Abstract
In order to study the basic physical phenomena underlying complex lipid transbilayer movement in biological membranes, we have measured the transmembrane diffusion of spin-labelled analogues of sphingolipids in phosphatidylcholine (PC) large unilamellar vesicles in the absence or presence of cholesterol, going from a fluid ( liquid disordered) ld, phase to a more viscous, liquid ordered (lo), phase. We have found cholesterol to reduce the transverse diffusion of glucosylceramide (GlcCer) and galactosylceramide (GalCer) in a concentration-dependent manner. However, surprisingly, we could neither detect any influence of cholesterol on the rapid flip-flop of ceramide nor on the flip-flop of dihydroceramide, for which the τ1/2 of flip-flop remains in the order of 1 minute at 20°C in the presence of cholesterol. As a consequence of rapid flip-flop of ceramide in both the lo and the ld phase, ceramide is likely to distribute between the two monolayers of a membrane, and could in principle partition into segregated domains in each side of the plasma membrane of eukaryotic cells.
Introduction
In pure lipid bilayers, the spontaneous movement of a lipid from one membrane leaflet to the other, i.e. the spontaneous transverse or transmembrane diffusion called ‘flip-flop’, is generally a slow phenomenon associated with long lipid lifetimes in each membrane leaflet. τ1/2 for phosphospholipids and sphingolipids ranges from several tens of minutes to several hours Citation[1–3]. However, certain lipids of biological importance have been reported to undergo fast flip-flop (τ < 1 min) at physiological temperatures. These generally correspond to lipids with a small polar moiety or a polar head-group that can be protonated. Among those are free fatty acids, cholesterol, diacylglycerides, phosphatidic acid and ceramides Citation[4–9]. In biological membranes, active and passive lipid transbilayer movement mediated by proteins occurs in addition to the spontaneous flip-flop taking place within a pure lipid bilayer. As a consequence, the steady-state asymmetrical distribution of lipids in biological membranes is the result of lipid traffic with many different time scales involved.
Lipid movement within a bilayer highly depends on the physical state of the bilayer phase. Lateral diffusion, as well as the flip-flop rate, are decreased when lipids are embedded in a highly ordered bilayer, corresponding to a gel or a condensed phase enriched in cholesterol Citation[10]. In the last years, much attention was paid to the hypothesis of the liquid ordered phase (lo) coexisting with fluid, liquid disordered (ld) domains in cell membranes, hence, forming functional lateral domains (or rafts) Citation[11], Citation[12]. In model membranes, a lo phase is formed by mixing an unsaturated lipid (principal constituent of the ld phase) with high amounts of a saturated lipid and/or cholesterol at suitable proportions. Phase diagrams have been constructed for different temperatures and different proportions of such lipids Citation[13], Citation[14]. Although the lateral diffusion of lipids is still significant in the lo phase, it is lower in the presence of cholesterol than in a fluid ld phase depleted of cholesterol Citation[15], Citation[16]. A phase modification from fluid ld to ordered lo phase triggered by a decrease in temperature or by an increase of cholesterol content can be expected to modify the transverse diffusion as well, although cholesterol molecules themselves (at least cholesterol analogues) were shown to experience a fast flip-flop in lipid bilayers Citation[6], Citation[17].
In general, the content of cholesterol in eukaryotic cellular membranes increases from the organelle membranes to the plasma membrane: The cholesterol-to-lipid molar ratio in the ER is small compared to that of the Golgi membrane, and even more so compared to the plasma membrane Citation[18]. Cholesterol reduces membrane permeability to ions and modifies the physical properties of cell membranes by being in particular responsible for the formation of rafts Citation[19]. Some sphingolipid precursors are synthesized in the monolayer opposite to the final product Citation[20]. This raises the question whether sphingolipid synthesis and trafficking could be controlled by a cholesterol-regulated spontaneous transmembrane diffusion in conjunction with other translocation mechanisms involving flippases Citation[21]. In a previous publication, we have shown that non-labelled ceramide rapidly flips between the two monolayers of a fluid lipid bilayer, as well as in the plasma membrane of erythrocytes Citation[7]. The rapid flip flop of ceramide was determined by us using shape change of giant vesicles and was also measured independently by other techniques in other laboratories Citation[8], and more recently by Citation[9]. We will show in this article that the flip rate of ceramide appears to be independent of cholesterol presence, but for other sphingolipids cholesterol reduces considerably the transmembrane diffusion and therefore can be useful to stabilise a lipid asymmetry.
In the present paper, we measured the spontaneous transbilayer movement of spin-labelled sphingolipid analogues in large unilamellar vesicles (LUVs), a well-controlled physical model, commonly used for measuring lipid transbilayer movement in membranes. Variation of LUVs’ lipid composition by modulation of cholesterol and sphingomyelin content permitted us to generate liquid disordered and liquid ordered phases, respectively. We measured the flip-flop of spin-labelled analogues of several sphingolipids: glucosylceramide (GlcCer), galactosylceramide (GalCer), lactosylceramide (LacCer), ceramide (Cer), dihydroceramide (dihyCer) and sphingomyelin (SM) (see ). Experiments were performed at 20 and 37°C, and revealed a broad spectrum of flip-flop half-times within the sphingolipid class, as well as great differences between the effect of the bilayer physical phase. A remarkable exception was the transmembrane diffusion of ceramide and of dihydocermide, which appeared to be independent of the lipid phase.
Box 1. Chemical structure of the spin labelled analogues of sphingolipids with a short sn-2 chain: Spin-labelled ceramide (SL-Cer); Spin-labelled Galactosylceramide (SL-GalCer); Spin-labelled Glucosylceramide (SL-GlcCer); Spin-labelled Lactosylceramide (SL-LacCer); Spin-labelled Sphingomyelin (SL-SM); Spin-labelled dihydroceramide (SL-dihyCer).
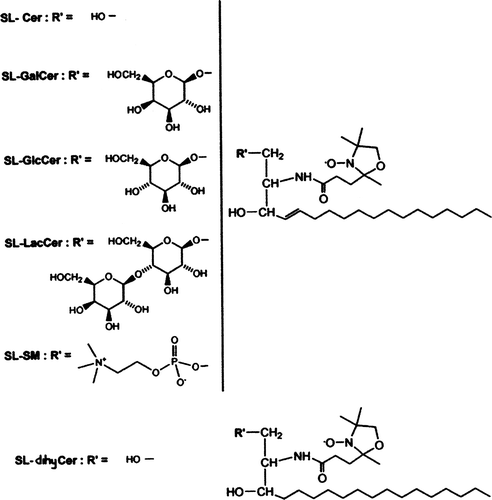
Indeed while the spontaneous transverse diffusion of monohexosylsphingolipids was notably decreased by the presence of cholesterol and almost stopped in the lo phase; surprisingly we found that the transverse diffusion of spin-labelled analogues of ceramide or of dihydroceramide are almost identical in the lo and ld phases, with a τ1/2, which remained in the order of 1 min at 20°C. If ceramides is an essential component of rafts Citation[22], the rapid transmembrane diffusion of ceramides in the liquid ordered phase has implications in relation with the existence of lateral domains in the eukaryotic plasma membrane.
Materials and methods
Materials
Ascorbic acid, bovine serum albumin (BSA), cholesterol, glucose, Hepes, sucrose, phospholipase C and reagents for lipid synthesis were purchased from Sigma-Aldrich. Potassium hexacyanoferrate (III) and NaCl were from Merck. KCl and MgSO4 were obtained from Prolabo (Paris, France) and Tris-HCl from Research Organics. egg-PC, POPC, cholesterol and brain SM were bought from Avanti Polar Lipids (Alabaster, AL, USA), Polycarbonate filters from Whatman (Maidstone, UK). Solvents were from SDS. Dihydrosphingosine was bought from Sigma (USA)
Spin-labelled lipids synthesis
Chemical structures of spin-labelled lipids employed in the present investigation are shown in . Spin-labelled (0,2) [1-palmitoyl-2-(4-doxylpentanoyl] GlcCer, GalCer, LacCer Citation[23], SM Citation[24] and Cer Citation[7] were synthesized in our laboratory as described previously. Spin-labelled (0,2) [1-palmitoyl-2-(4-doxylpentanoyl] SL-dihyCer, was synthesized by grafting 4-doxylpentanoic acid acid, on the amine function of the commercial dihydrosphingosine (see Materials) according to the method already reported Citation[7], yielding the expected spin labelled (0,2)-dihydroceramide. Spin-labelled dihydroceramide was purified on silica gel with gradient elution (chloroform:methanol: 100:0; 99:1; 98:2; 97:3; and 95:5) yielding 80% of the pure expected product. Dihydroceramide was identified by comparing the rf (∼ 0.75) on TLC (eluant:chloroform:methanol:water, 80:20:2.7) with that of the dehydrogenated homologous. Paramagnetic properties of the SL-(0,2)-dihydroceramide were checked by ESR spectroscopy and no further characterization was carried out.
Vesicle preparation
LUVs were prepared by adding buffer A (1 mM MgSO4, 250 mM sucrose, 50 mM Tris-HCl, pH 7.3) to a film of dried lipid (final concentration 4 mM), vortexing and four freeze-thawing cycles using liquid nitrogen, followed by repeated, sequential extrusion through 0.4 µm, 0.2 µm and 0.1 µm pore size polycarbonate filters using a Swinney 13 mm filter syringe system by Millipore (Bedford, MA, USA). LUVs in a ld phase were prepared with egg-PC, containing a mixture of chain lengths, resembling lipid mixtures in biological membranes or POPC. In order to detect the effect of the cholesterol content on the spontaneous transbilayer movement, 25 or 44% mol of cholesterol was incorporated in egg-PC LUVs. For the lo phase, the lipid composition of LUVs was POPC/SM/Chol in a molar ratio of 20/20/60 mol.
Measurement of SL-lipid transbilayer movement in LUVs
SL-lipid transbilayer inward movement in LUVs was determined as described by Citation[23]. Briefly, LUVs were added to SL-lipids (final concentration 20 µM, that is, a molar fraction of 0.005 of total lipid) in buffer A and incubated on ice for 10 min to allow for integration of the analogue into the outer membrane leaflet. LUVs were transferred to 20°C. At indicated time points, 50 µl aliquots were added to 30 µl ice-cold BSA (final concentration 5%) in buffer A and incubated on ice for 30 sec before addition of 5 µl ascorbic acid (final concentration 10 mM). The amount of SL-lipid inaccessible to reduction was immediately measured on a Bruker (Wissembourg, France) ER 200D-SRC Electron Spin Resonance (ESR) Spectrometer.
For the determination of SL-lipid transbilayer outward movement, symmetrically labelled LUVs were prepared from dry lipid films containing lipids (8 mM) and SL-lipids (final concentration in solution 5–10 µM for each lipid species). LUVs were added to a buffer containing BSA (4.4% in weight) and incubated as described above to remove the spin-labelled lipids from the outer leaflet. After addition of ascorbic acid (10 mM final concentration), the nitroxides exposed on the outer leaflet were subsequently chemically reduced. The remaining signal inaccessible to reduction was determined in the same sample at 20°C or 37°C (indicated in the text when necessary) at indicated time points.
ESR signal analysis
SL-lipid was quantified from the full height of the midfield-peak. In the presence of ascorbic acid, the full height of the low field-peak was determined in LUV preparations using EPR3 Stelar (Mede, Italy) software.
Results
Characterization of the acyl chain order of liposomes
The analysis of the extreme splittings (A) or the line widths (B, 1C) of ESR spectra permit the evaluation of the mobility of a probe, attached to a lipid chain either near the polar head group or near the methyl terminal of the alkyl chains. In order to characterize the acyl chain order of lipid vesicles, we recorded ESR spectra at 20°C of three different spin labelled PC molecules (SL-PCCitation[10], Citation[3], SL-PCCitation[1], Citation[14], SL-PCCitation[5], Citation[10]) which are characterized by the position of the probe on the sn-2 chain. The different analogues were incorporated in LUVs with diverse lipid compositions, corresponding to different lipid phases. Tested lipid compositions ranged from pure (egg PC or POPC) (fluid or liquid disordered phase, ld) to POPC/SM/Chol (liquid ordered phase, lo), passing through intermediated compositions where lo and ld phases coexisted (egg-PC with 25 or 44% mol of cholesterol). When passing from a fluid ld phase to an lo phase by increasing the content of cholesterol, we found gradual differences in line shapes. SL-PC is expected to be inserted into the ld phase. Correspondingly, differences in ESR line shapes were not remarkable when the two lipid phases coexisted.
Figure 1. ESR spectra for different spin labelled phosphatidylcholines embedded in a ld membrane (dashed line) or in lo membrane (solid line). (A) SL-PC (10,3) (the probe is attached near the polar head group). (B) SL-PC (5,10) (the probe is attached at carbon 11 of the sn2 chain) and (C) SL-PC (1,14) (the probe is attached near the methyl terminal of the alkyl chains at carbon 15). The line broadening, indicative of a decrease on molecular motion, was systematically observed for all probes when inserted in more ordered phases. Differences in spectra line shape depends on the position of the nitroxide group on the acyl chain.
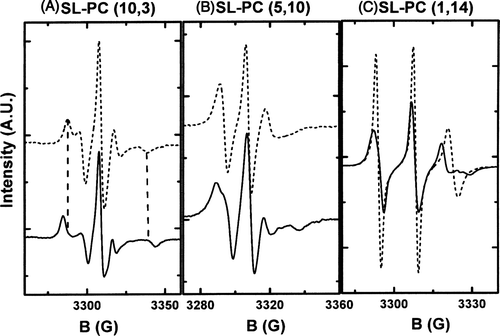
For clarity, only shows the spectra of the three different SL-PC analogues when inserted in the ld phase (dashed line) or in the lo phase (continuous line). For all probe molecules, a broadening of the line spectrum was observed in the lo phase versus the ld phase at the same temperature revealing a clear increase in chain order. reveals that a lipid mixture which corresponds to the composition of the liquid ordered phase (lo) is indeed more visquous than pure egg-PC or POPC liposomes at the same temperature (phase ld). The ESR spectra in A show highly deviating line shapes for the two phases. ESR spectra obtained with different spin-labelled fatty acids suggest a homogeneous ordered phase. Thus, a priori, the fraction of fluid ld phase that could coexist in the lo sample must be very small. Additionally, if the probes were clustered in a small fraction of the vesicle surface, electron spin-electron spin interactions would broaden the ESR spectra.
Cholesterol reduces the spontaneous transbilayer movement of monohexosylsphingolipids in a concentration-dependent manner
In a first series of experiments, the influence of cholesterol on lipid translocation was investigated for different sphingolipid analogues. The inward spontaneous transbilayer movement of SL-GlcCer (black symbols) and SL-GalCer (open symbols) was assessed in egg-PC LUVs in the absence or presence cholesterol (from 0–44 mol%) at 20°C (A). The coexistence of two different lipid phases was confirmed by ESR spectra line shapes. The ratio of labelled lipid to egg-PC was typically 0.01 (mol ratio) to avoid spin-spin interactions between spin-labelled lipids or possible artefacts due to a high concentration of molecules with one short chain. Experimental data points obtained from the back-exchange method (see Materials and methods) were fitted to an exponential growth function, f = a(1−e−t/τ)where τ is related with the spontaneous transbilayer halftime. Halftimes were calculated from this equation.
Figure 2. (A) Inward movement of SL-GlcCer (solid symbols) and SL-GalCer (open symbols) in egg-PC LUVs containing 0% (squares), 25% (circles) or 44% (triangles) cholesterol at 20°C. (B) Inward movement of SL-GalCer in (POPC/SM/Chol; 20/20/60 mol) LUVs (lo phase, open rhombus) at 20°C. For comparison, data from A corresponding to the inward transport of SL-GalCer in more fluid phases, were also replotted (squares and triangles). After 10 min incubation of SL-lipids with LUVs on ice, tubes were transferred to 20°C. At indicated time points, samples were mixed with ice-cold BSA (final concentration 5%) and incubated on ice for 30 sec before addition of ascorbic acid (final concentration 10 mM). SL-lipid inaccessible to reduction was quantified by ESR. Results are means +/− SEM of at least n=3 independent experiments.
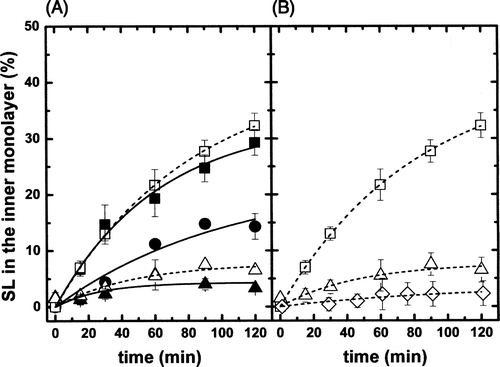
The fact that internalization curves of SL-GlcCer and SL-GalCer do almost superimpose reflects the structural similarity of GalCer and GlcCer (). In agreement with earlier results Citation[23], SL-monohexosylsphingolipid transbilayer movement was found to be of the order of ∼ 40 min in the continuous ld phase (0% of cholesterol) at 20°C. τ1/2was increased to ∼ 270 min in vesicles containing 44% of cholesterol. The flip-flop half time in LUVs possessing an intermediate cholesterol concentration (25% mol) was ∼ 90 min.
In the continuous lo phase (POPC/SM/Chol, 20/20/60 mol), the inward spontaneous flip-flop of SL-GalCer was quasi inexistent (B, open rhombus). For comparison, the kinetics of probe internalization in the ld phase (egg-PC, open square) and in the lo+ld phase (egg-PC/Chol, 56/44 mol, open triangle) are also shown. The τ1/2 for SL-GalCer calculated from fitting of the experimental data was increased to ∼ 3000 min in the lo phase. This numerical value is an approximation because of the very small values of the internalization measurement which prevents a rigorous determination, but it is a clear indication of a practically total immobilization of SL-GalCer within the lo phase, and of the high interaction of SL-GalCer with cholesterol and/or SM.
A different behaviour was found for SL-LacCer and SL-SM. A and 3B show the inward diffusion of SL-SM and SL-LacCer at 20°C in egg-PC LUVs (black symbols, ld phase) and in a lo phase (open symbols). The influence of cholesterol on the spontaneous flip-flop of these two molecules was difficult to detect as the transmembrane diffusion without cholesterol is already very slow for these lipids Citation[23]. For both probes, halftimes were one two- to threefold greater in the lo phase than in the ld phase (shifting from ∼ 800 min and ∼ 400 min to ∼ 1500 min and ∼1300 min, for SL-SM and SL-LacCer, respectively).
Figure 3. (A) Inward movement of SL-SM in POPC LUVs (ld phase, solid squares) and in (POPC/SM/Chol; 20/20/60 mol) LUVs (lo phase, open squares). Temperature was 20°C. (B) Inward movement of SL-LacCer in POPC LUVs (ld phase, solid squares) and in (POPC/SM/Chol; 20/20/60 mol) LUVs (lo phase, open squares). Temperature: 20°C. Results are means +/- SEM of two duplicated independent experiments.
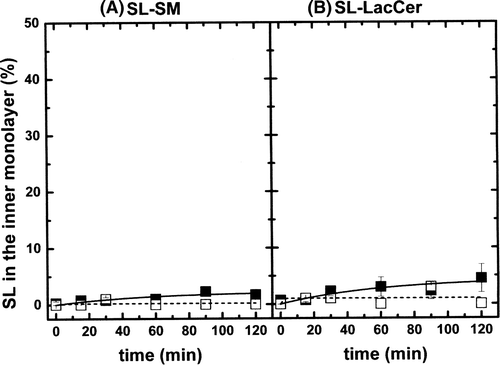
Increase of temperature accelerates the spontaneous transbilayer movement of monohexosphingolipids
In order to evaluate the influence of temperature on the flip-flop rates of SL-GalCer and SL-GlcCer, we have measured the inward translocation at a temperature of 37°C, in egg-PC LUVs with or without 44% mol cholesterol. Translocation curves plotted in A show that SL-GalCer and SL-GlcCer exhibit similar kinetic behaviour, as reported in . In the absence of cholesterol (ld phase), flip-flop was strongly accelerated when compared to values obtained at 20°C, yielding τ1/2 of 1.5 min. When the spin labelled probes were embedded in egg-PC LUVs with a high amount of cholesterol (44% mol), the half-time was also decreased almost thirty-fold, changing from 270–10 min.
Figure 4. (A) Inward movement of SL-GlcCer (solid symbols) and SL-GalCer (open symbols) in egg-PC LUVs containing 0% (squares) or 44% (triangles) cholesterol at 37°C. For comparison, data from A corresponding to the inward teransport of SL-GlcCer at 20°C were also replotted (dashed-dot lines). (B) Inward movement of SL-LacCer in POPC LUVs (ld phase, solid squares) and in (POPC/SM/Chol; 20/20/60 mol) LUVs (lo phase, open squares) at 37°C.
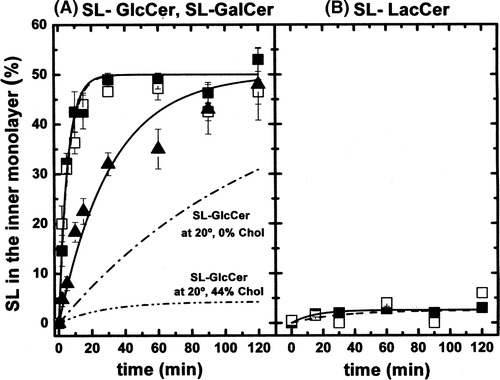
When the transbilayer movement of SL-LacCer was assayed in both ld and lo phases (B) at 37°C, slow transbilayer movement was obtained for the dihexosylsphingolipid SL-LacCer in both lipid phases, with half-times of 600 and 400 min for the ld and lo phase, respectively. Taking into in account the uncertainty of measurements at incubation times short in relation to translocation rates, half-times found here for molecules with a slow transbilayer movement are rather similar to those found at 20°C. Once again, these results show the low ability of LacCer to undergo spontaneous transbilayer movement.
Flip-flop of SL-Cer and SL-dihyCer is rapid in the lo phase
We found previously that transmembrane diffusion of a spin-label ceramide is of the order of 1 min at 20°C in the fluid phase in Large Unilamellar Vesicles (LUVs) Citation[7]. Similar transbilayer rates were obtained with spin-labelled ceramide in cholestrol containing LUVs. The outward transmembrane movement was measured actually for two different ceramide analogues: (i) A spin-labelled analogue of ceramide, and (ii) a spin-labelled analogue of dihydroceramide (see ). The spin-labels were incorporated into LUVs in the lo phase (POPC/SM/cholesterol (20/20/60% mol). Surprisingly, the transbilayer movement of ceramide, shown in , was almost as fast in the lo phase than in a fluid ld phase (τ1/2∼1,1 min). Furthermore, we did not detect any meaningful difference between ceramide spin-labelled analogue and dihydroceramide spin-labelled analogue. The same values were obtained for flop and flip rates (data not shown).For the sake of clarity, all halftimes τ1/2 of flip-flop measured for lipid analogues used here are collected in .
Figure 5. (A) Outward movement of SL-Cer in POPC LUVs (ld phase, solid squares) and in(POPC/SM/Chol; 20/20/60 mol) LUVs (lo phase, open squares). Temperature: 20°C. (B) Outward movement of SL-dihyCer in POPC LUVs (ld phase, solid squares) and in (POPC/SM/Chol; 20/20/60 mol) LUVs (lo phase, open squares). Temperature: 20°C.
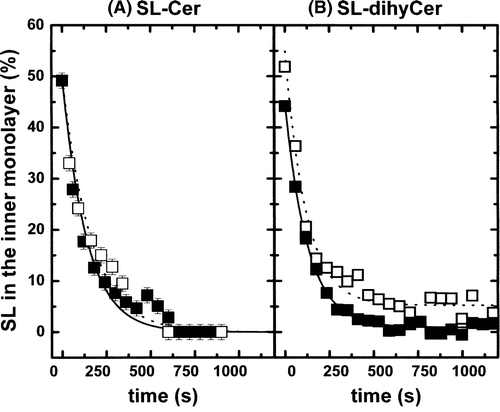
Table I. Halftimes τ1/2 (in minutes) of flip-flop for sphingolipids analogues in different lipid phases at 20 and 37°C.
Discussion
Validity of spin-label probes
In this article, spin-labeled sphingolipids with one short-chain bearing a nitroxide ring were used as analogues of endogenous lipids to measure the spontaneous transmembrane diffusion of lipids. The short fatty acid chain increases the water-solubility of a lipid and consequently facilitates its introduction in a membrane. In the present case, these analogues were chosen mainly because their transmembrane orientation in a bilayer can be easily assessed: the probed lipid can either be extracted selectively from the external leaflet of a unilamellar vesicle with bovine serum albumine, and the fraction of labeled lipids present in the outer leaflet be determined by ESR spectroscopy Citation[25], or the transmembrane lipid asymmetry can be determined in situ by chemical reduction of the probes exposed on the outer leaflet. Actually, the short chain is probably a bigger perturbation than the nitroxide ring. The nitroxide ring is relatively small and its steric hindrance minimal, less important than most fluorescent probes Citation[26]. On the other hand, short chain lipids probably behave slightly differently from long chain lipids. The spontaneous diffusion of ceramide with a short C6 chain was shown to be significantly faster than that of the C16-Cer Citation[7]. Goñi and collaborators have shown that the short chain C2-Cer has very different properties than long chain ceramides and can destabilise a lipid bilayer Citation[27]. Methods suitable for the quantification of the transmembrane distribution of unlabeled lipids were originally based on exogenous phospholipase attack of the cell surface Citation[28]. The precision of this technique is limited and the in situ lipid degradation can cause artefacts. Recently, we have shown that the transmembrane distribution of lipids added to a giant liposome surface can be deduced from the shape of the liposomes without requiring labeled lipids Citation[7]. However, this technique is difficult to apply in the case of a mixture of different lipid phases. It has also been reported that LUVs have slightly different properties than GUV or true biological cell membrane because of the relatively low radius of curvature Citation[29]. For these reasons and also because this work was based on the use of lipid probes, the absolute values of τ1/2 may slightly differ from those in a biological cell membrane. Meanwhile, here we used a classical technique of investigation with spin-label lipids Citation[1], Citation[25] which has proven to give at least reliable information on comparative values of the flip-flop rates of different phospholipids Citation[30]. Nevertheless, we do not claim that values determined for one class of lipid probe is rigorously that of the corresponding endogenous lipid.
Short chain ceramides (C2, C6 and C12) have different properties than long chain ceramides. Thus, the spontaneous diffusion of ceramide with a short C6 chain was shown to be slightly faster than that of the C16-Cer Citation[7], short chain C2-Cer can destabilise a lipid bilayer Citation[27] and more recently it has been found that short ceramides can alter the physical properties of liquid ordered domains Citation[30]. Besides problems inherent to probes and to short chains, very fast flip–flop movements are difficult to determine by conventional ESR. One has to admit the limits of the ESR technique based on the use of aliquots removed periodically with less than 30 sec or 1 min intervals. Only stop flow ESR permits the precise measurement for τ1/2<1 min. Citation[31]. However, the results of many former studies have demonstrated that lipids with different structures, bearing one short chain with a nitroxide label, yield valuable information on the effect that the headgroup can have on phospholipids transbilayer movement. Citation[32–34].
In the following paragraphs we shall tentatively discuss the results obtained in this work in the context of the sphingolipid asymmetrical distribution in eukariotic lipid traffic, taking into account that the cholesterol content in eukaryotic cells gradually goes from ∼ 0% mol in the ER to 30–40% mol in the plasma membrane.
Monohexosyl sphingolipids
Although GlcCer and GalCer constitute only minor components of most mammalian membranes Citation[35], their transbilayer mobility may affect physiological processes, namely the clear cholesterol concentration-dependent effect on the spontaneous transmembrane diffusion of SL-GalCer and SL-GlcCer. GlcCer, synthesized from Cer in the lumenal cis-Golgi, is itself the precursor of LacCer in the lumenal trans-Golgi Citation[36]. The remaining transmembrane diffusion rate for GlcCer in presence of a moderate concentration of cholesterol (as in the case of the Golgi) could lead one to speculate that GlcCer will be accessible to enzymes of LacCer synthesis. The final destination for both GlcCer and GalCer is the outer monolayer of the plasma membrane. GalCer was even found in the plasma membrane of cells in which a brefeldin A assay – stopping anterograde vesicular transport between the compartments of the Golgi and thus leading to a redistribution of the Golgi into the ER – had previously been performed Citation[37]. A monomeric transport to the plasma membrane is hence hypothesized Citation[38]. In this case, GalCer (and GlcCer) molecules would be found in the inner monolayer of the plasma membrane. The remaining spontaneous flip-flop of SL-GalCer (SL-GlcCer) measured at high concentrations of cholesterol (10 min at 44% cholesterol and at 37°C, see A) should re-equilibrate the distribution in both monolayers, even for those molecules originating from vesicular traffic. However, GalCer and GlcCer are only found in the outer monolayer of the plasma membrane. Thus, the asymmetrical distribution of these lipids is likely to be maintained by a flippase Citation[36], Citation[39], Citation[40]. MDR1 (P-gP) has been proposed to be a good candidate for this Citation[8], Citation[21].
Lactosylceramide and sphingomyelin
A reduction of the transbilayer diffusion of SM and LacCer by cholesterol was not detected here, probably due to the very slow diffusion even in the absence of cholesterol. In fact, the time scale chosen did not allow the detection of differences that may be visible only after several hours. In previous experiments, cholesterol was shown to reduce the transbilayer movement of SL-PC in erythrocytes observed up to 18 h Citation[32]. Both LacCer and SM are transferred from the inner leaflet of the trans-Golgi, where they are synthesized Citation[41], Citation[42], to the outer leaflet of the plasma membrane by vesicular transport Citation[43], Citation[44]. The extremely slow spontaneous transverse diffusion measured here ensures the passage from the lumenal Golgi to the extracellular leaflet of the plasma membrane, two sides of identical topology.
SL-ceramide and SL dihydrocermide
It was proposed already Citation[7] that the very fast transmembrane diffusion of ceramide, independently of cholesterol concentration, facilitates its location in both sides of membranes where ceramide acts as a precursor of other sphingolipids: GalCer synthesis in the inner leaflet of ER Citation[45]; GlcCer in the outer leaflet of the cis-Golgi Citation[43], Citation[46], Citation[47] and the SM synthesis in the inner monolayer of the trans-Golgi Citation[48]. As pointed out above we have not been able to evidence convincing differences between SL-ceramide and SL-dihydrocermide.
Due to their lack of effective polar headgroup, both spin-labelled lipids flip rapidly in the ld phase (A). More surprisingly, and contrarily to the behaviour of the monohexosylsphingolipids SL-GalCer and SL-GlcCer, the rapid transbilayer movement was not affected when the ceramide analogues were embedded in the more ordered phase lo, with virtually identical values for SL-Cer and SL-dihyCer (B). In order to rule out the possibility of artefacts, we have regarded several scenarios. A first hypothesis to explain the rapid transmembrane diffusion of a lipid in the lo phase could be a lipid mixture deviating from the desired composition, in which a small percentage of segregated fluid domains could form. Ceramide might prefer such an environment, cluster and flip rapidly in the ld phase.
An alternative possibility, rather similar in effect, would be the formation of microdomains separated by ‘defects’ lines between small homogenous microdomains in the lo phase. Along these defects, the actual chain order state could be very different from the bulk lo phase. However, such hypotheses would be difficult to reconcile with the slow flip-flop of Gal-Cer. Indeed when it is incorporated in the same lipid preparation SL-Gal-Cer flipped at a much slower rate than in an ld phase. The flip rates of spin-labelled lyso-PC, SL-PC and SL-PE, which all bear a nitroxide, are very long (several hours), even in a fluid membrane environment Citation[32]. It can also be ruled out that, because of the ordered character of the lo phase, SL-Cer and SL-dihyCer were excluded from the bilayer and maintained in the aqueous phase where the nitroxide would be rapidly reduced by ascorbate. This would then also occur in control experiments with spin-labelled PC, for which no rapid flip-flop of spin-labelled PC investigated by this technique has ever been reported in an ordered phase. Thus, the rapid transmembrane diffusion of SL-Cer in the ld or lo phases cannot be easily explained by a probe artefact or an error in the detection assay. A last possibility reported by Goñi's laboratory consists in the ability of lipids prone to form non-lamellar structures, such as ceramide, to destabilize the lipid bilayer Citation[27], Citation[49], Citation[50]. However, ceramides should then accelerate the transbilayer movement of other lipids in the same membrane. Yet, we found that the presence of natural ceramide in the lipid composition does not modify the flip-flop rate of SL-PC in the same LUVs Citation[7].
We propose that this lipid scrambling could be explained by a local destabilization of the lipid bilayer upon in situ conversion of sphingomyelin (SM) into ceramide or upon a high asymmetrical increase of ceramide concentration by external addition of molecules, both cases would generate a high lipid mismatch between the two monolayers Citation[50], Citation[51]. While the rapid flip-flop of ceramide and of dihydroceramide analogues in the lipid ordered phase is not easy to explain, our experiments clearly indicate that the lipid headgroup is the bottle neck that prevents the lipids from flipping. It also confirms that the so-called liquid ordered lo phase is different from a condensed or crystalline phase since, in agreement with the data on lateral diffusion Citation[16], it allows hydrophobic molecules to move within.
The rapid transmembrane diffusion of SL-Cer and SL-dihyCer in the lo phase may have important biological consequences. Ceramide, is formed in vivo by hydrolysis of sphingomyelin in the outer monolayer of the plasma membrane and was reported to accumulate in rafts Citation[22], Citation[52]. Because of its propensity to flip rapidly from one monolayer to the other in a bilayer, ceramide might distribute between the two monolayers of the plasma membrane and could form domains in each side of the membrane. This remark is to be taken into account in discussions about lateral domains in the plasma membrane of eukaryotic cells Citation[9].
Conclusions
The present article reports several large amplitude effects indicating a clear difference in transmembrane diffusion between ceramides and the other sphingolipids. We believe that the results are indications of significant phenomena.
Firstly, the size of the polar headgroup is a major determinant of spontaneous transbilayer movement of sphingolipids, as shown by our results obtained with spin-labelled lipid analogues in fluid phase (ld). Spontaneous transbilayer movement is very slow for SL-LacCer and SL-SM, which possess a large polar headgroup. Conversely, the absence of an effective polar headgroup facilitates the spontaneous transbilayer movement of ceramide and of dihydroceramide. The transbilayer diffusion of SL-Cer is at least 100 times faster than that of SL-SM or SL-LacCer. As an intermediate case, monohexosylsphingolipids with medium-sized headgroups have a τ1/2 values one order of magnitude larger than that of SL-Cer. These results also confirm previous measurements Citation[23], and additionally allow direct comparison between the different sphingolipid species investigated by the same methodology.
Secondly, the spontaneous transbilayer movement of a lipid, besides depending on its own chemical structure, is affected by the physical state of its environment. We found that the spontaneous transbilayer movement of monohexosylsphingolipids decreased drastically with an increasing cholesterol content. In organelle membranes, where the cholesterol content is generally lower than in the plasma membrane, the transbilayer movement of various lipid analogues has been found to be relatively rapid Citation[23], Citation[34], Citation[53]. Even if this movement is largely due to active transport or facilitation of transbilayer movement by one or several proteins Citation[54], it would be conceivable to be favoured additionally by the low cholesterol content in these membranes. It can be hypothesized that the different concentrations of cholesterol along the trafficking route in eukaryotic cells control the asymmetrical distribution of sphingolipids during vesicular traffic by modulating their spontaneous transbilayer movement.
However, and finally, data obtained with SL-Cer and SL-dihyCer in a lo phase show that simple intuition can be misleading since we did not evidence any significant effect of cholesterol on the flip-flop of such molecules.
Acknowledgements
We thank R. de Nijls who carried out the initial experiments with spin-labels in lo and ld phases. The authors thank P. Hervé for her participation in the synthesis of spin-labelled lipids used in this work. We are grateful to Prof. A. Herrmann, Prof. S. Cribier and Dr N. Rodriguez for helpful discussions. This work was supported by grants from the Centre National de la Recherche Scientifique (UMR 7099), from the European Union-HPRNCT-2000-0077 (Research Training Network on ‘Sphingolipids synthesis and organization’), by a grant from the European Union (MRTN-CT2004-005330) ‘Phospholipid flippase’ and by the COST action D22 on Lipid-Protein Interactions. Declaration of interest: The authors report no conflicts of interest. The authors alone are responsible for the content and writing of the paper.
References
- Kornberg RD, McConnell HM. Inside-outside transitions of phospholipids in vesicle membranes. Biochemistry 1971; 10: 1111–1120
- Homan R, Pownall HJ. Transbilayer diffusion of phospholipids: dependence on headgroup structure and acyl chain length. Biochim Biophys Acta 1988; 938: 2155–2166
- Zachowski A. Phospholipids in animal and eukaryotic membranes: transverse asymmetry and movement. Biochem J 1993; 294: 1–14
- Kleinfeld AM, Chu P, Romero C. Transport of long-chain native fatty acids across lipid bilayer membranes indicates that transbilayer flip-flop is rate limiting. Biochemistry 1997; 36: 14146–14158
- Zeng Y, Han X, Schlesinger P, Gross RW. Nonesterified fatty acids induce transmembrane monovalent cation flux: host-guest interactions as determinants of fatty acid-induced ion transport. Biochemistry 1998; 37: 9497–9508
- Hamilton JA. Fast flip-flop of cholesterol and fatty acids in membranes: implications for membrane transport proteins. Curr Opin Lipidol 2003; 14: 263–271
- López-Montero I, Rodriguez N, Cribier S, Pohl A, Vélez M, Devaux PF. Rapid transbilayer movement of ceramides in phospholipid vesicles and erythrocytes. J Biol Chem 2005; 280: 25811–25819
- Eckford PD, Sharom FJ. The reconstituted P-glycoprotein multidrug transporter is a flippase for glucosylceramide and other simple glycosphingolipids. Biochem J 2005; 389: 517–526
- Mitsutake S, Igarashi Y. Transbilayer movement of ceramide in the plasma membrane of live cells. Biochem Biophys Res Commun 2007; 359: 622–627
- Wimley WC, Thompson TE. Exchange and flip-flop of dimyristoylphosphatidylcholine in liquid-crystalline, gel, and two-component, two-phase large unilamellar vesicles. Biochemistry 1990; 29: 1296–1303
- Simons K, Ikonen E. Functional rafts in cell membranes. Nature 1997; 387: 569–572
- Engelman DM. Membranes are more mosaic than fluid. Nature 2005; 438: 578–580
- de Almeida RF, Fedorov A, Prieto M. Sphingomyelin/phosphatidylcholine/cholesterol phase diagram: boundaries and composition of lipid rafts. Biophys J 2003; 85: 2406–2416
- Veatch SL, Keller SL. Seeing spots: complex phase behavior in simple membranes. Biochim Biophys Acta 2005; 1746: 172–185
- Devaux P, McConnell HM. Lateral diffusion in spin-labeled phosphatidylcholine multilayers. J Am Chem Soc 1972; 94: 4475–4481
- Kahya N, Scherfeld D, Bacia K, Poolman B, Schwille P. Probing lipid mobility of raft-exhibiting model membranes by fluorescence correlation spectroscopy. J Biol Chem 2003; 278: 28109–28115
- Müller P, Herrmann A. Rapid transbilayer movement of spin labeled steroids in human erythrocytes and in liposomes. Biophys J 2002; 82: 1418–1428
- Van Meer G. Lipid traffic in animal cells. Annu Rev Cell Biol 1989; 5: 247–275
- Simons K, Ikonen E. How cells handle cholesterol. Science 2000; 290: 1721–1726
- Van Meer G, Lisman Q. Sphingolipid transport: rafts and translocators. J Biol Chem 2002; 277: 25855–25858
- Van Helvoort A, Smith AJ, Sprong H, Fritsche I, Schinkel AH, Borst P, van Meer G. MDR1 P-glycoprotein is a lipid translocase of broad specificity, while MDR3 P-glycoprotein specifically translocates phosphatidylcholine. Cell 1996; 87: 507–517
- Megha, London E. 2004. Ceramide selectively displace cholesterol from ordered lipid domains. J Biol Chem 279:9997–10004
- Buton X, Herve P, Kubelt J, Tannert A, Burger KN, Fellmann P, Muller P, Herrmann A, Seigneuret M, Devaux PF. Transbilayer movement of monohexosylsphingolipids in endoplasmic reticulum and Golgi membranes. Biochemistry 2002; 41: 13106–13115
- Zachowski A, Fellman P, Devaux PF. Absence of transbilayer diffusion of spin-labelled sphingomyelin on human erythrocytes. Comparison with the diffusion of several spin-labelled glycerophospholipids. Biochim Biophys Acta 1985; 815: 510–514
- Fellmann P, Zachowski A, Devaux PF. Synthesis and use of spin-labelled lipids for studies of the transmembrane movement of phospholipids. Methods Mol Biol 1994; 27: 161–175
- Devaux PF, Fellmann P, Herve P. Investigation on lipid asymmetry using lipid probes: Comparison between spin-labelled lipids and fluorescent lipids. Chem Phys Lipids 2002; 116: 115–134
- Sot J, Goñi FM, Alonso A. Molecular associations and surface-active properties of short- and long-N-acyl chain ceramides. Biochim Biophys Acta 2005; 1711: 12–19
- Verkleij AJ, Zwaal RF, Roelofsen B, Comfurius P, Kastelijn D, van Deenen LL. The asymmetric distribution of phospholipids in the human red cell membrane. A combined study using phospholipases and freeze-etch electron microscopy. Biochim Biophys Acta 1973; 323: 178–193
- Traïkia M, Warschawski DE, Lambert O, Rigaud JL, Devaux PF. Asymmetrical membranes and surface tension. Biophys J 2002; 83: 1443–1454
- Chiantia S, Kahya N, Schwille P. Raft domain reorganization driven by short- and long-chain ceramide: a combained AFM and FCS study. Langmuir 2007; 23: 7659–7665
- Marx U, Lassmann G, Holzhutter HG, Wustner D, Muller P, Hohlig A, Kubelt J, Herrmann A. Rapid flip-flop of phospholipids in endoplasmic reticulum membranes studied by a stopped-flow approach. Biophys J 2000; 78: 2628–2640
- Seigneuret M, Devaux PF. ATP-dependent asymmetric distribution of spin-labelled phospholipids in the erythrocyte membrane: relation to shape changes. Proc. Natl Acad Sci USA 1984; 81: 3751–3755
- Morrot G, Herve P, Zachowski A, Fellmann P, Devaux PF. Aminophospholipid translocase of human erythrocytes: phospholipid substrate specificity and effect of cholesterol. Biochemistry 1989; 28: 3456–3462
- Zachowski A, Henry JP, Devaux PF. Control of transmembrane lipid asymmetry in chromaffin by an ATP-dependent protein. Nature 1989; 340: 75–76
- Holthuis JC, Pomorski T, Raggers RJ, Sprong H, Van Meer G. The organizing potential of sphingolipids in intracellular membrane transport. Physiol Rev 2001; 81: 1689–1723
- Lannert H, Gorgas K, Meissner I, Wieland FT, Jeckel D. Functional organization of the Golgi apparatus in glycosphingolipidbiosynthesis. Lactosylceramide and subsequent glycosphingolipids are formed in the lumen of the late Golgi. J Biol Chem 1998; 273: 2939–2946
- Kano F, Sako Y, Tagaya M, Yanagida T, Murata M. Reconstitution of brefeldin A-induced golgi tubulation and fusion with the endoplasmic reticulum in semi-intact chinese hamster ovary cells. Mol Biol Cell 2000; 11: 3073–3087
- Warnock DE, Lutz MS, Blackburn WA, Young WW, Jr, Baenziger JU. 1994. Transport of newly synthesized glucosylceramide to the plasma membrane by a non-Golgi pathway. Proc Natl Acad Sci USA 91:2708–2712.
- Burger KN, van der Bijl P, van Meer G. Topology of sphingolipid galactosyltransferases in ER and Golgi: transbilayer movement of monohexosyl sphingolipids is required for higher glycosphingolipids biosynthesis. J Cell Biol 1996; 133: 15–28
- Lala P, Ito S, Lingwood CA. Rotroviral transfection of Madin-Darby canine kidney cells with human MDR1 results in a major increase in globotriasylceramide and 10(5)- to 10(6)-fold increased cell sensitivity to verocytotoxin. Role of p-glycoprotein in glycolipid synthesis. J Biol Chem 2000; 275: 6246–6251
- Jeckel D, Karrenbauer A, Birk R, Schimdt RR, Wieland F. Sphingomyelin is synthesized in the cis Golgi. FEBS Lett 1990; 261: 155–157
- Futerman AH, Stieger B, Hubbard AL, Pagano RE. Sphingomyelin synthesis in the rat liver occurs predominantly at the cis and medial cisternae of the Golgi apparatus. J Biol Chem 1990; 265: 8650–8657
- Jeckel D, Karrenbauer A, Burger KN, van Meer G, Wieland F. Glucosylceramide is synthesized at the cytosolic surface of various Golgi subfractions. J Cell Biol 1992; 117: 259–267
- Kallen KJ, Allan D, Whatmore J, Quinn P. Synthesis of surface sphingomyelin in the plasma membrane recycling pathway of BHK cells. Biochim Biophys Acta 1994; 1191: 52–58
- Sadeghlar F, Sandhoff K, van Echten-Deckert G. Cell type specific localization of sphyngomyelin biosynthesis. FEBS Lett 2000; 478: 9–12
- Coste H, Martel MB, Got R. Topology of glucosylceramide synthesis in Golgi membranes from porcine submaxillary glands. Biochim Biophys Acta 1986; 858: 6–12
- Futerman AH, Pagano RE. Determination of the intracellular sites and topology of glucosylceramide synthesis in rat liver. Biochem J 1991; 280: 295–302
- Van Meer G, Holthuis JC. Sphyngolipid transport in eukariotic cells. Biochim. Biophys Acta 2000; 1486: 145–170
- Contreras FX, Villar AV, Alonso A, Kolesnick RN, Goñi FM. Sphingomyelinase activity causes transbilayer lipid translocation in model and cell membranes. J Biol Chem 2003; 278: 37169–37174
- Contreras FX, Basañez G, Alonso A, Herrmann A, Goñi FM. Asymmetric addition of ceramides but not dihydroceramides promotes transbilayer (flip-flop) lipid motion in membranes. Biophys J 2005; 88: 348–359
- López-Montero I, Vélez M, Devaux PF. Surface tension induced by sphingomyelin to ceramide conversion in lipid membranes. Biochim Biophys Acta 2007; 1768: 553–561
- Bollinger CR, Teichgräber V, Gulbins E. Ceramide-enriched membrane domains. Biochim Biophys Acta 2005; 1746: 284–294
- Herrmann A, Zachowski A, Devaux PF. Protein-mediated phospholipid translocation in the endoplasmic reticulum with a low lipid specificity. Biochemistry 1990; 29: 2023–2027
- Papadopulos A, Vehring S, López-Montero I, Kutschenko L, Stöckl M, Devaux PF, Kozlov M, Pomorski T, Herrmann A. Flippase activity detected with unlabelled lipids by shape changes of giant unilamellar vesicles. J Biol Chem 2007; 282: 15559–15568