbb family members also produce increased activation of inflammatory pathways. (Biagioli et al. Citation2009). Decreased expression of Alas2 in blood taken from cancer patients was observed within 16h after 2 Gy radiation therapy (Nie et al. Citation2019).
Radiation injury alters energy expenditure and metabolic processes in mouse lung
Using IPA we focused on genes relevant to glucose uptake or resistance. As noted, glucose concentration in the airway may impact bacterial colonization and hospitalization of patients after radiation injury. Further, glucose metabolism disorders are associated with a greater likelihood of radiation-induced pneumonitis in cancer patients (Kong et al. Citation2019). Briefly, the changes we observe by 12 Gy in mice in Slc19a2, Adrb2, Cpt1c and Pkib indicate increased glucose uptake and utilization (Labay et al. Citation1999; Oishi et al. Citation2002; Gao et al. Citation2009; Blanchet et al. Citation2015; De Franco et al. Citation2015; Jungtrakoon et al. Citation2019; Blondin et al. Citation2020; de Souza e Silva et al. Citation2022). However there are more genes associated with insulin resistance and decreased glucose utilization by 8 and 12 Gy including Sphk1, ApoD, Aldh1a1, Fkbp5, Hmgcs2, Cfb and Pdk4 insulin resistance/decreased glucose use as indicated in (Jeoung and Harris Citation2008; Do Carmo et al. Citation2009; Kiefer et al. Citation2012; Wang et al. Citation2014; Balsevich et al. Citation2017; Sidibeh et al. Citation2018; Geisler et al. Citation2019; Annema et al. Citation2022). Further research is needed to understand the longterm impact of these changes on organ function and survival.
Fibrosis
Lung pneumonitis or fibrosis can develop months after radiation. We sought to determine if any markers for radiation fibrosis could be observed at this early time point. As noted by Dabjan et al. (Citation2016) humans may develop lung pneumonitis weeks after total absorbed doses of 7–10 Gy to the upper body, and lower doses are needed when individuals receive whole-body radiation (Dabjan et al. Citation2016). In prior data, TGF-B is highlighted as the initial driver for fibrosis, in our data we observe the upregulation of one member of the TGF-B family, Gdf15 which is upregulated in response to inflammation and induces fibroblast activation as well as further inflammation in a potential feed-forward cycle (Verhamme et al. Citation2017; Guo et al. Citation2021). Interleukins, Cxcl, Ccl and their receptors function to increase inflammation in response to stimulus through their receptors, though evidence exists indicating they also function in tissue remodeling (Ding and Tredget Citation2015; She et al. Citation2021). Increased expression of A disintegrin and metalloproteinase 17 (Adam17), matrix metalloproteinases (Mmp), collagen genes, Wnt1-inducible signaling protein 1 (Wisp1), and integrins lead to increased extracellular matrix deposition and fibroblast activation, steps in the pathogenesis of fibrosis (Henderson and Sheppard Citation2013; Klee et al. Citation2016; Chen et al. Citation2021). Further experiments using later time points would be useful in understanding whether these early changes accurately predict dysfunction.
Dysregulation of miR-34a-5p and miR-142 after radiation
Only three miRNA, miR-34a-5p, miR-142-3p and miR-142-5p, were dysregulated in total, 48h after radiation injury in the lung. This data agrees with previous studies. In one study, C3H and C57BL/6 received 13.92-13.99 Gy radiation to the whole thorax; both strains showed a significant upregulation of miR-34a-5p in the lungs at 120 and 180 d (Rogers et al. Citation2020). Further, miR-142-5p and miR-142-3p were decreased in the serum of these mice on day 2 and 5 post-irradiation (Rogers et al. Citation2020). In addition, miR-142-5p was found to be decreased in the serum of C57BL/6 mice at both 24 h and 7 d post 2 Gy radiation compared to the control (Acharya et al. Citation2015). Unfortunately, earlier time points for the lung tissue were not performed, so we could not compare these results to our study. Interestingly, in addition, miR-142-5p was found to be decreased in the serum of C57BL/6 mice at both 24 h and 7 d post 2 Gy radiation compared to the control (Acharya et al. Citation2015).
Multiple lncRNA in our study have previously been observed after radiation injury in our research
In contrast, less is known about lncRNA, though its role in cancer and radiotherapy is receiving recent attention (Yao et al. Citation2019; Amirinejad et al. Citation2020). Of particular interest, the lncRNA DNA-damage induced noncoding RNA (DINO) was shown to be upregulated in normal B cells 6h after 2 Gy radiation (Marney et al. Citation2021). Research from our own lab has previously demonstrated dysregulation of Trp53cor1 (also called lincRNA-p21), Abhd11os, and Dino after radiation injury in mouse heart and whole blood (Aryankalayil et al. Citation2018; Aryankalayil et al. Citation2021). Previous genome-wide research has focused on a single dose of radiation over several time points (Zhang et al. Citation2019). This suggests these may be useful markers to determine radiation exposure but they might not be lung-specific markers of radiation injury.
Tumor Protein P53 Pathway Corepressor 1 (Trp53cor1), has multiple functions, including activation of Cdkn1a/p21 and promoting apoptosis (Tran et al. Citation2015; Pal et al. Citation2020), which we have observed in our study. The Abhydrolase domain containing 11, opposite strand (Abhd11os), showed downregulation in the lungs of mice after ventilator-induced lung injury (Xu et al. Citation2018). Earlier studies from our laboratory reported the differential expression of Trp53cor1 and Abhd11os in mouse heart and in mouse blood after whole-body irradiation (Aryankalayil et al. Citation2018; Aryankalayil et al. Citation2021).
Specific genes are altered after WBI in both mouse and pig providing potential cross-species biomarkers
Our study focused on early transcriptional changes in mouse lung after radiation injury. No animals died during this time period. Due to this early time point, we are unable to provide information on the death of animals due to radiation. However, previous work in our lab focused on studying gene dysregulation in Gottingen mini pigs followed for a period of 45 d to access survival after radiation exposure (Chopra et al. Citation2020) This may provide information on shared potential biomarkers of survival (Chopra et al. Citation2020). Interestingly, lipoprotein lipase (Lpl) and Pdk4 were markedly upregulated in the lungs of non-surviving pigs compared to survivors. Mouse data similarly shows that increasing doses of radiation caused a statistically significant increase in Lpl and Pdk4 (Supplemental Table S1). Similarly, we observed the downregulation of polypeptide N-acetylgalactosaminyltransferase 15 (Galnt15) and Tripartite motif-containing protein 55 (Trim55) in non-survivor pigs compared to survivors. While Trim55 decreased as radiation dose increased in mice, Galnt15 increased. In addition, the lungs of both irradiated mice and irradiated pigs showed downregulation of immune pathways including IL-8 signaling.
This investigation focused on RNA dysregulation after varying doses of radiation in the lung to develop biomarkers for bio-dosimetry and for the prediction of long-term radiation-induced lung damage. Through the study of mRNA-miRNA-lncRNA we clarified pathways most dysregulated by radiation including a decrease in cell viability, lymphopoeisis and cell migration as radiation doses increased. We highlighted the importance of miR-34a-5p, miR-142-3p and miR-142-5p as potential miRNA biomarkers both in the microarray of C57BL/6 and through qRT-PCR in both C57BL/6 and C3H/HeJ. We also further validated the dysregulation of Dino andTrp53cor1 through microarray and qRT-PCR.
Future directions
Determining gene expression dysregulation in the lungs 48h after radiation injury provides insight into early markers of damage that may predict long-term outcome. This information will be useful for both treating normal tissue damage in cancer patients post-radiotherapy or for determining radiation countermeasures for those who were accidentally or intentionally exposed to radiation in a nuclear event. Understanding short- and long-term organ injury is crucial to develop and implement treatment post-radiation exposure. Our next steps will be to compare RNA biomarkers from upper thoracic radiation in the serum of nonhuman primate over a longer time course to biomarkers observed in pig and mouse models. This comparison will allow us to see if these markers are identifiable across species. We will also seek to verify the results from this experiment in a human lung-on-a-chip model.
Consent form
All the authors have read and approved the manuscript in all respects for publication.
Author contributions
Conceptualization: M.J.A. and CNC; Methodology: M.J.A., MAB, SM, JMM, MS, LM; investigation, M.J.A., M.A.B., S.M., JD; IE; writing – original draft, M.J.A MAB, SC, SM, AS; writing – review & editing, M.J.A., M.A.B.,C.N.C, S.M., S.C and C.N.C.; funding acquisition, M.J.A C.N.C.; supervision, M.J.A., MAB and C.N.C.
Supplemental Material
Download Zip (2.2 MB)Disclosure statement
No potential conflict of interest was reported by the author(s).
Data availability statement
Data is available at NCBI GEO #GSE202586.
Additional information
Funding
Notes on contributors
Molykutty J. Aryankalayil
Molykutty J. Aryankalayil is a senior scientist at the Radiation Oncology Branch, at National Cancer Institute, at NIH. Her research focuses on using coding and non-coding RNAs as biomarkers to assess radiation injury. To bridge the gap between animal models and static in vitro cell cultures, she is now using microfluidic cell cultures known as human organs-on-a-chip model, for studying radiation-induced normal tissue injury.
Michelle A. Bylicky
Michelle A. Bylicky graduated from the Uniformed Services University of the Health Sciences with a Ph.D. She is currently a Research Fellow in the Radiation Oncology Branch at the National Cancer Institute.
Shannon Martello
Shannon Martello graduated from Johns Hopkins University with bachelors and master’s degrees in chemical and biomolecular engineering. She was postbaccalaureate in the laboratory during this project. She is now working toward a Ph.D. in chemical and biomolecular engineering at Vanderbilt University.
Sunita Chopra
Sunita Chopra received her Ph.D. in cancer biology from the Indian Institute of Science, Bangalore, India. She is currently a visiting postdoctoral fellow in the National Cancer Institute’s Radiation Oncology Branch, where she studies radiation-responsive coding and noncoding RNA signatures in the blood of whole-body-irradiated animal models.
Mary Sproull
Mary Sproull received her doctorate in Biodefense from George Mason University. She is currently a research scientist in the Radiation Oncology Branch at the National Cancer Institute.
Jared M. May
Jared M. May graduated from Penn State University with a degree in chemistry. He was postbaccalaureate in the laboratory during this project. He is now working toward an MD at Northwestern University.
Aman Shankardass
Aman Shankardass graduated from Case Western Reserve University with a degree in Biomedical Engineering. He was an NIH summer intern for two years in the laboratory and is currently working in 3D tissue histology research at LifeCanvas Technologies in Cambridge, MA.
Laurel MacMillan
Laurel MacMillan, MS, is a statistician at Gryphon Scientific whose work has involved the analysis of several novel experimental microarray and sequencing datasets and the development of classification models to predict radiation exposure based on differentially expressed genes. Ms. MacMillan holds a B.A. in mathematics from the University of Virginia and an M.S. in statistics from American University.
Claire Vanpouille-Box
Claire Vanpouille-Box received her PhD from the University of Angers. She was a postdoctoral fellow at NYU Langone Medical Center during this project. She is currently an Assistant Professor at Weill Cornell Medicine.
Iris Eke
Iris Eke received her MD from the Technical University of Munich and her PhD from the Technical University of Dresden, Germany. She was a visiting fellow in the Radiation Oncology Branch during this project. She is currently in the radiation oncology residency at Stanford.
Kevin M. K. Scott
Kevin M. K. Scott graduated from Elon University with a B.S. in biochemistry. He is currently a postbaccalaureate fellow in the laboratory. He is currently applying to graduate programs and is planning to attain his PhD in cancer biology.
Juan Dalo
Juan Dalo graduated from Penn State University with a degree in biomedical engineering. He is currently postbaccalaureate at the laboratory. He is now applying to medical school to pursue an MD.
C. Norman Coleman
C. Norman Coleman received his MD from Yale University. He is the Senior Investigator and project Principal Investigator in the Radiation Oncology Branch, Center for Cancer Research, Associate Director, Radiation Research Program, National Cancer Institute and Senior Medical Advisor, in the Administration for Strategic Preparedness and Response, Department of Health and Human Services.
References
- Acharya SS, Fendler W, Watson J, Hamilton A, Pan Y, Gaudiano E, Moskwa P, Bhanja P, Saha S, Guha C, et al. 2015. Serum microRNAs are early indicators of survival after radiation-induced hematopoietic injury. Sci Transl Med. 7(287):287ra69.
- Agarwal V, Bell GW, Nam JW, Bartel DP. 2015. Predicting effective microRNA target sites in mammalian mRNAs. Elife. 4:e05005.
- Aken BL, Ayling S, Barrell D, Clarke L, Curwen V, Fairley S, Fernandez Banet J, Billis K, García Girón C, Hourlier T, et al. 2016. The Ensembl gene annotation system. Database. 2016:baw093.
- Amirinejad R, Rezaei M, Shirvani-Farsani Z. 2020. An update on long intergenic noncoding RNA p21: a regulatory molecule with various significant functions in cancer. Cell Biosci. 10(1):1–9.
- Annema W, Gawinecka J, Muendlein A, Saely CH, Drexel H, von Eckardstein A. 2022. Elevated levels of apolipoprotein D predict poor outcome in patients with suspected or established coronary artery disease. Atherosclerosis. 341:27–33.
- Aryankalayil MJ, Chopra S, Levin J, Eke I, Makinde A, Das S, Shankavaram U, Vanpouille-Box C, Demaria S, Coleman CN. 2018. Radiation-induced long noncoding RNAs in a mouse model after whole-body irradiation. Radiat Res. 189(3):251–263.
- Aryankalayil MJ, Chopra S, Makinde A, Eke I, Levin J, Shankavaram U, MacMillan L, Vanpouille-Box C, Demaria S, Coleman CN. 2018. Microarray analysis of miRNA expression profiles following whole body irradiation in a mouse model. Biomarkers. 23(7):689–703.
- Aryankalayil MJ, Martello S, Bylicky MA, Chopra S, May J, Shankardass A, MacMillan L, Sun L, Sanjak J, Vanpouille-Box C, et al. 2021. Analysis of lncRNA-miRNA-mRNA expression pattern in heart tissue after total body radiation in a mouse model. J Transl Med. 19(1):336.
- Baker EH, Baines DL. 2018. Airway glucose homeostasis: a new target in the prevention and treatment of pulmonary infection. Chest. 153(2):507–514.
- Balsevich G, Häusl AS, Meyer CW, Karamihalev S, Feng X, Pöhlmann ML, Dournes C, Uribe-Marino A, Santarelli S, Labermaier C, et al. 2017. Stress-responsive FKBP51 regulates AKT2-AS160 signaling and metabolic function. Nat Commun. 8(1):1–12.
- Beach TA, Groves AM, Johnston CJ, Williams JP, Finkelstein JN. 2018. Recurrent DNA damage is associated with persistent injury in progressive radiation-induced pulmonary fibrosis. Int J Radiat Biol. 94(12):1104–1115.
- Biagioli M, Pinto M, Cesselli D, Zaninello M, Lazarevic D, Roncaglia P, Simone R, Vlachouli C, Plessy C, Bertin N, et al. 2009. Unexpected expression of α- and β-globin in mesencephalic dopaminergic neurons and glial cells. Proc Natl Acad Sci U S A. 106(36):15454–15459.
- Blanchet E, Van de Velde S, Matsumura S, Hao E, LeLay J, Kaestner K, Montminy M. 2015. Feedback inhibition of CREB signaling promotes beta cell dysfunction in insulin resistance accession numbers GSE60158 blanchet et al. Cell Rep. 10(7):1149–1157.
- Blondin DP, Nielsen S, Kuipers EN, Severinsen MC, Jensen VH, Miard S, Jespersen NZ, Kooijman S, Boon MR, Fortin M, et al. 2020. Human brown adipocyte thermogenesis is driven by β2-AR stimulation. Cell Metab. 32(2):287.e7–300.e7.
- Do Carmo S, Fournier D, Mounier C, Rassart E. 2009. Human apolipoprotein D overexpression in transgenic mice induces insulin resistance and alters lipid metabolism. Am J Physiol Endocrinol Metab. 296(4):E802–E811.
- Chen G, Feng Y, Sun Z, Gao Y, Wu C, Zhang H, Cao J, Chen Z, Cao J, Zhu Y, et al. 2019. mRNA and lncRNA expression profiling of radiation-induced gastric injury reveals potential radiation-responsive transcription factors. Dose Response. 17(4):155932581988676.
- Chen JY, Cheng WH, Lee KY, Kuo HP, Chung KF, Chen CL, Chen BC, Lin CH. 2021. Abnormal ADAM17 expression causes airway fibrosis in chronic obstructive asthma. Biomed Pharmacother. 140:111701.
- Chopra S, Moroni M, Martello S, Bylicky M, May J, Hritzo B, MacMillan L, Norman Coleman C, Aryankalayil MJ. 2020. Gene expression profiles from heart, lung and liver samples of total-body-irradiated minipigs: Implications for predicting radiation-induced tissue toxicity. Radiat Res. 194(4):411–430.
- Cui Y, Wilder J, Rietz C, Gigliotti A, Tang X, Shi Y, Guilmette R, Wang H, George G, Nilo De Magaldi E, et al. 2014. Radiation-induced impairment in lung lymphatic vasculature. Lymphat Res Biol. 12(4):238–250.
- Dabjan MB, Buck CMS, Jackson IL, Vujaskovic Z, Marples B, Down JD. 2016. A survey of changing trends in modelling radiation lung injury in mice: bringing out the good, the bad, and the uncertain. Lab Invest. 96(9):936–949.
- Ding J, Tredget EE. 2015. The role of chemokines in fibrotic wound healing. Adv Wound Care. 4(11):673–686.
- Dong G, Li Y, Zhao Q, Pang B, Qi X, Wei J, Hou W. 2021. Effects of diabetes on the development of radiation pneumonitis. Respir Res. 22(1):160.
- De Franco E, Flanagan SE, Houghton JA, Allen HL, Mackay DJ, Temple K, Ellard S, Hattersley AT. 2015. The effect of early, comprehensive genomic testing on clinical care in neonatal diabetes: an international cohort study. Lancet. 386(9997):957–963.
- Gao J, Peng S, Shan X, Deng G, Shen L, Sun J, Jiang C, Yang X, Chang Z, Sun X, et al. 2019. Inhibition of AIM2 inflammasome-mediated pyroptosis by Andrographolide contributes to amelioration of radiation-induced lung inflammation and fibrosis. Cell Death Dis. 10(12):1–15.
- Gao XF, Chen W, Kong XP, Xu AM, Wang ZG, Sweeney G, Wu D. 2009. Enhanced susceptibility of Cpt1c knockout mice to glucose intolerance induced by a high-fat diet involves elevated hepatic gluconeogenesis and decreased skeletal muscle glucose uptake. Diabetologia. 52(5):912–920.
- Geisler CE, Ghimire S, Bogan RL, Renquist BJ. 2019. Role of ketone signaling in the hepatic response to fasting. Am J Physiol Gastrointest Liver Physiol. 316(5):G623–G631.
- Ghandhi SA, Smilenov LB, Elliston CD, Chowdhury M, Amundson SA. 2015. Radiation dose-rate effects on gene expression for human biodosimetry Functional and structural genomics. BMC Med Genom. 8(1):22.
- Giuranno L, Ient J, Ruysscher DD, Vooijs MA. 2019. Radiation-induced lung injury (RILI). Front Oncol. 9:877.
- Goudarzi M, Mak TD, Chen C, Smilenov LB, Brenner DJ, Fornace AJ. 2014. The effect of low dose rate on metabolomic response to radiation in mice. Radiat Environ Biophys. 53(4):645–657.
- Guo H, Zhao X, Li H, Liu K, Jiang H, Zeng X, Chang J, Ma C, Fu Z, Lv X, et al. 2021. GDF15 promotes cardiac fibrosis and proliferation of cardiac fibroblasts via the MAPK/ERK1/2 pathway after irradiation in rats. Radiat Res. 196(2):183–191.
- Hall J, Jeggo PA, West C, Gomolka M, Quintens R, Badie C, Laurent O, Aerts A, Anastasov N, Azimzadeh O, et al. 2017. Ionizing radiation biomarkers in epidemiological studies – an update. Mutat Res Rev Mutat Res. 771:59–84.
- Hanania AN, Mainwaring W, Ghebre YT, Hanania NA, Ludwig M. 2019. Radiation-induced lung injury: assessment and management. Chest. 156(1):150–162.
- Henderson NC, Sheppard D. 2013. Integrin-mediated regulation of TGFβ in fibrosis. Biochim Biophys Acta. 1832(7):891–896.
- Himburg H, Sasine J, Yan X, Kan J, Dressman H, Chute J. 2016. A molecular profile of the endothelial cell response to ionizing radiation. Radiat Res. 186(2):141–152.
- Jackson IL, Vujaskovic Z, Down JD. 2010. Revisiting strain-related differences in radiation sensitivity of the mouse lung: recognizing and avoiding the confounding effects of pleural effusions. Radiat Res. 173(1):10–20.
- Jackson IL, Xu P, Hadley C, Katz BP, McGurk R, Down JD, Vujaskovic Z. 2012. A preclinical rodent model of radiation induced lung injury for medical countermeasure screening in accordance with the FDA animal rule. Health Phys. 103(4):463–473.
- Jain V, Berman AT. 2018. Radiation pneumonitis: old problem, new tricks. Cancers. 10(7):222.
- Jakubik D, Fitas A, Eyileten C, Jarosz-Popek J, Nowak A, Czajka P, Wicik Z, Sourij H, Siller-Matula JM, De Rosa S, et al. 2021. MicroRNAs and long non-coding RNAs in the pathophysiological processes of diabetic cardiomyopathy: emerging biomarkers and potential therapeutics. Cardiovasc Diabetol. 20(1):1–29.
- Jarzebska N, Karetnikova ES, Markov AG, Kasper M, Rodionov RN, Spieth PM. 2021. Scarred lung. An update on radiation-induced pulmonary fibrosis. Front Med. 7:585756.
- Jeong I, Lim J, Park J, Oh Y. 2020. Aging-related changes in the gene expression profile of human lungs. Aging. 12(21):21391–21403.
- Jeoung NH, Harris RA. 2008. Pyruvate dehydrogenase kinase-4 deficiency lowers blood glucose and improves glucose tolerance in diet-induced obese mice. Am J Physiol Endocrinol Metab. 295(1):E46–E54.
- Jungtrakoon P, Shirakawa J, Buranasupkajorn P, Gupta MK, De Jesus DF, Pezzolesi MG, Panya A, Hastings T, Chanprasert C, Mendonca C, et al. 2019. Loss-of-function mutation in thiamine transporter 1 in a family with autosomal dominant diabetes. Diabetes. 68(5):1084–1093.
- Kato A, Hulse KE, Tan BK, Schleimer RP. 2013. B lymphocyte lineage cells and the respiratory system. J Allergy Clin Immunol. 131(4):933–957.
- Katsui K, Ogata T, Sugiyama S, Yoshio K, Kuroda M, Yamane M, Hiraki T, Kiura K, Toyooka S, Kanazawa S. 2021. Visceral adipose mass and radiation pneumonitis after concurrent chemoradiotherapy in patients with non-small-cell lung cancer. Cancer Diagn Progn. 1(2):61–67.
- Kiefer FW, Orasanu G, Nallamshetty S, Brown JD, Wang H, Luger P, Qi NR, Burant CF, Duester G, Plutzky J. 2012. Retinaldehyde dehydrogenase 1 coordinates hepatic gluconeogenesis and lipid metabolism. Endocrinology. 153(7):3089–3099.
- Klee S, Lehmann M, Wagner DE, Baarsma HA, Konigshoff M. 2016. WISP1 mediates IL-6-dependent proliferation in primary human lung fibroblasts. Sci Rep. 6:20547.
- Kong M, Lim YJ, Kim Y, Chung MJ, Min S, Shin DO, Chung W. 2019. Diabetes mellitus is a predictive factor for radiation pneumonitis after thoracic radiotherapy in patients with lung cancer. Cancer Manag Res. 11:7103–7110.
- Kopf S, Kumar V, Kender Z, Han Z, Fleming T, Herzig S, Nawroth PP. 2021. Diabetic pneumopathy–a new diabetes-associated complication: mechanisms, consequences and treatment considerations. Front Endocrinol. 12:765201.
- Kultova G, Ichy A, Rehulkova H, Myslivcova-Fucikova A. 2020. The hunt for radiation biomarkers: current situation. Int J Radiat Biol. 96(3):370–382.
- Labay V, Raz T, Baron D, Mandel H, Williams H, Barrett T, Szargel R, McDonald L, Shalata A, Nosaka K, et al. 1999. Mutations in SLC19A2 cause thiamine-responsive megaloblastic anaemia associated with diabetes mellitus and deafness. Nat Genet. 22(3):300–304.
- Li S, Zhang Q-Z, Zhang D-Q, Feng J-B, Luo Q, Lu X, Wang X-R, Li K-P, Chen D-Q, Mu X-F, et al. 2017. GDF-15 gene expression alterations in human lymphoblastoid cells and peripheral blood lymphocytes following exposure to ionizing radiation. Mol Med Rep. 15(6):3599–3606.
- Li Y, Zou L, Yang X, Chu L, Ni J, Chu X, Guo T, Zhu Z. 2019. Identification of lncRNA, MicroRNA, and mRNA-associated CeRNA network of radiation-induced lung injury in a mice model. Dose Response. 17(4):1559325819891012.
- Macaeva E, Saeys Y, Tabury K, Janssen A, Michaux A, Benotmane MA, De Vos WH, Baatout S, Quintens R. 2016. Radiation-induced alternative transcription and splicing events and their applicability to practical biodosimetry. Sci Rep. 6(1):1–13.
- Małachowska B, Tomasik B, Stawiski K, Kulkarni S, Guha C, Chowdhury D, Fendler W. 2020. Circulating microRNAs as biomarkers of radiation exposure: a systematic review and meta-analysis. Int J Radiat Oncol Biol Phys. 106(2):390–402.
- Malaviya R, Gow AJ, Francis M, Abramova EV, Laskin JD, Laskin DL. 2015. Radiation-induced lung injury and inflammation in mice: role of inducible nitric oxide synthase and surfactant protein D. Toxicol Sci. 144(1):27–38.
- Marney CB, Anderson ES, Adnan M, Peng KL, Hu Y, Weinhold N, Schmitt AM. 2021. p53-intact cancers escape tumor suppression through loss of long noncoding RNA Dino. Cell Rep. 35(13):109329.
- May JM, Bylicky M, Chopra S, Coleman CN, Aryankalayil MJ. 2021. Long and short non-coding RNA and radiation response: a review. Transl Res. 233:162–179.
- Mei T, Yang X, Yu Y, Tian X, Deng Q, Xu Y, Zhou L, Zhou X, Liu Y, Zou B, et al. 2022. Secondary infections after diagnosis of severe radiation pneumonitis (SRP) among patients with non-small cell lung cancer: pathogen distributions, choice of empirical antibiotics, and the value of empirical antifungal treatment. Int J Radiat Oncol Biol Phys. 112(1):179–187.
- Mori MA, Ludwig RG, Garcia-Martin R, Brandão BB, Kahn CR. 2019. Extracellular miRNAs: from biomarkers to mediators of physiology and disease. Cell Metab. 30(4):656–673.
- Mukherjee S, Grilj V, Broustas CG, Ghandhi SA, Harken AD, Garty G, Amundson SA. 2019. Human transcriptomic response to mixed neutron-photon exposures relevant to an improvised nuclear device. Radiat Res. 192(2):189–199.
- Nie YH, Liu XD, Huang R, Xie DF, Yin WJ, Guan H, Yu ZJ, Zhou PK. 2019. Analysis of mRNA expression patterns in peripheral blood cells of 3 patients with cancer after the first fraction of 2 Gy irradiation: an integrated case report and systematic review. Dose Response. 17(1):155932581983347.
- Oishi K, Hofmann S, Diaz GA, Brown T, Manwani D, Ng L, Young R, Vlassara H, Ioannou YA, Forrest D, et al. 2002. Targeted disruption of Slc19a2, the gene encoding the high-affinity thiamin transporter Thtr-1, causes diabetes mellitus, sensorineural deafness and megaloblastosis in mice. Hum Mol Genet. 11(23):2951–2960.
- Pal S, Garg M, Pandey A. 2020. Deciphering the mounting complexity of the p53 regulatory network in correlation to long non-coding RNAs (lncRNAs) in ovarian cancer. Cells. 9(3):527.
- Peng Y, Li J, Luo D, Zhang S, Li S, Wang D, Wang X, Zhang Z, Wang X, Sun C, et al. 2021. Muscle atrophy induced by overexpression of ALAS2 is related to muscle mitochondrial dysfunction. Skelet Muscle. 11(1):1–11.
- Pervin M, Unno K, Konishi T, Nakamura Y. 2021. L-arginine exerts excellent anti-stress effects on stress-induced shortened lifespan, cognitive decline and depression. IJMS. 22(2):508.
- Pinnix CC, Smith GL, Milgrom S, Osborne EM, Reddy JP, Akhtari M, Reed V, Arzu I, Allen PK, Wogan CF, et al. 2015. Predictors of radiation pneumonitis in patients receiving intensity modulated radiation therapy for hodgkin and non-hodgkin lymphoma. Int J Radiat Oncol Biol Phys. 92(1):175–182.
- Plett PA, Sampson CH, Chua HL, Joshi M, Booth C, Gough A, Johnson CS, Katz BP, Farese AM, Parker J, et al. 2012. Establishing a murine model of the hematopoietic syndrome of the acute radiation syndrome. Health Phys. 103(4):343–355.
- Ritchie ME, Phipson B, Wu D, Hu Y, Law CW, Shi W, Smyth GK. 2015. Limma powers differential expression analyses for RNA-sequencing and microarray studies. Nucleic Acids Res. 43(7):e47.
- Ritchie ME, Silver J, Oshlack A, Holmes M, Diyagama D, Holloway A, Smyth GK. 2007. A comparison of background correction methods for two-colour microarrays. Bioinformatics. 23(20):2700–2707.
- Rogers CJ, Lukaszewicz AI, Yamada-Hanff J, Micewicz ED, Ratikan JA, Starbird MA, Miller TA, Nguyen C, Lee JT, Olafsen T, et al. 2020. Identification of miRNA signatures associated with radiation-induced late lung injury in mice. PLOS One. 15(5):e0232411.
- Sándor N, Schilling-Tóth B, Kis E, Benedek A, Lumniczky K, Sáfrány G, Hegyesi H. 2015. Growth Differentiation factor-15 (GDF-15) is a potential marker of radiation response and radiation sensitivity. Mutat Res Genet Toxicol Environ Mutagen. 793:142–149.
- Schmitt AM, Garcia JT, Hung T, Flynn RA, Shen Y, Qu K, Payumo AY, Peres-Da-Silva A, Broz DK, Baum R, et al. 2016. An inducible long noncoding RNA amplifies DNA damage signaling. Nat Genet. 48(11):1370–1376.
- Shaverdian N, Veruttipong D, Wang J, Schaue D, Kupelian P, Lee P. 2016. Pretreatment immune parameters predict for overall survival and toxicity in early-stage non-small-cell lung cancer patients treated with stereotactic body radiation therapy. Clin Lung Cancer. 17(1):39–46.
- She YX, Yu QY, Tang XX. 2021. Role of interleukins in the pathogenesis of pulmonary fibrosis. Cell Death Discov. 7(1):52.
- Sidibeh CO, Pereira MJ, Abalo XJ, Boersma G, Skrtic S, Lundkvist P, Katsogiannos P, Hausch F, Castillejo-López C, Eriksson JW. 2018. FKBP5 expression in human adipose tissue: potential role in glucose and lipid metabolism, adipogenesis and type 2 diabetes. Endocrine. 62(1):116–128.
- Singh VK, Pollard HB. 2017. Ionizing radiation-induced altered microRNA expression as biomarkers for assessing acute radiation injury. Expert Rev Mol Diagn. 17(10):871–874.
- Smyth GK. 2004. Linear models and empirical bayes methods for assessing differential expression in microarray experiments. Stat Appl Genet Mol Biol. 3(1):1–25.
- de Souza e Silva S, Leite N, Furtado-Alle L, de Souza RLR, Corazza PRP, Tradiotto MC, Milano GE, da Silva LR, Pizzi J, Lopes MdF, et al. 2022. ADRB2 gene influences responsiveness to physical exercise programs: a longitudinal study applied to overweight or obese Brazilian children and adolescents. Gene. 820:146296.
- Stump B, Cui Y, Kidambi P, Lamattina AM, El-Chemaly S. 2017. Lymphatic changes in respiratory diseases: more than just remodeling of the lung? Am J Respir Cell Mol Biol. 57(3):272–279.
- Tomasik B, Chałubińska-Fendler J, Chowdhury D, Fendler W. 2018. Potential of serum microRNAs as biomarkers of radiation injury and tools for individualization of radiotherapy. Transl Res. 201:71–83.
- Torre-Bouscoulet L, Muñoz-Montaño WR, Martínez-Briseño D, Lozano-Ruiz FJ, Fernández-Plata R, Beck-Magaña JA, García-Sancho C, Guzmán-Barragán A, Vergara E, Blake-Cerda M, et al. 2018. Abnormal pulmonary function tests predict the development of radiation-induced pneumonitis in advanced non-small cell lung cancer. Respir Res. 19(1):72.
- Tran U, Rajarajacholan U, Soh J, Kim T, Thalappilly S, Sensen C, Riabowol K. 2015. LincRNA-p21 acts as a mediator of ING1b-induced apoptosis. Cell Death Dis. 6(3):e1668–e1668.
- Tuleta I, Frangogiannis NG. 2021. Diabetic fibrosis. Biochim Biophys Acta Mol Basis Dis. 1867(4):166044.
- Vellichirammal NN, Sethi S, Pandey S, Singh J, Wise SY, Carpenter AD, Fatanmi OO, Guda C, Singh VK. 2022. Lung transcriptome of nonhuman primates exposed to total- and partial-body irradiation. Mol Ther Nucleic Acids. 29:584–598.
- Verhamme FM, Seys LJM, de Smet EG, Provoost S, Janssens W, Elewaut D, Joos GF, Brusselle GG, Bracke KR. 2017. Elevated GDF-15 contributes to pulmonary inflammation upon cigarette smoke exposure. Mucosal Immunol. 10(6):1400–1411.
- Volovat SR, Volovat C, Hordila I, Hordila DA, Mirestean CC, Miron OT, Lungulescu C, Scripcariu DV, Stolniceanu CR, Konsoulova-Kirova AA, et al. 2020. MiRNA and LncRNA as potential biomarkers in triple-negative breast cancer: a review. Front Oncol. 10:2423.
- de Vries M, Faiz A, Woldhuis R, Postma D, de Jong T, Sin D, Bossé Y, Nickle D, Guryev V, Timens W, et al. 2018. Lung tissue gene-expression signature for the ageing lung in COPD. Thorax. 73(7):609–617.
- Wang J, Badeanlou L, Bielawski J, Ciaraldi TP, Samad F. 2014. Sphingosine kinase 1 regulates adipose proinflammatory responses and insulin resistance. Am J Physiol Endocrinol Metab. 306(7):E756–E768.
- Wang WT, Han C, Sun YM, Chen TQ, Chen YQ. 2019. Noncoding RNAs in cancer therapy resistance and targeted drug development. J Hematol Oncol. 12(1):1–15.
- Wirsdörfer F, Jendrossek V. 2016. The role of lymphocytes in radiotherapy-induced adverse late effects in the lung. Front Immunol. 7:591.
- Wong JL, Evans SE. 2017. Bacterial pneumonia in cancer patients: novel risk factors and current management. Clin Chest Med. 38(2):263–277.
- Wu Q, Jiang D, Chu H. 2012. Cigarette smoke induces growth differentiation factor 15 production in human lung epithelial cells: implication in mucin over-expression. Innate Immun. 18(4):617–626.
- Xu B, Wang Y, Li X, Mao Y, Deng X. 2018. RNA-sequencing analysis of aberrantly expressed long non-coding RNAs and mRNAs in a mouse model of ventilator-induced lung injury. Mol Med Rep. 18(1):882–892.
- Yao Z, Zhang Y, Xu D, Zhou X, Peng P, Pan Z, Xiao N, Yao J, Li Z. 2019. Research Progress on Long Non-Coding RNA and Radiotherapy. Med Sci Monit. 25:5757–5770.
- Zhang S, Du L, Wang L, Jiang X, Zhan Y, Li J, Yan K, Duan W, Zhao Y, Wang L, et al. 2019. Evaluation of serum exosomal LncRNA‐based biomarker panel for diagnosis and recurrence prediction of bladder cancer. J Cell Mol Med. 23(2):1396–1405.
- Zhang T, Cheng G, Sun L, Deng L, Wang X, Bi N. 2019. Transcriptome alteration spectrum in rat lung induced by radiotherapy. Sci Rep. 9(1):1–8.
- Zhang Y, Jiang J, Nouraie M, Roth M, Tabib T, Winters S, Chen X, Sembrat J, Chu Y, Cardenes N, et al. 2019. GDF15 is an epithelial-derived biomarker of idiopathic pulmonary fibrosis. Am J Physiol Lung Cell Mol Physiol. 317(4):L510–L521.