ABSTRACT
Biopolymers are promising potential soil stabilizers due to their ease of application and stabilization efficacy. Biopolymers are biologically occurring polymers that form hydrogels when added to soil in the presence of water. Hydrogels are three-dimensional polymer networks formed through the interaction of polymer chains with soil particles and pore water. The chemical properties of the biopolymer and external factors (like temperature) affect the physical characteristics of the hydrogels formed. Cross-linking of biopolymer chains with another monomer or biopolymer enables the development of hydrogels with enhanced physical integrity and mechanical properties. Recent studies have shown that the biopolymers, guar and xanthan gums, improve the mechanical and durability properties of soil. As a galactomannan, guar gum naturally forms cross links with xanthan gum, and the study presented here evaluates the impact of this cross-linking on plasticity, shrinkage, strength and durability. Cross-linked specimens with higher guar gum have higher plasticity indices and linear shrinkage; however, when the amount of xanthan gum is increased, these values reduce. Strength tests suggest that cross-linking addresses some of the shortcomings of each biopolymer and improves the overall mechanical behaviour of the soil. The durability performance of cross-linked specimens was found to be comparable with specimens stabilized with individual biopolymers.
Introduction
Earth building construction techniques such as rammed earth create structural units (whole walls) using compacted soil-water mixtures. These earthen units need to possess the requisite strength and durability to sustain different structural loads and to resist environmentally driven deterioration. A cementitious stabilizer such as cement is usually added to the soil mixture when the soil used for construction cannot provide the required strength and durability. The addition of cement to the soil to improve its engineering properties is, of course, a common practice employed in many geotechnical applications, in particular ground improvement, and is popular primarily because it rapidly improves both soil strength and durability for a wide range of soils of varying gradation and mineralogy (Bryan, Citation1988). However, there are many downsides associated with using cement as a soil stabilizer. Cement is a major contributor to global greenhouse emissions (Olivier & Peters, Citation2020) and thus increases the carbon footprint of the soil stabilization process. In many cases, the amount of cement used for stabilization in earthen construction has an equivalent carbon footprint of a conventional fired brick or concrete block which makes the stabilization process unsustainable (Lax, Citation2010; Maskell et al., Citation2014). In addition to the carbon costs, standard cements have high embodied energy; they make soil ecologically inert which reduces vegetation growth, they can cause groundwater contamination and finally, they reduce the re-use potential of the soil mixture. This situation has motivated many researchers across the globe to seek more sustainable replacements to cement for stabilization.
Biopolymers have been identified as promising soil stabilizers as they have a simple application procedure and stabilization efficacy. They are naturally occurring polysaccharides synthesized through biological processes and have been extensively used as viscosifiers in food, agriculture and cosmetic industries (Katzbauer, Citation1998; Mudgil et al., 2011). Recent studies have used biopolymers such as starch, alginate, guar and xanthan gums to reduce soil permeability (Bouazza et al., Citation2009; Khatami and O’Kelly, Citation2013), guar, xanthan and gellan gums to increase soil shear strength (Cabalar & Canakci, Citation2011; Chang et al., Citation2015; Soldo et al., Citation2020), xanthan gum to improve compressibility(Latifi et al., Citation2017), guar and xanthan gums to enhance durability (Qureshi et al., Citation2017) and to improve the understanding of long-term performance of xanthan gum stabilized soils (Chang et al., Citation2015; Reddy & Varaprasad, Citation2021). Amongst the different biopolymers which have been previously investigated as a soil stabilizer, guar and xanthan gums are known to possess good stability against temperature and pH changes (Mudgil et al., Citation2014). The intrinsic chemical properties of the biopolymer have a significant influence on the engineering behaviour of the stabilized soil. Guar gum is a neutral polysaccharide having numerous galactose groups to the side chains of the polymer and primarily interacts through chemically weak hydrogen bonds. Hence, when added to soil, guar gum has a significant influence only on the compressive strength of the stabilized soil rather than its tensile strength. Further, with a high affinity towards water, guar gum may hold more water within the soil, which could make the soil susceptible to high shrinkage. In the case of xanthan gum, an anionic polysaccharide, the interaction with soil particles occurs through both ionic and hydrogen bonding which improves both the compressive and tensile strengths of the stabilized soil (Chen, Citation2014). With better tensile strength, xanthan gum stabilized soil would essentially have better durability against water-induced erosion than guar gum stabilized soil (Soldo et al., Citation2020). Though xanthan gum has shown superior performance over guar gum in regards to mechanical behaviour and durability performance, the overall embodied energy and greenhouse gas emissions associated with the production of xanthan gum is substantially higher than that of guar gum (Gresta et al., Citation2014; Krishna Leela & Sharma Citation2000). Also, the energy required in production for xanthan gum is about 10 times greater than for an equivalent amount of cement (Lo et al., Citation1997). Thus, one needs to balance the benefits to the soil properties with measures of sustainability.
On addition to the soil, biopolymers initially interact with pore water to form ‘hydrogels’ and in turn, these hydrogels interact with soil particles by either coating themselves to the coarse-grained soil particles and/or by forming chemical bonds with clay particles(Chen et al., Citation2016). These complex interactions between biopolymer hydrogels, pore water and soil particles lead to the formation of a 3D network of hydrogels connecting soil particles which provide a stabilizing effect to the soil (Ayeldeen et al., Citation2016; Chang et al., Citation2015; Mendonça, Citation2020). Further on drying, these hydrogel formations connecting the soil particles change their physical nature from a ‘rubbery’ to a ‘glassy’ state which has a significant influence on soil strength improvement (Ayeldeen et al., Citation2016). The physical integrity, mechanical properties and the chemical activity of the hydrogels are dependent on the chemical properties of the biopolymer (Gulrez et al., Citation2011; Varaprasad et al., Citation2017). On saturation, the outer chains of the biopolymer absorb and hold water which fills up the voids in the soil matrix. This makes the hydrogels swell before starting their dilution, which is the process where the hydroxyl groups of the hydrogel dissociate and dissolve in the water. The amount of water held by the hydrogels and the time taken for complete dilution depends on the intrinsic chemical properties of the biopolymer (Gulrez et al., Citation2011; Nugent et al., Citation2009). To delay or avoid this dilution of the hydrogels, they are usually cross-linked (Ullah et al., Citation2015; Varaprasad et al., Citation2017), which is a process where the outer chains of the biopolymer are made to interact with other polymer chains to enhance the physical integrity and mechanical properties of the hydrogel. Literature recommendations suggest that neutral polysaccharide galactomannans such as guar gum instantaneously form cross-links with a helix-forming polysaccharides such as xanthan gum without any initiation (Casas et al., Citation2000; Katzbauer, Citation1998). Cross-linking between guar and xanthan gums has been used for stabilizing of fly ash suspensions to retard spontaneous coal combustion, for long-term stabilization of iron nanoparticles in groundwater remediation and to improve fluid properties in hydraulic fracturing (Fischer et al., Citation2001; Shi et al., Citation2018; Xue & Sethi, Citation2012, respectively). Very few studies, however, have focussed on cross-linking specifically for soil stabilization. Chen (Citation2014) and Ding et al. (Citation2019) have previously used guar and xanthan gum cross links to improve the resistance of mine tailings against wind erosion. Chen (Citation2014) reported that cross-linking lowered the rate of erosion of the stabilized material, while Ding et al. (Citation2019) reported that cross-linked specimens with higher concentrations of xanthan gum had better penetration resistance and dust control performance. However, there are no studies that report the effect of cross-linking on soil plasticity, strength gain and durability. In the following study, a novel assessment of cross-linking guar and xanthan gums is made to understand its impact on soil plasticity, strength gain and durability of earthen construction material such as rammed earth.
Materials and methods
In this study, the effectiveness of cross-linking of biopolymers for soil stabilization was evaluated on a soil mixture used for manufacturing earthen construction materials like rammed earth or compressed earth. An engineered soil mixture was used comprising 20% refined kaolin, 70% sand and 10% gravel by mass (denoted as Soil 2-7-1) (Chemical analysis of the kaolin by X-ray fluorescence showed SiO2 47% and Al2O3 38%) was chosen for this study, as it suits the recommendations from a number of sources for earthen construction materials (Houben & Guillaud, Citation1994; Olivier & Mesbah, Citation1987) and following much previous work in this area using this mixture. As the engineered soil mixture is classified as Clayey Sand (SC) as per UK standard BS Citation5930 (Citation2015), the engineering behaviour of this soil mixture can be considered representative of natural soils with similar physical properties. The physical properties of the unamended engineered soil mixture, namely, the Atterberg limits and linear shrinkage were determined in accordance to BS Citation1377-Citation2 (Citation1990) and compaction properties (using the 2.5 kg Proctor test) in accordance with BS Citation1377-Citation4 (Citation1990). presents the physical properties of unamended engineered soil mixture.
Table 1. Physical properties of the unstabilized soil mixture used in this study.
The two biopolymers used in this study, namely, guar and xanthan gums, in powdered form, were obtained from a company in the UK. Guar gum is processed from the endosperm of a cluster beans (Cyamopsis tetragonolobus) belonging to Leguminosae plant species, an annual crop usually grown in semi-arid regions (mainly in India and Pakistan). Chemically, guar gum is a neutrally charged polysaccharide composed of galactose and mannose groups (Chudzikowski, Citation1971; Mudgil et al., Citation2014). Xanthan gum is biologically synthesized from a plant-based pathogenic bacterium called Xanthomonas campestris. It is chemically an anionic polysaccharide which has repeated pentasaccharide units formed by two glucose units, two mannose units and glucuronic acid unit in its backbone and glucuronic acid between two mannose units in its trisaccharide side chains (Becker et al., Citation1998; Garcıa-Ochoa et al., Citation2000; Katzbauer, Citation1998). Both of these biopolymers have been used extensively as thickening agents in the food, cosmetic and pharmaceutical industries due to their ability to remain volumetrically stable against varying temperature and pH conditions (Katzbauer, Citation1998; Mudgil et al., 2011). Only in the recent past, they have been investigated for the purpose of soil stabilization due to their ability to improve various soil properties in a relatively short span of time. Based on the past literature recommendations (Chang et al., Citation2015), the total amount of combined biopolymer content for cross-linking in this study was kept at 2.0% of the dry soil mass. The different combinations of biopolymer cross-linking used in this study are presented in . As per the recommendations given in the literature, earthen construction material stabilized with 8% cement has comparable compressive strength (3–5 MPa) of a fired brick (Kerali, Citation2001; Venkatarama Reddy & Jagadish, Citation1989). Hence, the performance of the biopolymer stabilized samples in this study was compared with 8.0% cement stabilized samples. CEM II type cement which was used in this study conformed to the specifications set out in BS EN Citation197-Citation1(Citation2011).
Table 2. Stabilizer combinations along with amount of additional water needed for different biopolymer contents.
Atterberg limits and linear shrinkage tests
The engineered soil mixture was taken on a 425 µm sieve and washed thoroughly until no visible fine portion of the soil passed through the sieve (BS Citation1377-Citation2 (Citation1990)). The portion of the soil fraction which passed through the sieve was retained and dried in the oven for 24 h at 100 ± 5 °C. After 24 h of drying, the soil fraction was broken down into smaller fines using a rubber mallet; required quantities of biopolymer/s were then introduced to these soil fractions and mixed thoroughly with distilled water until a firm-to-stiff consistency was achieved. The wet mixture was then stored in air-tight polythene bags to equilibrate for 24 h, after which in accordance to BS Citation1377-Citation2 (Citation1990) liquid limit (using cone penetration method), plastic limit and linear shrinkage tests were conducted.
Strength tests
Unconfined compression tests
Cylindrical specimens of dimension 38 mm diameter and 76 mm height were prepared and tested in unconfined compression (UC). Initially, the engineered soil mix and biopolymer were mixed thoroughly before adding the water equivalent to the optimum water content (OWC) of the unamended soil mixture. It was observed that, additional water above OWC was needed for a biopolymer stabilized sample to make the soil mix workable. The quantity of additional water needed to make the soil mix workable varied with the type and quantity of biopolymer used in a particular combination and was determined prior to the preparation of each sample. presents the quantity of additional water required for different biopolymer combinations. After the water was added, the soil was thoroughly mixed for about 2–3 min until a uniform mixture was obtained. This wet soil mix was then introduced into UC specimen mould and statically compacted in three layers to achieve the dry density of 19.62 kN/m3, which equals the maximum dry density of the unamended soil mixture. The compacted sample was then extruded out of the mould and left to dry cure under the laboratory environment (relative humidity (RH) of 50% and temperature of 21 °C) and later tested after 7 and 28 days. For each designated curing period, three replicates were prepared and tested for UC in accordance with the British Standard (BS Citation1377-Citation7, Citation1990).
On the completion of the designated curing period, the mass and dimension of the test specimen were recorded prior to testing. As the surface of the test specimen was level without any capping the test specimen was set under the loading platen of a Shimadzu universal testing machine (). The specimen was then uniaxially loaded at a controlled displacement rate of 0.5 mm/min until failure. The selected displacement rate of 0.5 mm/min was based on the previous tests conducted by Beckett (Citation2011). Loads and displacements during the test were automatically recorded using the software TRAPEZIUM LITE X. For assessing the performance of biopolymer stabilization, identical tests were carried out on samples of the unamended soil after 7 days of air curing and on samples stabilized with 8.0% cement by mass after 7 and 28 days of air curing. Like biopolymer-stabilized samples, air curing took place inside the laboratory atmosphere.
Tensile tests
Determination of tensile strength of earthen construction materials is often neglected as it is considered to be low, however, its estimation is crucial in understanding its behaviour under seismic conditions (Araki et al., Citation2016; Bui et al., Citation2014) and for durability. In order to determine tensile strength, ‘bowtie’ shaped specimens were prepared and tested in tension using the procedure outlined in Stirling et al. (Citation2015). The specimens possess a mirrored isosceles trapezium plan cross-section (27 × 16 × 46 mm), where the presence of the reduced cross-sectional area at the centre, induces tensile failure at the neck of the specimen ((b)). The test specimens were manufactured in a bow-tie-shaped mould and otherwise, the preparation procedure of these specimens was similar to that of UC test specimens, i.e. compacting the soil in three layers in a sample mould to achieve the required dry density. Biopolymer stabilized tensile specimens were cured under the same conditions as that of the UC test specimens and then also tested after 7 and 28 days. On the day of testing, the mass and dimension of the cured specimens were noted. The test specimen was introduced into the modified 60 mm square direct shear testing rig. The sample was secured between the loading jaws of the modified rig. By action of the motor of the testing rig, the carriage jaw moves away from the restrained jaw uniaxially, which induces tension on the sample. The rate of deformation can be controlled similarly to a direct shear test, in this case, it was maintained at 0.5 mm/min. The loading on the sample continued until obvious failure. Load and displacement values were recorded manually. For all the specimens tested, the testing duration was about 8–10 min.
For comparison, identical tests were carried out on samples of the unamended soil after 7 days and on samples stabilized with 8·0% cement by mass after 7 and 28 days of air curing.
Durability tests
The impact of cross-linking on durability against rainfall-induced erosion was assessed in accordance with the Geelong drip test as described in New Zealand Standard NZDS Citation4298 (Citation1998). For drip tests, test specimens in the form of 150 mm × 150 mm × 20 mm tiles were prepared. The tiles were manufactured in the same way as the other specimens but in a steel 150 mm cube mould; however, the bulk mass of the soil mixture was statically compacted to a single layer to achieve the maximum dry density of the unamended soil mix using a Denison T60C hydraulic press. To avoid drainage and ensure safe extrusion of the sample, the cube mould was lined with Teflon paper. To achieve the required thickness of 20 mm, the compacting pressure had to be maintained around 2.0–2.3 MPa. Once compacted, the cube mould was dismantled, and the tile specimen carefully removed. The tiles were then left to cure in the laboratory atmosphere at a RH of 50% and a temperature of 21 °C. Geelong erosion tests were then performed on samples cured for 7 and 28 days. The test procedure involves the dripping of 100 mL of deionized water using a peristaltic pump within 60 min from a height of 400 mm onto the surface of the sample; this simulates the effect of 500 mm of annual rainfall (Heathcote, Citation1995). The test specimen was kept at an inclination of 2H:1 V. At the end of the test, the depth of erosion was recorded using the depth rod of a Vernier calliper for each tested specimen ().
Results and discussion
Effect of cross-linking on soil plasticity and linear shrinkage
presents the results of Atterberg limits and linear shrinkage tests for all samples in this study. The liquid and plastic limits for the unamended soil mixture were 36.2% and 18.4%, respectively. For all the biopolymer stabilized combinations, the liquid limit was higher than that of the unamended soil mixture. Within the biopolymer stabilized combinations, the guar gum stabilized soil mix had the highest liquid limit, while the xanthan gum stabilized soil mix had the least. From the liquid limit values for cross-linked combinations, it can be observed that as the percentage of xanthan gum increased within the combination, the liquid limit decreased. It can be noted from , unlike the liquid limit, the effect of biopolymer on the plastic limit of the stabilized soil mix was less significant. The plastic limit of the cross-linked combinations was slightly higher than that of other combinations, indicating that the application of biopolymers in combination induces cross-linking which influences soil plasticity. The impact of biopolymer stabilization on soil plasticity is better understood through the plasticity chart plotted in . The classification of the fine portion for the unamended soil fraction as per BS Citation1377-Citation2 (Citation1990) was Clay of intermediate plasticity (CI). With the addition of the biopolymer, this classification changed to Clay of high plasticity (CH) except for soil stabilized only with xanthan gum. However, the effect of cross-linking is quite evident from the plasticity chart, as the amount of xanthan gum increases in the soil (or guar gum decreases), its plasticity index reduces. The linear shrinkage value of the unamended soil mixture was 5.0%. For the guar gum stabilized specimen, the linear shrinkage value was at 12.1%, while for xanthan gum it was 6.0%. The variation in linear shrinkage values for cross-linked specimens had a similar decreasing trend as that of liquid limit, i.e. as the amount of xanthan gum in the soil mix increased, the linear shrinkage reduced. The influence of xanthan gum on the volumetric stability of the cross-linked specimen is quite evident.
Table 3. Atterberg limits and linear shrinkage values.
The changes in liquid limit and linear shrinkage values for biopolymer stabilized soil mixes can be attributed to the intrinsic chemical properties of the biopolymers and the type of chemical interaction they have with the clay particles. Nugent et al. (Citation2009) noted that on the addition and mixing of biopolymers, two important interactions occur within the soil matrix. Firstly, the hydroxyl groups of the biopolymer readily interact with pore water which increases its viscosity, and this tends to increase the liquid limit of the soil. Secondly, the interaction between biopolymer chains and clay particles leads to soil agglomerations which, in turn, reduces the overall surface area of soil particles. Thus, lower amounts of water are required to hydrate these agglomerations, and this tends to lower the liquid limit of the soil. The combination of these two interactions determines the final liquid limit of the soil. Being a neutral polysaccharide, guar gum essentially interacts with pore water and clay particles in the soil matrix through hydrogen bonding (Chudzikowski, Citation1971). Having a higher affinity towards water, the hydroxyl ions interact of the polymer chains interact more with pore water than the clay particles within the soil matrix. Hence, the effect of viscosity will be more predominant in guar gum stabilized soil (Nugent et al., Citation2009) and thus the observed liquid limit is far higher than that of other combinations. On drying, these hydrogen bonds are easily broken, and the large amount of water held by the biopolymer chains of the guar gum escapes from the soil mix, thus, the observed linear shrinkage values are higher than other biopolymer combinations. On the other hand, xanthan gum is an anionic polysaccharide which will readily interact with clay particles within the soil matrix and form larger and stable agglomerations through ionic bonding (Chang et al., Citation2019). These stable agglomerations reduce the overall surface area within the soil mixture and thus the observed liquid limit is lower than all other combinations. Even on drying, these agglomerations remain stable and provide volumetric stability for the soil, thus the observed linear shrinkage value is lower than all other combinations. In the case of cross-linked combinations, the change in liquid limit and linear shrinkage values are not proportionate with the amount of biopolymer present. This indicates apart from the above interactions of the individual biopolymer, the cross-linking of the two biopolymers also has an influence on the soil plasticity and shrinkage properties. As observed in , there is a slight increase in plasticity index and linear shrinkage for specimens with higher concentrations of guar gum; however, as the amount of xanthan gum in the other two cross-linked combinations was increased, these values reduced. The results suggest that equal or higher proportions of xanthan gum would be effective during cross-linking; otherwise, the effect of cross-linking may be considered less favourable in tackling soil plasticity and shrinkage.
Effect of cross-linking on soil strength
(a,b) shows the axial stress/strain plots recorded during the UC tests for all samples after 7 and 28 days of curing. Key conclusions should be drawn from the results >0.5% strain as the differences in stiffness at low axial strains are caused by sample bedding. At 7 and 28 days, there is a considerable difference in stiffness between biopolymer combinations, with higher concentrations of xanthan gum leading to higher stiffness. The increased stiffness after 28 days appears primarily due to the transformation of the physical state of the hydrogels from a ‘rubber’ to’ a glassy’ state (Ayeldeen et al., Citation2016)
Figure 5. Axial stress versus strain plots for all biopolymer combinations (a) 7 days and (b) 28 days.
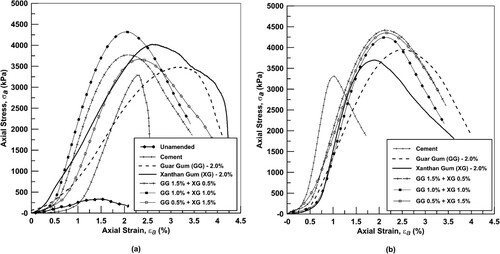
(a,b) shows the stiffness and UC peak strength for all combinations for both 7 and 28 days of curing. The average compressive strength of an unamended specimen was 370 kPa, while for cement stabilized specimens, it was 2779 and 3076 kPa after 7 and 28 days, respectively. The compressive strengths of all biopolymer combinations were in the range of 3550–4326 kPa, which are higher than unamended specimens and are approximately 35% higher after 7 days and 30% after 28 days than cement stabilized specimens. Though all biopolymer combinations have shown higher strength than unamended and cement stabilized specimens, there was no optimum combination of cross-linking which has shown significantly higher compressive strength. presents the peak tensile strength for all combinations tested in this study. All biopolymer combinations showed higher tensile strength than unamended samples after 7 and 28 days curing. It can be observed from that after 7 days of curing, all biopolymer combinations had lower tensile strengths than cement stabilized specimens; however, after 28 days, biopolymer combinations with xanthan gum content > 1.0% had higher tensile strengths than cement stabilized specimens.
Figure 6. UC strength results for all samples tested in this study. (a) Stiffness and (b) peak strength.
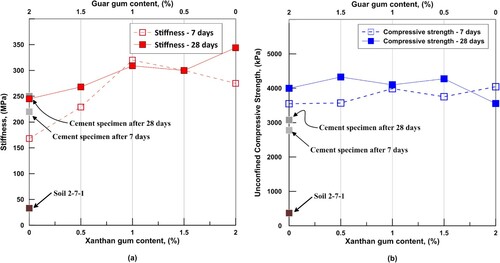
The mechanical behaviour of individually stabilized biopolymer specimens has been previously reported by Muguda et al. (Citation2017). It was noted that the hydrogel formation and soil suction contribute to both compressive and tensile strength of the biopolymer stabilized soil. In the case of guar gum stabilized soil, once the hydrogels transform to a glassy state on drying, they tend to reduce soil suction and contribute only to the improvement of compressive strength. With reduced soil suction and weaker hydrogen bonds, the hydrogels do not contribute to tensile strength improvement. For xanthan gum stabilized soil, after 28 days, there was a slight decrease in compressive strength; however, the ionic bonding of the biopolymer chains and hydrogel transformation improved soil suction and, in turn, contributed to stiffness and tensile strength. The results from the strength tests in the present study seem to suggest that cross-linking the biopolymers has addressed the shortcomings of both biopolymers. All cross-linked specimens did not show any signs of reduction in compressive strength after 28 days unlike xanthan gum stabilized specimens. From the tensile tests presented in the previous study, the tensile strength of guar gum stabilized specimens reduced after 28 days even for specimens prepared with higher biopolymer content (up to 3.0% of dry soil mass). The tensile strength after 28 days for 1.5% guar gum stabilized specimen was 55 kPa, while it was 165 kPa for 1.5% xanthan gum stabilized specimen. In the present study, after 28 days, the tensile strength of the cross-linked specimen with 1.5% guar gum and 0.5% xanthan gum was about 200 kPa. The higher tensile strength for this specimen can be attributed to the interactions which occur between guar and xanthan gum biopolymer chains. Hence it can be concluded that in the case of cross-linked specimens, additional interaction between individual biopolymer chains and with soil particles contributes to the stabilization process (Fischer et al., Citation2001; Mwamufiya, Citation1998). This shows that small replacements of guar gum with xanthan gum result in an improvement in tensile strength. At equal proportions of guar and xanthan gums and with higher xanthan gum, the tensile strength of cross-linked specimens after 28 days is higher than that of a cement stabilized soil specimen. The increase in tensile strength through the addition of biopolymers would be an added advantage in improving the seismic resistance of biopolymer stabilized earthen construction materials. Further, having addressed the shortcomings of each biopolymer, cross-linking has facilitated better stabilization in terms of better mechanical behaviour of the stabilized material.
Effect of cross-linking on soil durability
shows typical failure surfaces for unamended, cement and biopolymer stabilized tiles after the completion of drip tests. It can be noticed from the figure that unamended samples have higher eroded depth with an erosion of fine-grained particles from adjacent areas of the pitted depth of the tile, while cement stabilized samples had the smallest eroded depths. In the case of biopolymer stabilized tiles, the final eroded depths were less than unamended and higher than that of cement stabilized samples with no visible surface erosion of fine-grained particles. presents the final eroded depths at the end of the test for tiled specimens for all the combinations considered. In the plot, for each combination, erosional depth measured at 7 and 28 days are plotted adjacent to each other. Based on the recommendation given by NZS Citation4298 (Citation1998), unamended specimens have a high erodibility index of 3, while for the cement stabilized specimen it was 2. For all biopolymer combinations, the final depths of erosion were well within 5 mm and the erodibility index was 2. Between the biopolymer stabilized specimens, guar gum stabilized specimens had the highest erosional depths, while the xanthan gum stabilized specimen showed greater resistance against erosion. The performance of the cross-linked specimens is similar to that of individually stabilized specimens. As the amount of xanthan gum in the cross-linked specimen increases the depth of erosion reduced. As per Frencham (Citation1982), biopolymer stabilized specimens which have erosional depths lesser than 5 mm can be classified to be ‘slightly erodible’ and in terms of NZS Citation4298 (Citation1998), they may be subjected to more adverse durability test such as the spray test for precise assessment.
Figure 8. Typical failure surfaces after drip tests (a) unamended, (b) cement stabilized tiles and (c) biopolymer stabilized tiles.
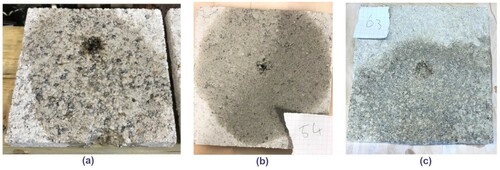
To date there are very few studies in the literature which have extensively investigated the durability performances of biopolymer stabilized soils. Qureshi et al. (Citation2017) have compared the slake durability performance of xanthan gum stabilized subgrade with that of cement stabilized material. It was reported that 10% cement stabilized subgrade material showed weaker durability performance than xanthan gum stabilized materials with stabilizer content higher than 2.0% of the dry soil mass. It was noted that the repeated wetting and drying cycles led to degradation of cement stabilized material, while in xanthan gum stabilized material, the wetting aided the hydrogels within the soil to rehydrate and hold the sand particles together. Ding et al. (Citation2019) is another study that reports the durability performances of xanthan-guar cross-linked soils against wind-driven erosion. It was noted that the viscosity of the biopolymer solutions was directly linked with penetration resistance and durability performance. Further, it was observed that guar and xanthan cross links (at 1:3 ratio) provided the best performance in both strength and durability. These studies suggest that the ability of the biopolymer to alter the properties of water within the soil matrix leads to the improvement of soil’s durability. This stabilization effect of the biopolymers is also seen in the drip tests conducted. As observed here, the repeated dripping of water on the earthen materials leads to erosion of fine-grained particles from the surface and localized saturation. As observed in , the addition of biopolymer prevents the erosion of the fine-grained particles due the interactions of polymer chains with clay particles within the earthen material, unlike that of unamended samples in which fine-grained particles wash off during dripping. Further, as the repeated dripping creates localized saturation, the ability of the biopolymer to absorb and hold water prevents pitting of the material leading to lesser eroded depths, as seen from the results presented in . The performance of biopolymer stabilized samples in these two fronts of deterioration is directly linked with physical and chemical characteristics of the hydrogels formed and the results certainly suggest that the cross-linking of xanthan gum with guar gum would provide better durability performance as against only guar gum stabilized samples. The tests in this study, though aimed at assessing construction materials, have revealed the reliable stabilization performance of biopolymers against water-induced erosion. The test results are promising for using biopolymers in this way and making use of aspects of cross-linking to improve soil durability in any other ground improvement cases.
Implications for biopolymer soil stabilization
As indicated above, biopolymers are extensively used as stabilizers in food, cosmetic and other industries to stabilize a wide range of materials (Mudgil et al., 2011) and there is a growing research interest within the scientific community to understand the capabilities of using biopolymers as a soil stabilizer. In comparison with cement, there are several advantages of using biopolymers as a soil stabilizer. Firstly, the amount of biopolymer content required to stabilize an equivalent amount of soil is about one-fourth as that of cement(Chang et al., Citation2015; Chang et al., Citation2015) with no special curing requirements. Hence, in many practical applications, only small quantities of biopolymers would be required to stabilize large quantities of soil. Without the need of any special curing techniques, biopolymers may be used effectively in constrained spaces for stabilization purposes. About 95% of strength gain for biopolymer stabilized soils occur within 7 days of application (Ayeldeen et al., Citation2016), while for cement stabilized soils the time taken is assumed to be around 28 days. The rapid gain of soil strength due to the addition of biopolymers can ensure the structure element (rammed earth walls) can become functional and thus speed up the construction process. All these promising characteristics of biopolymer stabilization make them a productive alternative for soil stabilization.
At the same time, there are few downsides associated with biopolymer stabilization which may, in the end, limit their practical application. The hydrogels formed by certain biopolymers (like guar gum) are hydrophilic in nature which will draw pore water into the soil matrix. This will have a significant influence on the soil plasticity and shrinkage properties and may pose problems to the volumetric stability of the soil. Out of the available biopolymers, only a few have shown comparable stabilization performance to cement and of those, xanthan gum appears to be one of the best choices for soil stabilization. Biopolymer stabilized soils are susceptible to deterioration on full saturation, which may potentially limit their use in some geotechnical applications. It is also yet to be ascertained whether biopolymer production can cater to the requirement of construction needs if they are actively used as a stabilization agent. To date, there are no studies that report full-scale implementation of biopolymers thus, they have yet to be field verified. Also, without a full Life Cycle Assessment, their exact sustainability credentials cannot be ascertained, and this may also limit their practical application.
Cross-linking of biopolymers may address the issues concerning biopolymer choice, volumetric stability, strength degradation and sustainability credentials. In the food and pharmaceutical industries, it is a common practice to cross-link a biopolymer to improve the stabilization process (Ullah et al., Citation2015; Varaprasad et al., Citation2017). Cross-linked hydrogels tend to have better physical integrity and stability against varying pH and temperature. As seen from the present study, optimized cross-linking between guar and xanthan gum appears to lead to better volumetric stability of biopolymer stabilized soils, addressing the shortcomings of each biopolymer in their contribution towards soil strength and have comparable durability performance as against individually stabilized specimens and cement stabilized specimens. Considering cross-linking can be achieved in different techniques such as the introduction of ionic biopolymer, chemical initiator or thermal treatment, there are many unexplored ways in which the soil can be stabilized through cross-linking.
Conclusions
Biopolymers have been identified as promising replacements to cement for soil stabilization in many geotechnical applications. Based on the recommendations given in the literature that guar and xanthan gums readily interact with each other and form cross-links, this study has made a novel attempt to cross-link guar and xanthan gums with each other to stabilize an earthen construction material. Higher concentrations of guar gum in cross-linked specimens resulted in higher plasticity index and linear shrinkage values; however, equal or higher proportions of xanthan gum in cross-linked specimens had better volumetric stability. Cross-linking the biopolymers with each other in a way addressed the shortcomings of each biopolymer, i.e. improvement of tensile strength and no signs of reduction in strength after 28 days. Further, all cross-linked specimens exhibited similar durability performance against water-induced deterioration as that of the specimens stabilized only with guar or xanthan gums. Cross-linking combinations consisting of equal amounts of xanthan and guar gums were found to have comparable behaviour of cement stabilized specimen and hence higher amounts of xanthan gum are not required to achieve desired strength and durability. Any future studies which can precisely quantify the cross-linking between xanthan and guar gum in relation to soil stabilization would improve the understanding of stabilization mechanisms and improve the efficacy of the stabilization process. Considering biopolymers can be cross-linked in several different ways, it gives an opportunity to alter biopolymer stabilization and modify the soil in ways they have not been able to do so with existing stabilizers such as cement.
Disclosure statement
No potential conflict of interest was reported by the author(s).
Additional information
Funding
References
- Araki, H., Koseki, J., & Sato, T. (2016). Tensile strength of compacted rammed earth materials. Soils and Foundations, 56(2), 189–204. https://doi.org/10.1016/j.sandf.2016.02.003
- Ayeldeen, M. K., Negm, A. M., & El Sawwaf, M. A. (2016). Evaluating the physical characteristics of biopolymer/soil mixtures. Arabian Journal of Geosciences, 9(5), 1–13. https://doi.org/10.1007/s12517-016-2366-1
- Becker, A., Katzen, F., Pühler, A., & Ielpi, L. (1998). Xanthan gum biosynthesis and application: A biochemical /genetic perspective. Applied Microbiology and Biotechnology, 50(2), 145–152. https://doi.org/10.1007/s002530051269
- Beckett, C. (2011). The role of material structure in compacted earthen building materials: Implications for design and construction. Durham University. http://etheses.dur.ac.uk/3313/
- Bouazza, A., Gates, W. P., & Ranjith, P. G. (2009). Hydraulic conductivity of biopolymer-treated silty sand. Géotechnique, 59(1), 71–72. https://doi.org/10.1680/geot.2007.00137
- Bryan, A. J. (1988). Criteria for the suitability of soil for cement stabilization. Building and Environment, 23(4), 309–319. https://doi.org/10.1016/0360-1323(88)90037-6
- BS 1377-2. (1990). Methods of test for soils for civil engineering purposes – part 2 : classification tests. BSI.
- BS 1377-4. (1990). Methods of test for soils for civil engineering purposes - part 4 : compaction tests (Issue 1). BSI.
- BS 1377-7. (1990). Methods of test for soils for civil engineering purposes - part 7 : shear strength tests (total stress). BSI.
- BS 5930. (2015). Code of practice for ground investigations (B. S. Institution (ed.); Issue 1). British Standards Institution.
- BS EN 197-1. (2011). Cement. Composition, specifications and conformity criteria for common cements (British Standards Association (ed.); Issue October 2015). British Standards Association.
- Bui, T. T., Bui, Q. B., Limam, A., & Maximilien, S. (2014). Failure of rammed earth walls: From observations to quantifications. Construction and Building Materials, 51, 295–302. https://doi.org/10.1016/j.conbuildmat.2013.10.053
- Cabalar, A. F., & Canakci, H. (2011). Direct shear tests on sand treated with xanthan gum. Proceedings of the Institution of Civil Engineers – Ground Improvement, 164(2), 57–64. https://doi.org/10.1680/grim.800041
- Casas, J. A., Mohedano, A. F., & García-Ochoa, F. (2000). Viscosity of guar gum and xanthan/guar gum mixture solutions. Journal of the Science of Food and Agriculture, 80(12), 1722–1727. https://doi.org/10.1002/1097-0010(20000915)80:12%3C1722::AID-JSFA708%3E3.0.CO;2-X
- Chang, I., Im, J., Prasidhi, A. K., & Cho, G. C. (2015, January). Effects of xanthan gum biopolymer on soil strengthening. Construction and Building Materials, 74, 65–72. https://doi.org/10.1016/j.conbuildmat.2014.10.026
- Chang, I., Kwon, Y. M., Im, J., & Cho, G. C. (2019). Soil consistency and interparticle characteristics of xanthan gum biopolymer–containing soils with pore-fluid variation. Canadian Geotechnical Journal, 56(8), 1206–1213. https://doi.org/10.1139/cgj-2018-0254
- Chang, I., Prasidhi, A. K., Im, J., & Cho, G.-C. (2015). Soil strengthening using thermo-gelation biopolymers. Construction and Building Materials, 77, 430–438. https://doi.org/10.1016/j.conbuildmat.2014.12.116
- Chen, R. (2014). Bio stabilisation for geopolymer enchancement and mine tailings dust control. The University of Arizona. http://hdl.handle.net/10150/319999
- Chen, R., Zhang, L., Budhu, M., Latifi, N., Horpibulsuk, S., Meehan, C. L., Majid, M. Z. A., Rashid, A. S. A., Chang, I., Prasidhi, A. K., Cho, G. C., Desert, S., Qureshi, M. U., Chang, I., Al-sadarani, K., Paper, C., Bessaih, N., Al, K., Katzbauer, B., … Cho, G. C. (2016). Applications of biopolymers and other biotechnological products in building materials. Geoderma, 139(1), 39–47. https://doi.org/10.1007/s00253-004-1714-3
- Chudzikowski, R. J. (1971). Guar gum and its applications. Journal of the Society of Cosmetic Chemists, 22(1), 43–60. https://doi.org/10.1016/j.ijbiomac.2016.04.001
- Ding, X., Xu, G., Liu, W. V., Yang, L., & Albijanic, B. (2019). Effect of polymer stabilizers’ viscosity on red sand structure strength and dust pollution resistance. Powder Technology, 352, 117–125. https://doi.org/10.1016/j.powtec.2019.04.046
- Fischer, C. C., Navarrete, R. C., Constien, V. G., Coffey, M. D., & Asadi, M. (2001). Novel application of synergistic Guar/non-acetylated xanthan gum mixtures in hydraulic fracturing. SPE International Symposium Polymer Oilfield Chemistry, 527–538. https://doi.org/10.2118/65037-MS
- Frencham, G. J. (1982). The performance of earth buildings. Deakin University.
- Garcıa-Ochoa, F., Santos, V. E., Casas, J. A., & Gomez, E. (2000). Xanthan gum: Production, recovery, and properties. Biotechnology Advances, 18(7), 549–579. https://doi.org/10.1016/s0734-9750(00)00050-1
- Gresta, F., De Luca, A. I., Strano, A., Falcone, G., Santonoceto, C., Anastasi, U., & Gulisano, G. (2014). Economic and environmental sustainability analysis of guar (Cyamopsis tetragonoloba L.) farming process in a Mediterranean area: Two case studies. Italian Journal of Agronomy, 9(1), 20. https://doi.org/10.4081/ija.2014.565
- Gulrez, S., Al-Assaf, S., & Phillips, G. (2011). Hydrogels: Methods of preparation, characterisation and applications. In A. Carpi (Eds.), Progress in molecular and environmental bioengineering—from analysis and modeling to technology applications (pp. 117–150). Intech. https://doi.org/10.5772/57353
- Heathcote, K. A. (1995). Durability of earthwall buildings. Construction and Building Materials, 9(3), 185–189. https://doi.org/10.1016/0950-0618(95)00035-E
- Houben H., and Guillaud H (1994). Earth construction. A comprehensive guide. distributeur Craterre-Eag.
- Katzbauer, B. (1998). Properties and applications of xanthan gum. Polymer Degradation and Stability, 59(1–3), 81–84. https://doi.org/10.1016/S0141-3910(97)00180-8
- Kerali, A. G. (2001). Durability of compressed and cement-stabilised building blocks September 357. http://www6.zetatalk.com/docs/Bricks/Durability_Of_Compressed_And_Cement-Stabilised_Building_Blocks_2001.pdf
- Khatami, H. R., & O’Kelly, B. C. (2013). Improving mechanical properties of sand using biopolymers. Journal of Geotechnical and Geoenvironmental Engineering, 139(August), 1402–1406. https://doi.org/10.1061/(ASCE)GT.1943-5606.0000861
- Latifi, N., Horpibulsuk, S., Meehan, C. L., Abd Majid, M. Z., Tahir, M. M., & Mohamad, E. T. (2017). Improvement of problematic soils with biopolymer – An environmentally friendly soil stabilizer. Journal of Materials in Civil Engineering, 29(2), 04016204. https://doi.org/10.1061/(ASCE)MT.1943-5533.0001706
- Lax, C. (2010). Life Cycle Assessment of rammed earth. University of Bath.
- Krishna Leela J., & Sharma, G. (2000). Studies on xanthan production from Xanthomonas campestris. Bioprocess Engineering, 23(6), 687–689. https://doi.org/10.1007/s004499900054
- Lo, Y.-M., Yang, S.-T., & Min, D. B. (1997). Ultrafiltration of xanthan gum fermentation broth: Process and economic analyses. Journal of Food Engineering, 31(2), 219–236. https://doi.org/10.1016/S0260-8774(96)00068-4
- Maskell, D., Heath, A., & Walker, P. (2014). Comparing the environmental impact of stabilisers for unfired earth construction. Key Engineering Materials, 600(1), 132–143. https://doi.org/10.2966/scrip
- Mendonça, A. C. S. (2020). Use of microbial biopolymer to decrease soil permeability by bioclogging. University of Coimbra.
- Mudgil, D., Barak, S., & Khatkar, B. S. (2014). Guar gum: Processing, properties and food applications – A review. Journal of Food Science and Technology, 51(3), 409–418. https://doi.org/10.1007/s13197-011-0522-x
- Muguda, S., Booth, S. J., Hughes, P. N., Augarde, C. E., Perlot, C., Bruno, A. W., & Gallipoli, D. (2017). Mechanical properties of biopolymer-stabilised soil-based construction materials. Géotechnique Letters, 7(4), 309–314. https://doi.org/10.1680/jgele.17.00081
- Mwamufiya, I. M. (1998). Phase behavior and viscoelastic properties of high and low molecular weight guar mixtures with xanthan. Masters Thesis. Princeton University. http://arks.princeton.edu/ark:/88435/dsp013b5919057
- Nugent, R. A., Zhang, G., & Gambrell, R. P. (2009). Effect of exopolymers on the liquid limit of clays and its Engineering implications. Transportation Research Record: Journal of the Transportation Research Board, 2101(1), 34–43. https://doi.org/10.3141/2101-05
- NZS 4298. (1998) Materials and workmanship for earth buildings [Building Code Compliance Document E2 (AS2)] In New Zealand Technical Committee Standards New Zealand.
- Olivier, J. G. J., & Peters, J. A. H. W. (2020). Trends in global CO2 and total greenhouse gas emissions: Report 2019 PBL Netherlands Environmental Assessment Agency 2020(February): 70. www.pbl.nl/en
- Olivier, M., & Mesbah, A. (1987). Influence of different parameters on the resistance of earth, used as a building material. Mud Architecture November.
- Qureshi, M. U., Chang, I., & Al-Sadarani, K. (2017). Strength and durability characteristics of biopolymer-treated desert sand. Geomechanics and Engineering, 12(5), 785–801. https://doi.org/10.12989/gae.2017.12.5.785
- Reddy, J. J., & Varaprasad, B. J. S. (2021). Long-term and durability properties of xanthan gum treated dispersive soils – An eco-friendly material. Materials Today: Proceedings, 44, 309–314. https://doi.org/10.1016/j.matpr.2020.09.472
- Shi, Q., Qin, B., Bi, Q., & Qu, B. (2018). Fly ash suspensions stabilized by hydroxypropyl guar gum and xanthan gum for retarding spontaneous combustion of coal. Combustion Science and Technology, 190(12), 2097–2110. https://doi.org/10.1080/00102202.2018.1491845
- Soldo, A., Miletić, M., & Auad, M. L. (2020). Biopolymers as a sustainable solution for the enhancement of soil mechanical properties. Scientific Reports, 10(1), 267. https://doi.org/10.1038/s41598-019-57135-x
- Stirling, R. A., Hughes, P., Davie, C. T., & Glendinning, S. (2015). Tensile behaviour of unsaturated compacted clay soils – A direct assessment method. Applied Clay Science, 112-113, 123–133. https://doi.org/10.1016/j.clay.2015.04.011
- Ullah, F., Othman, M. B. H., Javed, F., Ahmad, Z., & Akil, H. M. (2015). Classification, processing and application of hydrogels: A review. Materials Science and Engineering: C, 57, 414–433. https://doi.org/10.1016/j.msec.2015.07.053
- Varaprasad, K., Raghavendra, G. M., Jayaramudu, T., Yallapu, M. M., & Sadiku, R. (2017). A mini review on hydrogels classification and recent developments in miscellaneous applications. Materials Science and Engineering: C, 79, 958–971. https://doi.org/10.1016/j.msec.2017.05.096
- Venkatarama Reddy, B. V., & Jagadish, K. S. (1989). Properties of soil cement blocks masonry. Masonry International, 3(2), 80–84. https://www.masonry.org.uk/downloads/properties-of-soil-cement-block-masonry/
- Xue, D., & Sethi, R. (2012). Viscoelastic gels of guar and xanthan gum mixtures provide long-term stabilization of iron micro- and nanoparticles. Journal of Nanoparticle Research, 14(11), 1239. https://doi.org/10.1007/s11051-012-1239-0.