Abstract
Kat1 is a highly selective inward-rectifying K+ channel that opens for extended periods under conditions of extreme hyperpolarization. Over 200 point mutants in the pore region of the Kat1 K+ channel were generated and examined in the yeast Saccharomyces cerevisiae and Xenopus oocytes to assess the effect of the mutations on ion selectivity. Substitutions at the tyrosine of the signature sequence G-Y-G resulted in the most significant alterations in ion selectivity, consistent with its role in the selectivity filter. However, greater than 80% of the mutations throughout the greater pore region also conferred a defect in selectivity demonstrating that the entire pore of Kat1 contributes to the ion selectivity of this channel. Surprisingly, we identified a novel class of mutant channel that conferred enhanced selectivity of K+ over Na+. Mutants of this class frequently displayed sensitivity to the competing ion Cs+. This finding has led us to speculate that the Kat1 channel pore has evolved to balance not only K+/Na+ selectivity, but selectivity over Cs+, and possibly a wide spectrum of potential competing ions.
Introduction
The crystal structures of voltage-gated K+ channels have led to significant progress in understanding the mechanism of selective ion permeation Citation[1–6] (Reviewed Citation[7–9]). In KcsA, current models suggest that a fully hydrated K+ ion approaches the channel vestibule where two discrete K+-binding positions are observed outside of the selectivity filter Citation[2]. At the outer position, the K+ ion remains fully hydrated, but upon movement to the inner position, some of the waters molecules are replaced by interactions with 4 carbonyl oxygens that face out of the pore from the signature sequence Glycine79 Citation[2] (analogous to Gly264 of Kat1). The K+ ion then enters the selectivity filter, the narrow pathway formed by the convergence of the four channel subunits, where it becomes fully dehydrated Citation[1].
The design of the selectivity filter is largely responsible for the high rate of conductivity and specificity of K+ channels. The selectivity filter is lined with the carbonyl oxygens of five amino acids including the G-Y-G signature sequence Citation[1]. The filter contains four binding sites (Positions 1–4) that surround the fully dehydrated K+ ion with 8 carbonyl oxygens Citation[1]. It is believed that rapid ion conduction occurs when the selectivity filter is occupied by two ions, either in the 1/3 or 2/4 positions of the pore with a water molecule alternating in between the ion-bound positions Citation[2], Citation[10–12]. The diameter and spacing of the carbonyl cages that form the four binding sites are optimized to allow the rapid flow of a K+ (1.33 Å ionic radius), or ions of similar size including Tl+ (1.40Å) or Rb+ (1.48Å). The larger Cs+ (1.69Å), though capable of binding within the selectivity filter Citation[13], does not freely pass through the pore and acts as a channel blocker Citation[14]. It has been proposed that the spacing between the binding sites of the K+ channel filter cannot accommodate the queue of Cs+ and water molecules required for optimal transport Citation[13]. The ability of the channel to efficiently dehydrate the K+ ion is another critical aspect of the design of the pore that also ensures both the rapid and K+-specific conduction. The architecture of the selectivity filter precisely positions the carbonyl oxygens to strip away the waters from the hydrated K+ ion with minimal energy requirement. In contrast, the energy cost to dehydrate smaller ions such as Na+ (0.95Å) that do not fit precisely into the carbonyl cage may prevent the flow of these ions Citation[1], Citation[2]. In addition, several other factors including conformational shifts between the active and inactive channel, binding site flexibility, gating, charge density, and channel co-factors may contribute to the ion specificity of the channel.
Multiple structures of the K+ channels show remarkable similarity in the pore region Citation[1], Citation[3–6] and this basic design is likely to be present across the family of voltage gated K+ channels due to the high amino acid conservation among channel pores. The design of the carbonyl oxygen-lined pore satisfies the two most evolutionarily critical functions of the channel pore, extremely rapid and specific permeation of K+. However, despite the conservation of the architecture of the channel pore, it is likely that subclasses of K+ channels exhibit subtle differences in structure and/or mechanism that are undetectable by X-ray crystallography. Thus, functional studies of K+ channels continue to contribute to the understanding of these important molecules.
Kat1 is a six-transmembrane voltage-gated inward-rectifying K+ channel from Arabidopsis thaliana that is expressed in guard cells Citation[15], Citation[16]. Kat1 opens for extended periods under conditions of extreme hyperpolarization to allow the influx of K+ leading to the swelling and opening of the stoma. Thus, it is critical that Kat1 is highly specific for K+ over Na+ to maintain the proper ionic gradient of the cells.
Expression of Kat1 in Saccharomyces cerevisiae cells that lack the endogenous K+ uptake system allows growth at low concentrations of potassium in the growth medium due to high affinity K+ uptake by the channel Citation[17], Citation[18]. We used this system to investigate ion selectivity of wild-type and mutant forms of Kat1 by adding competing ions such as sodium, cesium, and TEA to the growth medium and assessing their affect on growth under potassium-limiting conditions. We also performed two-electrode voltage clamp experiments in Xenopus oocytes to quantify novel ion specificity phenotypes that were detected with the yeast system. Our findings suggest that mutations in the G-Y-G signature sequence of Kat1 have dramatic effects on ion selectivity including increased permeation and blockage by Na+ and other ions, while changes throughout the entire pore region have significant, but smaller effects on ion selectivity.
We have also identified a gain-of-function class of channel that exhibits increased selectivity of K+ over Na+. This discovery was unexpected given the extremely high specificity of the wild-type Kat1 channel. Interestingly, a significant number of channels that conferred increased resistance to Na+ in yeast displayed loss of selectivity with respect to other ions suggesting that evolutionary pressures on the design of the K+ channel pore are not limited to specificity of K+ over Na+.
Material and methods
Mutational analysis of Kat1
The KAT1 coding sequence was cloned into the multi-copy yeast expression vector pYES2 (Invitrogen) under the control of the galactose-inducible promoter GAL1. The resulting plasmid was modified to include a poly-(A) tail at the 3' end of the gene. To facilitate subcloning of mutant pore region fragments, unique Hind III and BglII sites that did not alter the amino acid coding sequence of Kat1 were introduced at nucleotide positions 726 and 1239, respectively.
Site-directed mutations were generated using a 4-primer PCR method Citation[19]. Two outside primers were designed such that they encompassed the unique HindIII and BglII sites in the KAT1 construct. The first round of amplification generated two fragments which overlapped by approximately 30 nucleotides centered around the target codon site. The two amplicons were purified using an ion exchange column to remove primers and nucleotides. In a second PCR reaction, the two amplicons were mixed and served as the template as they were amplified using the two outside primers. The full-length product was then purified, digested, and cloned into the unique HindIII and BglII sites that were generated in KAT1. Ligation reactions with the purified vector and mutant PCR fragments were used to transform Escherichia coli. Transformants were inoculated separately into LB-AMP media and DNA was prepared using a crude mini-prep method and sequenced by dideoxy methods Citation[20] (USB).
Media and strains
Potassium transport characteristics of the wild-type (strain R757) and trk1Δ trk2Δ (strain CY162) strains of S. cerevisiae used in this study have been previously described Citation[17], Citation[21]. Yeast transformations were performed by electroporation Citation[22] and the Ura+ transformants were patched onto permissive medium (100 mM KCl) lacking uracil. After several days of growth, the patches were replica plated to the various test media. These media all contained 2% galactose and 2% sucrose for induction of the GAL1 promoter. All test media were low salt (LS) Citation[17], Citation[18], pH 5.9 (with arginine), lacking Na+ and NH4+. Growth was assessed after 2–7 days, depending upon the type of medium. Media were supplemented with KCl to 0.1, 1, 2, or 100 mM. The following competing ions were added to test media as chloride salts: Na+ (150, 500, 600, and 700 mM), Cs+ (5 and 15 mM), and Tetraethylammonium (TEA) (75 and 150 mM).
Two-electrode voltage clamp analysis
RNA from KAT1 and mutant KAT1 cDNAs was synthesized using mMessage mMachine (Ambion Inc.). Ooctyes were isolated from Xenopus laevis, (Nasco) defolliculated, injected with RNA, and incubated at 18°C in modified Barth's solution containing 96 mM Citation[23], Citation[24]. Between 2.5 and 25 ng of RNA was injected depending on expression or activity level of the channel. Recordings were made one to four days after RNA injection using an Oocyte Clamp OC-725B amplifier (Warner Instruments), filtered at 1 KHZ (Frequency Devices 902). Data were recorded and analyzed using Axon Instruments software AxoData and AxoGraph on a Macintosh PowerPC. Leakage currents were subtracted using a P/6 subtraction method Citation[25].
Basic bath solutions contained 100 mM XCl (X = K+, Na+, or Cs+), 1.8 mM CaCl2, 1.0 mM KHC03, 1.0 mM MgCl2, 10 mM HEPES (pH 7.0 with Tris base. Solutions at low pH were prepared by the addition of HCl.). Some combinations of bath solutions were used and are described in the text. At least 10 ml of bath solution was perfused during solution changes.
Amplitude and permeability ratio calculations
The magnitude of current was determined at the end of a one second pulse to −130 mV. Amplitude ratios were determined by dividing the current measurement of the test ions by the potassium current. All values are means±SE. Reversal potentials were measured by first activating channels with a one-second hyperpolarizing pulse to −130 or −150 mV, then stepping to different test potentials. The permeability ratio PX/PK was determined using the equation PX/PK=(e VrevF/RT)[K+]/[X+] based on the Nernst-Planck electrodiffusion equation Citation[26].
Results
Expression and selectivity of mutant Kat1 channels in S. cerevisiae
The yeast genetic system was used to express wild-type and mutant forms of the Kat1 K+ channel. S. cerevisiae cells in which the endogenous K+ transporters Trk1 and Trk2 are deleted are unable to grow on standard media (< 7 mM K+), but are capable of growing on medium supplemented with KCl (100 mM K+) Citation[17], Citation[18]. Expression of wild-type KAT1 in trk1Δ trk2Δ cells restores the ability to grow on medium containing as little as 50 µM K+Citation[15]. Channel selectivity can be tested by assessing growth of trk1Δ trk2Δ cells expressing Kat1 channels in the presence of a competing ion such as sodium Citation[16].
Site-directed mutations were introduced at 22 highly conserved positions in the pore of Kat1 (). In total, 208 mutants containing single amino acid substitutions were analyzed (). The mutant channels were introduced into trk1Δ trk2Δ cells and growth was initially assessed under K+-limiting conditions (2 mM K+) in the absence of other ions. The growth phenotypes conferred by the mutant Kat1 channels are summarized in and a panel of representative mutants is shown in . A total of 86 mutants were incapable of conferring growth on 2 mM K+ and were thus considered to be non-functional. These channels were not extensively analyzed, but some general features of this class are worth noting. At several positions, Y252, G262, and D265, substitutions were not well tolerated; only highly conservative changes at these positions allowed channel function. Furthermore, as we had previously shown, substitution at G264 is incompatible with channel function Citation[16].
Figure 1. Analysis of 22 highly conserved positions in the pore of Kat1. (A) Two subunits of the KcsA channel (Protein Data Bank structure 1bl8 ‘Potassium channel (KCSA) from Streptomyces lividans’ Citation[1], Citation[38]) are shown in which the analogous positions to the 22 amino acids that were mutated in Kat1 are highlighted (PyMOL software) Citation[39]. Shown in green are the five amino acids of the selectivity filter; in blue, the 11 amino acids upstream of the selectivity filter; and in red, the six amino acids downstream of the selectivity filter. (B) The amino acids from the pore region of Kat1 were aligned to the following K+ channels: KcsA Citation[40] from S. lividans Shaker Citation[41] from D. melanogaster, MthK Citation[42] from M. thermoautotrophicum, KirBac Citation[5] from B. pseudomallei, KvAP Citation[43] from A. pernix, and Kv1.2 Citation[44] from R. norvegicus. This Figure is reproduced in colour in Molecular Membrane Biology online.
![Figure 1. Analysis of 22 highly conserved positions in the pore of Kat1. (A) Two subunits of the KcsA channel (Protein Data Bank structure 1bl8 ‘Potassium channel (KCSA) from Streptomyces lividans’ Citation[1], Citation[38]) are shown in which the analogous positions to the 22 amino acids that were mutated in Kat1 are highlighted (PyMOL software) Citation[39]. Shown in green are the five amino acids of the selectivity filter; in blue, the 11 amino acids upstream of the selectivity filter; and in red, the six amino acids downstream of the selectivity filter. (B) The amino acids from the pore region of Kat1 were aligned to the following K+ channels: KcsA Citation[40] from S. lividans Shaker Citation[41] from D. melanogaster, MthK Citation[42] from M. thermoautotrophicum, KirBac Citation[5] from B. pseudomallei, KvAP Citation[43] from A. pernix, and Kv1.2 Citation[44] from R. norvegicus. This Figure is reproduced in colour in Molecular Membrane Biology online.](/cms/asset/139626f3-2f8c-46de-a78a-b7e8f8212e44/imbc_a_419006_f0001_b.jpg)
Figure 2. Activity and specificity of mutant Kat1 channels expressed in trk1Δ trk2Δ and TRK1 TRK2 cells. (A) Point mutations were introduced into residues of the highly conserved pore region of Kat1 from position 249–270. Mutant channels were expressed in trk1Δ trk2Δ and growth was assessed on permissive medium (100 mM KCl), low K+ media (2 or 0.1 mM KCl), and 2 mM KCl media supplemented with Na+ (150 and 500 mM NaCl). (B) To test for increased Na+ permeation, mutant channels were expressed in TRK1 TRK2 cells and growth was assessed on permissive medium (100 mM KCl) and media supplemented with Na+ (1.5 mM KCl/700 mM NaCl).
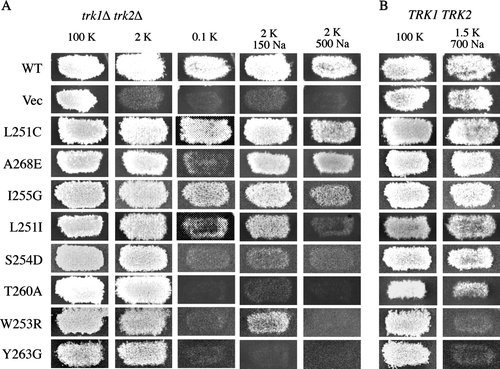
Table I. Positions of amino acid substitutions in Kat1 used in this study and phenotypes of mutant channels determined in Saccharomyces cerevisiae.
The remaining 122 mutant channels were functional and conferred growth of trk1Δ trk2Δ cells on medium containing 2 mM K+. The selectivity of these channels was tested by assessing growth of trk1Δ trk2Δ cells under low K+ conditions in the presence of Na+ as a competing ion ( and A). A channel capable of conferring strong growth in the presence of Na+ was considered K+-selective because Na+ neither blocked K+ uptake, nor was taken up at sufficient levels through the channel to cause toxicity (A, WT, L251C, and A268E). Conversely, a mutant channel whose ability to confer growth on low potassium was inhibited by the presence of Na+ was considered to be less K+-selective. Whether the channel could be permeated by Na+ or simply blocked by this ion was determined by additional analysis in yeast and two-electrode voltage clamp analysis in Xenopus oocytes (see Mutant Kat1 channels that permeate Na+).
The media used to test selectivity of Kat1 channels expressed in trk1Δ trk2Δ cells contained 2 mM KCl supplemented with 150 mM NaCl or 500 mM NaCl. Under both conditions the highly selective wild-type channel was capable of conferring strong growth (A, WT), consistent with previous results and electrophysiological data showing that this channel neither permeates, nor is blocked by Na+Citation[16], Citation[23]. Mutant channels incapable of conferring growth in the presence of 500 mM Na+ were designated Na+-sensitive and mutant channels incapable of conferring growth in the presence of 500mM or 150 mM Na+ were designated Na+-hypersensitive.
Of the 122 functional channels, 43 exhibited the Na+-sensitive phenotype, 23 of which displayed Na+-hypersensitivity (). Na+ sensitivity or hypersensitivity was associated with mutations at 15 of the 22 sites targeted for mutagenesis suggesting that most of this region is required for selectivity of K+ over Na+. Notably, 12 of the 23 Na+-hypersensitive mutants represented amino acid changes at Y263, a site in Kat1 previously analyzed by mutational analysis Citation[16]. A panel of representative mutants conferring Na+-sensitive and hypersensitive growth of trk1Δ trk2Δ cells is shown in A. Cells expressing S254D, T260A, and Y263G were hypersensitive to Na+ and unable to grow in the presence of 150 or 500 mM Na+, while cells expressing L251I, W253R, and I255G were only sensitive to the higher concentration of Na+.
Mutant Kat1 channels that permeate Na+
Channels that were unable to confer growth of trk1Δ trk2Δ cells in the presence of Na+ were broadly classified as ‘non-selective’ with respect to Na+. The loss of ion selectivity could represent increased permeation and/or block of K+ permeation by Na+. To identify those mutants that might increase permeation by Na+, the Kat1 channels were expressed in wild-type (TRK1 TRK2) cells and growth was assessed on medium containing 1.5 mM K+ supplemented with 700 mM Na+. TRK1 TRK2S. cerevisiae cells expressing wild-type Kat1 or harboring vector alone are not sensitive to this concentration of external Na+ and thus are capable of conferring strong growth under these conditions. In contrast, growth of TRK1 TRK2 cells expressing mutant alleles of KAT1 that result in increased permeation of Na+ was inhibited by this concentration of the ion (B).
This growth inhibition test previously identified amino acid substitutions at position Y263 of Kat1 as being able to confer increased Na+ permeation Citation[16]. In that study most functional substitutions at Y263 conferred increased permeation of Na+; 13 of the 15 mutants tested resulted in Na+-permeant channels (). In striking contrast, here we find that the majority of mutations at 21 other positions in the pore do not result in increased permeation of Na+ (). Only two other mutants, W253R and T256G, resulted in overtly Na+-permeant channel. Weak Na+ permeation was conferred by mutants I255A, I255V, T260A, T261L and T261N (A and ). These results distinguish position Y263 of Kat1 with respect to Na+ permeation, while also suggesting that most other residues in the pore contribute to the ion selectivity of the channel in a different role.
One of the overtly Na+-permeant mutants, W253R, was analyzed in detail by growth phenotypes in yeast and two-electrode voltage clamp analysis. As shown in A the W253R channel conferred strong growth to trk1Δ trk2Δ cells on media containing 2 mM KCl, but growth was slightly inhibited by the presence of 150 mM NaCl and completely inhibited in the presence of 500 mM NaCl. In TRK1 TRK2 cells expressing this mutant, growth was completely abolished by the presence of 700 mM Na+ suggesting a severe increase in Na+ permeation.
Wild-type and W253R channels were expressed in Xenopus oocytes for analysis by two-electrode voltage clamp. Two bath conditions were used to test the selectivity of this mutant: Ringer solutions containing either 100 mM KCl or 100 mM NaCl. In the 100 mM KCl bath, the wild-type channel elicited large inward currents under hyperpolarization similar to those reported earlier Citation[23] (). Inward currents were not detected in the Na+ bath upon hyperpolarization of the oocyte but significant outward K+ tail currents were observed upon return to the holding potential (−40 mV). The outward tail currents were likely due to the extrusion of internal K+ through the closing Kat1 channels.
Figure 3. Two-electrode voltage clamp analysis of a sodium permeable Kat1 channel. Currents elicited from wild-type Kat1 and W253R in bath solutions containing 100 mM K+ and 100 mM Na+. Cells were held at −40 mV and hyperpolarized to −150 mV in 10 mV steps. Leak currents were subtracted using a P/6 method.
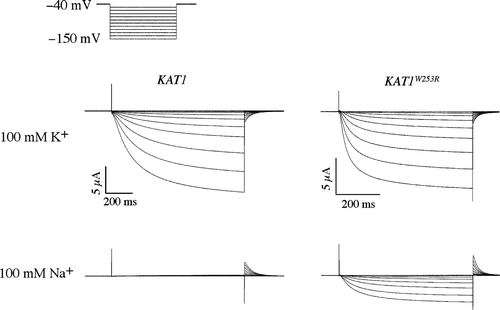
The W253R channel elicited large K+ currents upon hyperpolarization, displaying kinetics of opening and closing similar to that of the wild-type channel (). However, in contrast to the wild-type channel in the Na+ bath the W253R channel also elicited significant currents under hyperpolarization. The kinetics of the Na+ currents resembled those of the K+ current suggesting that the currents were not carried by chloride ions. In addition, this current was readily blocked by cesium further suggesting that it was carried by Na+ and not Cl- (data not shown).
Two methods were used to measure the selectivity of the wild-type and W253R channels. First, the magnitude of the Na+ current was measured at −130 mV and compared to the K+ current at that same membrane potential. Na+ flux for the wild-type channel was very small, and the magnitude of the Na+ current was < 1% (n=4) that of the K+ current. In contrast, the magnitude of the Na+ current for W253R was determined to be 16.6%±8.1% (n=4) that of the K+ current. Thus Na+ influx, which is virtually non-existent for the wild-type channel, was increased dramatically by the W253R mutation.
We next obtained the reversal voltage measurements for the wild-type and W253R channels to determine the Na+/K+ permeability ratio using the Goldman-Hodgin-Katz equation. In the K+ bath, the reversal potential determined for the wild-type channel was −4.5±3 mV (n=4). We were unable to determine an accurate reversal potential for the wild-type channel in the Na+ bath due to the lack of inward current. Because this suggested that the reversal potential in the Na+ bath was more negative than −170 mV, we used this value a conservative estimate of the reversal voltage in our calculation of the permeability ratio. We calculated the Na+/K+ permeability ratio of wild-type Kat1 to be approximately 0.15, consistent with the high selectivity of a K+ channel. The flow of current elicited by the W253R channel reversed direction in the pure K+ bath at −5.5±4.9 mV (n=4). In this case, accurate determination of the Na+ reversal potential was possible due to the significant currents elicited by the mutant channel in the pure Na+ bath. Tail currents measured in the Na+ bath were similar to those observed for the wild-type channel in the K+ bath, and there was no indication that Na+ was effectively blocking the channel. In the Na+ bath, the flow of current reversed at −93±6.8 mV (n=4) and the Na+/K+ permeability ratio for the W253R mutant was determined to be 3.1, approximately twenty times that of the wild-type channel. Note, this value would be higher were it not for the high estimate of Na+ permeation through wild-type Kat1.
Mutant Kat1 channels that are blocked by Na+
Several Kat1 mutants conferred Na+-sensitive growth of trk1Δ trk2Δ cells, but did not confer significant Na+-toxicity to wild-type cells. For example, although growth of trk1Δ trk2Δ cells expressing the T260A mutant on 2 mM KCl was completely inhibited in the presence of 150 mM or 500 mM NaCl (A), only slight growth inhibition was observed when this mutant was expressed in TRK1 TRK2 cells on media containing very high concentrations of Na+. This phenotype suggested that the mutant channel did not confer a significant increase in Na+ permeation, but rather was blocked or inactivated in the presence of Na+. Wild-type and T260A channels were expressed in Xenopus oocytes for analysis by two-electrode voltage clamp. The T260A mutant was functional in oocytes, though current amplitudes were lower than the wild-type channel () and this mutant exhibited a negative shift in voltage-dependent activation (Supplementary , online version only).
Figure 4. Two-electrode voltage clamp analysis of a sodium blocked Kat1 channel. Currents elicited from wild-type Kat1 and T260A in bath solutions containing 100 mM K+, 50 mM K+/50 mM Na+, 10 mM K+/90 mM Na+, and 100 mM Na+. Oocytes were held at −40 mV and hyperpolarized to −150 mV in 10 mV steps. Leak currents were subtracted using a P/6 method Citation[25].
![Figure 4. Two-electrode voltage clamp analysis of a sodium blocked Kat1 channel. Currents elicited from wild-type Kat1 and T260A in bath solutions containing 100 mM K+, 50 mM K+/50 mM Na+, 10 mM K+/90 mM Na+, and 100 mM Na+. Oocytes were held at −40 mV and hyperpolarized to −150 mV in 10 mV steps. Leak currents were subtracted using a P/6 method Citation[25].](/cms/asset/c5bb96d6-7e8d-4a7a-b6e7-208d23bcf47b/imbc_a_419006_f0004_b.gif)
Four bath conditions of decreasing K+:Na+ ratios were utilized containing 100 mM K+, 50 mM K+/50 mM Na+, 10 mM K+/90 mM Na+, and 100 mM Na+. In the pure K+ bath, both channels elicited large, slowly activating currents typical of those described previously for the Kat1 channel Citation[23]. In the 50 mM K+/50 mM Na+ bath, currents elicited by the wild-type channel were over 50% of that of the 100 mM K+ bath. Although large currents were also observed for the T260A channel, their magnitude relative to that of the 100 mM K+ bath was smaller than that of the wild-type channel. In the bath containing 10 mM K+/90 mM Na+, significant K+ currents were elicited by the wild-type channel. The magnitude of current measured in this bath was determined to be 33%±8% (n=3) that of the 100 mM K+ bath. The reversal voltages in the 100 mM K+ and 10 mM K+/90 mM Na+ baths were also determined to be −2.8±2.6 mV (n=4) and −58.3±4.2 mV (n=4) respectively, expected values for a highly selective K+ channel Citation[23]. In contrast, K+ currents elicited by T260A were nearly completely blocked in the presence of 90 mM Na+. Current measurements were determined to be 1.9%±0.06% (n=3) that of the 100 mM K+ bath, a greater than 15-fold reduction compared to the wild type. Accurate reversal potential measurements for T260A could not be determined in this bath due to the small magnitude of inward current. In the final bath solution containing 100 mM NaCl, no inward current was elicited by either the wild-type or T260A channels demonstrating that neither channel is permeable to Na+ under these conditions. Recordings from oocytes expressing the wild-type Kat1 channel displayed outward K+ tail currents apparent upon return to the resting voltage (−40 mV) in the baths containing 10 mM K+/90 mM Na+ and 100 mM Na+ (). These currents were not observed in recordings of the T260A channel suggesting that external Na+ also blocks the outward flow of K+ through the pore upon return to the resting potential when the driving force favors the efflux of K+.
A previous study of the Kat1 pore examined several mutations at position 256, some of which were found to affect sodium sensitivity of the channel Citation[27]. Under their test conditions, two mutants, T256E and T256Q, conferred sensitivity to Na+ when expressed in trk1Δ trk2Δ cells. The channels were not tested for Na+ permeation using the yeast system, but our present analysis of the same mutants did not reveal increased Na+ permeation (). When expressed in Xenopus oocytes, these mutants displayed increased block, but not permeation by Na+Citation[27]. Based on the results of Uozumi et al. and on the results with the T256E, T256Q, and T260A mutants described here, we conclude that mutations which confer Na+ sensitivity when expressed in trk1Δ trk2Δ cells but which do not confer Na+ toxicity to TRK1 TRK2 cells are likely to be blocked, but not permeated by sodium.
Mutant Kat1 channels that confer resistance to Na+
Two-thirds of functional Kat1 mutants did not confer Na+-sensitive growth and were classified as highly selective for K+ over Na+. We considered the possibility that some of the mutations in KAT1 might confer ‘Na+ resistance’ or increased K+ selectivity over Na+. To test this, wild-type S. cerevisiae cells expressing mutant Kat1 channels that were designated as highly selective were tested for their ability to grow on medium where the Na+:K+ ratio was 6,000:1 (0.1 mM K+/600 mM Na+). On this medium, cells expressing wild-type Kat1 or vector alone are incapable of growth despite the presence of the Trk1 and Trk2 transporters (, WT, Vec). Two non-exclusive scenarios can explain this phenotype: K+ uptake via the endogenous K+ transporters might not occur at a rate sufficient to allow growth in the presence of such high Na+:K+ conditions, and/or low affinity uptake of Na+ through some other portal of entry may result in cytotoxicity.
Figure 5. Testing mutant Kat1 channels for Na+ resistance. (A) To assess channel function and confirm that channels were not sensitive to Na+, the mutant Kat1 channels were expressed in trk1Δ trk2Δ growth was assessed on permissive medium (100 mM KCl) and 2 mM KCl media supplemented with Na+ (150 and 500 mM NaCl). Na+ resistance was shown by observing enhanced growth of (A) trk1Δ trk2Δ and (B) TRK1 TRK2 cells expressing mutant Kat1 channels on medium supplemented with 0.1 mM KCl/600 mM NaCl.
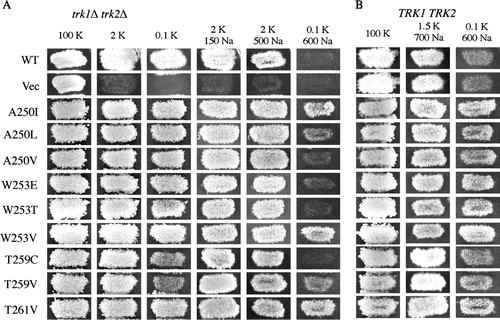
Surprisingly, 24 of the mutant Kat1 channels conferred Na+-resistant growth, i.e., growth on medium containing 0.1 mM K+/600 mM Na+ that was stronger than that exhibited by cells expressing the wild-type channel (; nine examples are shown in ). This phenotype suggested that the channels had developed enhanced selectivity for K+ over Na+. Although K+ channels have evolved to be highly selective for K+ over Na+, a surprisingly large percentage of the point mutants exhibited this apparently increased K+ selectivity phenotype in yeast. Several of the Kat1 mutants that conferred Na+-resistant growth also conferred growth of trk1Δ trk2Δ cells under identical conditions indicating that this property was independent of the endogenous yeast K+ transporters (A, A250I, A250L, W253V, T259V, T261V).
Several mutants that conferred Na+-resistant growth of wild-type yeast cells were also analyzed using two-electrode voltage clamp. However, analysis of this class of mutant in the oocyte system presented a unique problem. The wild-type Kat1 channel is highly selective for K+ over Na+; permeation or blockage by Na+ is virtually undetectable. Thus, mutations that conferred enhanced selectivity of K+ over Na+ would likely be indistinguishable from the wild-type channel in this system.
The wild-type and W253V channels were analyzed by two-electrode voltage clamp to assess whether a difference in selectivity could be detected (). Four bath conditions containing various concentrations of K+ and Na+ were used. In a bath containing 100 mM K+ and no Na+, both channels elicited large slowly activating K+ currents typical of those described previously (). Recordings in baths containing 10 mM K+/90 mM Na+ or 1 mM K+/99 mM Na+ also elicited significant inward currents by both channels. Tail currents recorded from both channels were in the positive direction due to an increased driving force for K+ efflux after return to the holding potential (−40 mV). We did not detect inward currents for either channel in the bath containing 100 mM Na+. Both channels displayed outward tail currents in the Na+ bath that were similar to those of the 1 mM K+/99 mM Na+ bath. In summary, the wild-type and W253 channels behaved identically in all four test baths under the conditions tested.
Figure 6. Two-electrode voltage clamp analysis of a sodium resistant Kat1 channel. Currents elicited from wild-type Kat1 and W253V in bath solutions containing 100 mM K+, 10 mM K+/90 mM Na+, 1 mM K+/99 mM Na+, and 100 mM Na+. Cells were held at −40 mV and hyperpolarized to −150 mV in 10 mV steps. Leak currents were subtracted using a P/6 method.
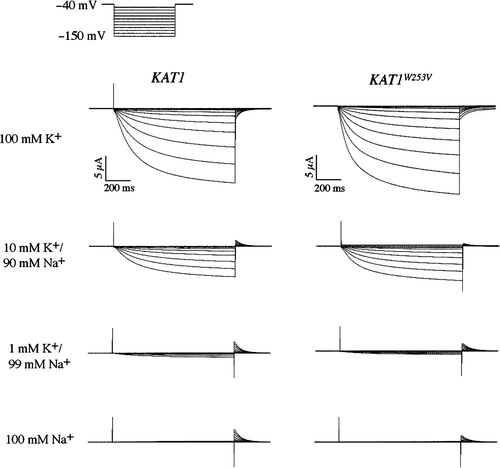
Oocytes expressing either the wild-type or W253V channel were pulsed to −130 mV to activate channel activity and depolarized to a series of test voltages to determine the zero current potential under each bath condition. In this experiment, the reversal potentials for the wild-type and W253V channels respectively were determined to be −2.8±2.6 mV (n=4) and −4.5±3.0 mV (n=4) in the 100 mM K+ bath, −58.3±4.2 mV (n=4) and −58.9±3.0 mV (n=4) in the 10 mM K+/90 mM Na+ bath, and −110.1±4.7 mV (n=4) and −113.1±3.4 mV (n=4) in the 1 mM K+/99 mM Na+ bath. These reversal potential shifts of approximately 58 mV from each bath solution were indicative of a perfectly selective K+ channel as predicted by the Nernst equation Citation[26]. The lack of any inward current in the 100 mM Na+ bath further showed that both the wild-type and W253V channels were highly selective against Na+.
Electrophysiological comparison between wild-type Kat1 and the W253V mutant failed to reveal differences between the channels. This is a limitation of the oocyte system for this type of analysis; because Na+ block or uptake is virtually undetectable in wild-type Kat1 by two-electrode voltage clamp, a decrease in affinity for Na+ block or Na+ uptake would also be undetectable. In contrast, the phenotypes generated by expression of the mutant Kat1 channels in yeast provided independent evidence for enhanced selectivity against Na+.
Sensitivity and resistance to Cs+ and TEA+
Cesium and tetraethylammonium (TEA) are potent blockers of Kat1 and other voltage-gated K+ channels. The collection of Kat1 mutants was tested for sensitivity to these blocking agents based upon growth phenotypes in S. cerevisiae on media supplemented with 5 mM Cs+ or 75 mM TEA+. Wild-type Kat1 confers strong growth of trk1Δ trk2Δ cells under both of these conditions (A) and mutants unable to confer growth on one of these media were designated ‘sensitive’ to the given blocking agent. Conversely, mutant channels able to confer increased resistance to Cs+ and/or TEA+ were identified by their ability to confer growth on media containing levels of these agents that are toxic to cells expressing wild-type Kat1. Thus, Cs+- and TEA+-resistant channels were found to confer growth to trk1Δ trk2Δ cells on media containing 2 mM K+ supplemented with 15 mM Cs+ or 150 mM TEA+.
Figure 7. Cesium and TEA-associated growth phenotypes conferred by mutant Kat1 channels. (A) trk1Δ trk2Δ cells expressing Kat1 channels were grown on permissive medium (100 mM KCl), low K+ media (2 or 0.1 mM KCl), and 2 mM KCl media supplemented with Cs+ (5 and 15 mM CsCl) or TEA+ (75 and 150 mM TEA+). (B) In wild-type cells, growth was assessed on permissive medium (100 mM KCl) and media supplemented with Na+ (1.5 mM KCl/700 mM NaCl or 0.1 mM KCl/600 mM NaCl).
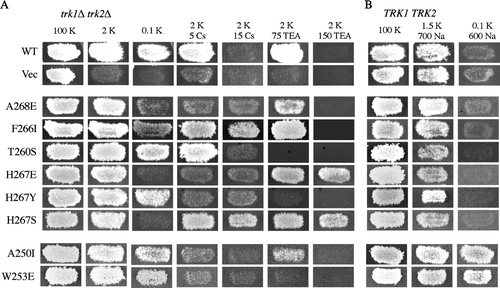
Representative mutants conferring phenotypes associated with Cs+ and TEA+ are shown in (a complete listing of Cs+ and TEA+ associated phenotypes is presented in ). One mutant, A268E, displayed Cs+ sensitivity and was unable to confer growth of trk1Δ trk2Δ cells on medium containing 2 mM K+/5 mM Cs+ (A). Conversely, the F266I channel was resistant to Cs+, and conferred strong growth on media supplemented with 5 or 15 mM Cs+. The T260S channel displayed TEA+ sensitivity and was unable to confer growth as strong as the wild-type channel on 2 mM K+/75 mM TEA+. In contrast, the H267E mutant was resistant to TEA+ and conferred growth of trk1Δ trk2Δ cells on 150 mM TEA+. Some mutants conferred aberrant growth phenotypes in the presence of either blocking agent. For example, the H267Y mutant was sensitive to both Cs+ and TEA+, while the H267S mutant was resistant to both of these blockers. Interestingly, several mutants displaying sensitivity to Cs+ and TEA+ were mapped to position H267 of Kat1, analogous to position T249 of Shaker that has been shown to be its external TEA+ binding site Citation[28].
Cesium is a potent voltage-dependent blocker of wild-type Kat1 with K+ currents substantially blocked by as little as 1 mM Cs+ in the bath solution. Block by cesium is typified by a substantial reduction in the overall magnitude of current, while at more extreme hyperpolarized potentials currents do not increase linearly and level off or become smaller Citation[29]. The cesium resistant mutant F266I was expressed in Xenopus oocytes for analysis by two-electrode voltage clamp (). Two bath solutions containing 90 mM K+ or 90 mM K+/10 mM Cs+ were used to analyze the effect of cesium on channel activity. The F266I mutant elicited large slowly activating K+ currents similar to those of the wild-type channel. In the presence of 10 mM Cs+, the current magnitude of both channels was reduced, although to a larger degree for the wild-type channel (). The magnitude of current for the wild-type channel determined in the 90 mM K+/10 mM Cs+ bath was determined to be 11%±3% (n=4) that of the magnitude of current measured in 90 mM K+. In contrast, the value for F266I was determined to be 20%±3% (n=4) representing approximately a 2-fold increase in the magnitude of K+ currents in a bath solution containing 10 mM CsCl. The mutation apparently does not completely remove Cs+ block, but does partially relieve the voltage-dependent block that is a characteristic of the wild-type channel. These findings are consistent with the cesium-resistant phenotype that was observed in yeast.
Figure 8. Two-electrode voltage clamp analysis of a cesium resistant Kat1 channel. Currents elicited from wild-type Kat1 and F266I in bath solutions containing 90 mM K+ and 90 mM K+/10 mM Cs+. Cells were held at −40 mV and hyperpolarized to −150 mV in 10 mV steps. Leak currents were subtracted using a P/6 method.
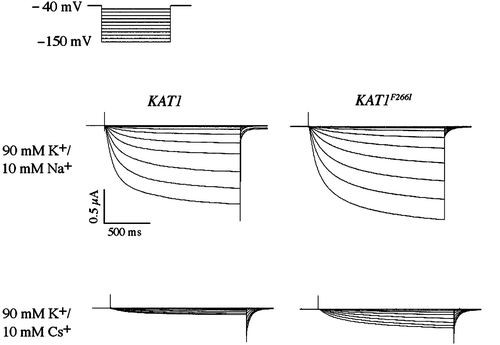
Interestingly, approximately half of the Na+- resistant mutants displayed increased sensitivity to Cs+; for example, the A250I and W253E channels were capable conferring of strong growth to TRK1Δ TRK2Δ cells on medium containing 0.1 mM K+/600 mM Na+, while unable to confer growth to trk1Δ trk2Δ cells in the presence of 5 mM Cs+ (B). The relationship between Cs+ sensitivity and Na+ resistance suggested a possible evolutionary connection between these two channel phenotypes that is addressed further in the Discussion section.
Discussion
The relationship between specific sites of the highly conserved K+ channel pore and cations that interact with the channel was studied by investigating the functional effects of 208 pore mutants. Ion selectivity of the mutant channels was assessed using two independent tests: Growth phenotypes in S. cerevisiae and recordings by two-electrode voltage clamp analysis in Xenopus oocytes. Our results show that K+ ion permeation and specificity is most critically related to the G-Y-G signature sequence of the pore. Most mutations at position Y263 of the signature sequence drastically increased Na+ permeation through the channel, while changes at the highly conserved flanking positions in the pore rarely resulted in such a severe loss of selectivity. These results are consistent with previous mutagenesis experiments Citation[16], Citation[30] as well as the published crystal structures of voltage-gated K+ channels that show the selectivity filter of the channel is formed by backbone carbonyls of the G-Y-G signature sequence Citation[1].
Of the 15 functional substitutions at position Y263, 13 resulted in a channel that conferred increased permeation to Na+. In contrast, of 189 point mutations at 21 other positions in the pore, only two (W253R and T256G) resulted in strongly Na+-permeant channels. One of these channels, W253R, was analyzed by two-electrode voltage clamp and shown to elicit significant Na+ currents. In the KcsA structure, the analogous tryptophan (W68) is intimately associated with the signature sequence tyrosine (Y263 of Kat1) forming hydrogen bonds and van der Waal contacts Citation[1]. This conserved pair of aromatics, positions Y252 and W253 of Kat1, has also been studied in Shaker (positions W434 and W435), for their role in channel inactivation, pore packing, and conductance properties. Several mutations at these positions have been shown to completely eliminate ion conductance, while other mutations allow Na+ and Li+ to pass through the channel Citation[31]. At position Y252 of Kat1, only the conservative phenylalanine substitution yielded a functional channel, while at position W253, numerous substitutions were tolerated, though most had significant modifications of ion specificity. These results suggest that the Y252-W253 pair play a significant role in the ion specificity of the Kat1 pore and that substitutions at either of these positions likely distort the structure of the selectivity filter.
Mutants that displayed a less severe defect in K+/Na+ selectivity, blockage, but not permeation by Na+ were also identified. This class of approximately 40 mutants conferred Na+-sensitive growth of trk1Δ trk2Δ cells, but did not confer Na+ toxicity to wild-type cells suggesting that they were blocked by this ion. One of these mutants, T260A, was analyzed in the oocyte system and in contrast to the wild-type channel, inward and outward K+ currents elicited by this mutant were effectively blocked by Na+. The T260A mutant also conferred slight Na+ toxicity to TRK1 TRK2 cells suggesting that low levels of Na+ permeated this channel; however, in a pure Na+ bath no inward current was detected. The ability to detect toxic ion permeation in S. cerevisiae underscores the sensitivity of growth phenotypes in this organism. Phenotypes that develop over a period of days are capable of detecting minor alterations in ion selectivity that are otherwise undetectable by conventional electrophysiology.
We considered the possibility that an effect on gating, or other channel function (for example a defect in general ion conductance, localization, expression level, folding, stability, pore collapse, etc.) could potentially be misinterpreted as a ‘selectivity’ phenotype. For example, if the presence of the competing ion in the growth medium led to inactivation of the channel, this situation might be interpreted as a defect in selectivity. In the case of the T260A mutant, this channel exhibited a negative shift in voltage-dependent activation that could potentially contribute to the observed growth phenotype. However, we believe that these potential issues are minimized for the following reasons. First, we initially tested all of the mutated channels for activity (conferring growth of trk1Δ trk2Δ cells on K+-limiting medium) to ensure that the mutant channels functioned at threshold levels. Second, the Kat1 channel is a strongly regulated by the membrane potential and opens under hyperpolarization (< −80 mV) Citation[23]. Although it is difficult to measure the membrane potential of yeast, estimates range from −80 mV (resting cells) to −130 mV (metabolizing cells) at pH 7.5 Citation[32], suggesting that the channel would be active when expressed in yeast. Third, the presence of competing ions is not known to influence Kat1 activity. Inward rectification in Kat1 is not dependent upon the external K+ concentration Citation[23], Citation[33] and there is not an apparent N-type inactivation domain as in Shaker Citation[34]. It is possible that a mutation could have conferred sensitivity to divalent cations, but this possibility seems unlikely because Kat1 is not blocked by divalent ions Citation[35] and because the growth media and bath solutions used contained limited Ca++ and Mg++ (typically 2 mM or lower). Fourth, we used the oocyte system to independently confirm the selectivity phenotypes observed in yeast. Of the channels tested in oocytes, we observed alterations in permeation and blockage by competing ions.
In addition to the Na+-permeable and Na+-blocked Kat1 mutants, we also identified a class of Na+-resistant channels that exhibited behavior consistent with increased K+>Na+ selectivity. This was surprising given the high degree of ion selectivity that Kat1 and other K+ channels already display for K+ over Na+. One explanation for this phenotype is that these mutations alter the structure of the selectivity filter, although we have shown that no mutation at any of the G-Y-G positions results in a Na+-resistant channel. It is possible that the Na+-resistant phenotype is due to indirect effects on the G-Y-G selectivity filter from a secondary site. Several Na+-resistant mutants were identified at position W253, the site of one of the strongly Na+-permeant mutant channels (W253R) that is believed to interact with the signature sequence tyrosine Citation[1]. Clusters of Na+-resistant channels were also identified at positions A250 and N270 that map to the vestibule region of the pore. Thus, we suggest that some of the Na+ resistant channel phenotypes may be a consequence of alterations to the pore outside of the selectivity filter (see below).
Kat1 is a highly selective inward-rectifying channel that opens for extended periods under conditions of extreme hyperpolarization. Its biological role under such circumstances is to maintain a high rate of K+ uptake while preventing uptake or blockage by Na+. The crystal structures of the K+ channel pore suggest a model in which K+ permeation is mediated by specific interactions between this ion and the carbonyl rings form the lining of the selectivity filter; Na+ does not efficiently traverse the pore due to its unfavorable interactions with these carbonyl rings. In agreement with this model, we have shown that alterations to the G-Y-G selectivity filter, but not other parts of the pore, can dramatically increase the Na+ permeation.
Nevertheless, examination of mutants at 22 positions throughout the pore revealed that every position that yielded at least two functional mutant channels exhibited defects in ion specificity. In fact, greater than 80% of the functional channel mutants exhibited an aberrant phenotype with regard to ion selectivity. These results suggest that the entire pore, not only the selectivity filter, is involved with determining ion selectivity of the channel.
Based upon these results, we hypothesize that the outer surface of the Kat1 pore contains a K+-selective binding surface to attract and concentrate this ion prior to entry into the narrow selectivity filter. Zhou et al. have shown by X-ray crystallography that K+ ions can occupy two sites above the selectivity filter in the vestibule region of the Kcsa channel Citation[2]. At the site nearest to the selectivity filter, the carbonyl group of the second of the signature sequence glycine residues interacts with the partially dehydrated K+ ion upon entry into the selectivity filter. Consiglio et al. have also demonstrated that residue K356 interacts with a binding site on the outer vestibule of Kv2.1 and that this site is specific for K+ over Na+Citation[36]. We suggest that the K+-selective binding surface of Kat1 contains pockets that are favorable for K+ binding and unfavorable for Na+ or other competing ions (). Furthermore, if a competing ion were to bind the K+ selective binding surface it could be readily exchanged for K+. This is unlike the narrow selectivity filter where, if Na+ were readily allowed to enter into the narrow selectivity filter and interact with the carbonyl oxygens, the time required to exchange the Na+ for a K+ would likely lead to a significant drop in K+ conduction or actual blockage of the pore. However, we do not observe a any evidence for Na+ block of K+ flow, even at extreme conditions when the K+:Na+ is 1:100 (or greater in the yeast system) suggesting that K+ is at least partially pre-selected before it enters the selectivity filter.
Figure 9. Model of a K+ selective binding site on the surface of the channel. Two binding sites are modeled, one for normal K+-binding (left) and Na+-resistant K+ binding (right). The possible interactions between these sites and K+, Na+, and Cs+ ions are shown. The arrows represent tight binding (large arrow), weak binding (small arrow), reversible binding (two arrows), and no binding (X-out arrow).
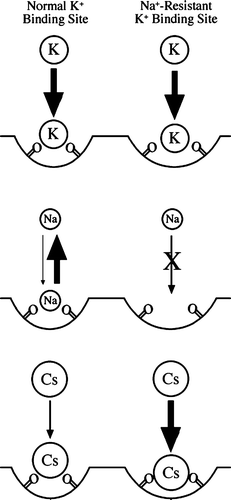
We propose that mutations in Kat1 outside of the selectivity filter that alter Na+ selectivity target the K+-selective binding site on the outer surface of the channel. This model suggests that alterations to this surface can both increase or decrease binding by Na+. In a mechanism similar to that of the selectivity filter, we propose that widening the binding site may decrease affinity for Na+ by decreasing the strength of interactions with negatively charged groups of the binding site (). In addition, we propose that a wider binding site may favor binding of large cations. We have observed that clusters of Na+ resistant mutants mapped to positions A250 and N270 on the outer vestibule of Kat1 and many mutants at these positions also exhibited altered specificity for TEA and/or Cs+. For example, at position A250, mutations to G, I, L and V conferred Na+ resistance in wild-type cells, but the I, L, and V mutants also conferred sensitivity to cesium in trk1Δ trk2Δ cells (, , A250I). Similarly, at position N270 substitutions to I, L, S, and V conferred Na+ resistance, while substitutions to C, D, G, I, M, P, and V conferred sensitivity to cesium and/or TEA ().
In summary, we propose that the entire pore is designed to create a finely-tuned mechanism for K+ selectivity. The outer surface and inner regions of this structure are precisely constructed such that K+ ions will approach, enter, and flow through the channel without interference from competing ions. Na+, the most likely ion to compete with K+, does not efficiently traverse, nor block the channel. Interestingly, we have shown that single amino acid substitutions in Kat1 can convert it into a channel that exhibits increased specificity for K+ over Na+. Such a change might be considered an improvement for an inward rectifying voltage-gated K+ channel, however, we have also shown that increased K+ selectivity over Na+ is often accompanied by a loss in selectivity to Cs+, TEA+, NH4+, and possibly other competitive ions. Cs+ is a physiologically relevant competing ion for a plant channel such as Kat1, therefore an increase in K+ selectivity over Na+ that is accompanied by a loss in selectivity to Cs+ could be detrimental for the plant (reviewed Citation[37]). Thus, we suggest that the Arabidopsis K+ channels have evolved to delicately balance K+ conductance with multiple competing ions including, but not limited to Na+ and Cs+.
As technological advancements in the structural study of K+ channels move forward, additional structures of K+ channels and K+ channel mutants will become available. Our identification of the Kat1 mutants that alter channel selectivity may be useful starting point for structural studies of ion selectivity. For example, mutations at Y263 of the selectivity filter should confer significant structural rearrangement of the selectivity filter, while the mutations at W253 may confer a more subtle effect. In addition, our discovery that sites that lie outside the selectivity filter can alter Na+ resistance of the channel should prove informative in future structural studies that address the mechanism of ion selectivity.
Acknowledgements
We thank Delphine Bichet, Helen Lai, and Friederike Haass for providing insightful comments on this work. Declaration of interest : The authors report no conflicts of interest. The authors alone are responsible for the content and writing of the paper.
Supplementary Material 1
Download GIF Image (32.5 KB)References
- Doyle DA, Morais Cabral J, Pfuetzner RA, Kuo A, Gulbis JM, Cohen SL, Chait BT, MacKinnon R. The structure of the potassium channel: Molecular basis of K+ conduction and selectivity. Science 1998; 280: 69–77
- Zhou Y, Morais-Cabral JH, Kaufman A, MacKinnon R. Chemistry of ion coordination and hydration revealed by a K+ channel-Fab complex at 2.0 A resolution. Nature 2001; 414: 43–48
- Jiang Y, Lee A, Chen J, Cadene M, Chait BT, MacKinnon R. Crystal structure and mechanism of a calcium-gated potassium channel. Nature 2002; 417: 515–522
- Jiang Y, Lee A, Chen J, Ruta V, Cadene M, Chait BT, MacKinnon R. X-ray structure of a voltage-dependent K+ channel. Nature 2003; 423: 33–41
- Kuo A, Gulbis JM, Antcliff JF, Rahman T, Lowe ED, Zimmer J, Cuthbertson J, Ashcroft FM, Ezaki T, Doyle DA. Crystal structure of the potassium channel KirBac1.1 in the closed state. Science 2003; 300: 1922–1926
- Long SB, Campbell EB, Mackinnon R. Crystal structure of a mammalian voltage-dependent Shaker family K+ channel. Science 2005; 309: 897–903
- Gouaux E, Mackinnon R. Principles of selective ion transport in channels and pumps. Science 2005; 310: 1461–1465
- Luzhkov VB, Aqvist J. Ions and blockers in potassium channels: Insights from free energy simulations. Biochim Biophys Acta 2005; 1747: 109–120
- Noskov SY, Roux B. Ion selectivity in potassium channels. Biophys Chem 2006; 124: 279–291
- Aqvist J, Luzhkov V. Ion permeation mechanism of the potassium channel. Nature 2000; 404: 881–884
- Berneche S, Roux B. Molecular dynamics of the KcsA K+ channel in a bilayer membrane. Biophys J 2000; 78: 2900–2917
- Shrivastava IH, Sansom MS. Simulations of ion permeation through a potassium channel: Molecular dynamics of KcsA in a phospholipid bilayer. Biophys J 2000; 78: 557–570
- Zhou Y, MacKinnon R. The occupancy of ions in the K+ selectivity filter: charge balance and coupling of ion binding to a protein conformational change underlie high conduction rates. J Mol Biol 2003; 333: 965–975
- LeMasurier M, Heginbotham L, Miller C. KcsA: It's a potassium channel. J Gen Physiol 2001; 118: 303–314
- Anderson JA, Huprikar SS, Kochian LV, Lucas WJ, Gaber RF. Functional expression of a probable Arabidopsis thaliana potassium channel in Saccharomyces cerevisiae. Proc Natl Acad Sci USA 1992; 89: 3736–3740
- Nakamura RL, Anderson JA, Gaber RF. Determination of key structural requirements of a K+ channel pore. J Biol Chem 1997; 272: 1011–1018
- Gaber RF, Styles CA, Fink GR. TRK1 encodes a plasma membrane protein required for high-affinity potassium transport in Saccharomyces cerevisiae. Mol Cell Biol 1988; 8: 2848–2859
- Ko CH, Buckley AM, Gaber RF. TRK2 is required for low affinity K+ transport in Saccharomyces cerevisiae. Genetics 1990; 125: 305–312
- Higuchi, R. 1990. Recombinant PCR. MA Innis, Gelfand, DH, Sninsky, JJ, White, TJ, In:. PCR Protocols: A guide to methods and applications. San Diego: Academic Press. pp 177–183.
- Sanger F, Nicklen S, Coulson AR. DNA sequencing with chain-terminating inhibitors. Proc Natl Acad Sci USA 1977; 74: 5463–5467
- Ko CH, Gaber RF. TRK1 and TRK2 encode structurally related K+ transporters in Saccharomyces cerevisiae. Mol Cell Biol 1991; 11: 4266–4273
- Becker, DM, Guarente, L. 1991. High-efficiency transformation of yeast by electroporation. C Guthrie, Fink, GR, In:. Guide to yeast genetics and molecular biology. San Diego: Academic Press. pp 182–187.
- Schachtman DP, Schroeder JI, Lucas WJ, Anderson JA, Gaber RF. Expression of an inward-rectifying potassium channel by the Arabidopsis KAT1 cDNA. Science 1992; 258: 1654–1658
- Shroeder JI. Heterologous expression and functional analysis of higher plant transport proteins in Xenopus oocytes. Methods 1994; 6: 70–81
- Bezanilla F, Armstrong CM. Inactivation of the sodium channel. I. Sodium current experiments. J Gen Physiol 1977; 70: 549–566
- Hille, B. 1992. Ionic channels of excitable membranes. 2nd. Sunderland, MA: Sinauer Associates, Inc.
- Uozumi N, Gassmann W, Cao Y, Schroeder JI. Identification of strong modifications in cation selectivity in an Arabidopsis inward rectifying potassium channel by mutant selection in yeast. J Biol Chem 1995; 270: 24276–24281
- Yool AJ, Schwarz TL. Alteration of ionic selectivity of a K+ channel by mutation of the H5 region. Nature 1991; 349: 700–704
- Ichida AM, Schroeder JI. Increased resistance to extracellular cation block by mutation of the pore domain of the Arabidopsis inward-rectifying K+ channel KAT1. J Membr Biol 1996; 151: 53–62
- Heginbotham L, Lu Z, Abramson T, MacKinnon R. Mutations in the K+ channel signature sequence. Biophys J 1994; 66: 1061–1067
- Starkus JG, Kuschel L, Rayner MD, Heinemann SH. Macroscopic Na+ currents in the ‘Nonconducting’ Shaker potassium channel mutant W434F. J Gen Physiol 1998; 112: 85–93
- Borst-Pauwels GWFH. Ion transport in yeast. Biochim Biophys Acta 1981; 650: 88–127
- Schroeder JI, Fang HH. Inward-rectifying K+ channels in guard cells provide a mechanism for low-affinity K+ uptake. Proc Natl Acad Sci USA 1991; 88: 11583–11587
- Marten I, Hoshi T. The N-terminus of the K channel KAT1 controls its voltage-dependent gating by altering the membrane electric field. Biophys J 1998; 74: 2953–2962
- Hoshi T. Regulation of voltage dependence of the KAT1 channel by intracellular factors. J Gen Physiol 1995; 105: 309–328
- Consiglio JF, Andalib P, Korn SJ. Influence of pore residues on permeation properties in the Kv2.1 potassium channel. Evidence for a selective functional interaction of K+ with the outer vestibule. J Gen Physiol 2003; 121: 111–124
- Hampton CR, Bowen HC, Broadley MR, Hammond JP, Mead A, Payne KA, Pritchard J, White PJ. Cesium toxicity in Arabidopsis. Plant Physiol 2004; 136: 3824–3837
- Bernstein FC, Koetzle TF, Williams GJ, Meyer EF, Jr, Brice MD, Rodgers JR, Kennard O, Shimanouchi T, Tasumi M. The Protein Data Bank. A computer-based archival file for macromolecular structures. Eur J Biochem 1977; 80: 319–324
- DeLano WL. The PyMOL Molecular Graphics System. DeLano Scientific, Palo Alto 2002
- Schrempf H, Schmidt O, Kummerlen R, Hinnah S, Muller D, Betzler M, Steinkamp T, Wagner R. A prokaryotic potassium ion channel with two predicted transmembrane segments from Streptomyces lividans. Embo J 1995; 14: 5170–5178
- Butler A, Wei AG, Baker K, Salkoff L. A family of putative potassium channel genes in Drosophila. Science 1989; 243: 943–947
- Smith DR, Doucette-Stamm LA, Deloughery C, Lee H, Dubois J, Aldredge T, Bashirzadeh R, Blakely D, Cook R, Gilbert K, Harrison D, Hoang L, Keagle P, Lumm W, Pothier B, Qiu D, Spadafora R, Vicaire R, Wang Y, Wierzbowski J, Gibson R, Jiwani N, Caruso A, Bush D, Reeve JN, et al. Complete genome sequence of Methanobacterium thermoautotrophicum deltaH: Functional analysis and comparative genomics. J Bacteriol 1997; 179: 7135–7155
- Kawarabayasi Y, Hino Y, Horikawa H, Yamazaki S, Haikawa Y, Jin-no K, Takahashi M, Sekine M, Baba S, Ankai A, Kosugi H, Hosoyama A, Fukui S, Nagai Y, Nishijima K, Nakazawa H, Takamiya M, Masuda S, Funahashi T, Tanaka T, Kudoh Y, Yamazaki J, Kushida N, Oguchi A, Kikuchi H, et al. Complete genome sequence of an aerobic hyper-thermophilic crenarchaeon, Aeropyrum pernix K1. DNA Res 1999; 6: 83–101–145–152
- Stuhmer W, Ruppersberg JP, Schroter KH, Sakmann B, Stocker M, Giese KP, Perschke A, Baumann A, Pongs O. Molecular basis of functional diversity of voltage-gated potassium channels in mammalian brain. Embo J 1989; 8: 3235–3244