Abstract
Outer membrane vesicles (OMVs) (∼50–250 nm in diameter) are produced by both pathogenic and nonpathogenic bacteria as a canonical end product of secretion. In this review, we focus on the OMVs produced by gram-negative bacteria. We provide an overview of the OMV structure, various factors regulating their production, and their role in modulating host immune response using a few representative examples. In light of the importance of the diverse cargoes carried by OMVs, we discuss the different modes of their entry into the host cell and advances in the high-throughput detection of these OMVs. A conspicuous application of OMVs lies in the field of vaccination; we discuss its success in immunization against human diseases such as pertussis, meningitis, shigellosis and aqua-farming endangering diseases like edwardsiellosis.
Introduction
Outer membrane vesicles (OMVs) are spherical outer membrane (OM) products of gram-negative (Escherichia coli, Pseudomonas aeruginosa, Pseudomonas gingivalis, Salmonella typhi, Yersinia pestis, Vibrio cholerae and Helicobacter pylori) and gram-positive bacteria, archaea and some eukaryotes such as fungi (Amano et al., Citation2010; Bauman & Kuehn, Citation2009; Brown et al., Citation2015; Ellis et al., Citation2010; Horstman & Kuehn, Citation2000; Ismail et al., Citation2003; Kulp & Kuehn, Citation2010; Mirlashari et al., Citation2001; Schwechheimer et al., Citation2013). Blebbing of the OM of gram-negative bacteria releasing vesicles was observed from late 1960s (Chatterjee & Das, Citation1967, Citation1966; Work et al., Citation1966), and electron micrographs from these actively growing bacteria (first observed in V. cholerae) showed OMV formation ranging in size between ∼40 and 110 nm. It was reported that the formation of OMVs was not dependent on the nature of growth media and cells from both active and stationary phases were able to produce OMVs. However, the functional relevance of OMV formation in the bacterial physiology and pathogenesis were elucidated by later works (Deatherage et al., Citation2009; Horstman and Kuehn, Citation2000; Kadurugamuwa & Beveridge, Citation1995; Li et al., Citation1998).
Outer membrane vesicles (∼50–250 nm in diameter) are produced by both pathogenic and nonpathogenic bacteria and are involved in diverse functions such as mediating bacterial intercellular communication, promoting virulence, elimination of unwanted components and modulating host immune response (Bauman & Kuehn, Citation2009; Ellis et al., Citation2010; Horstman & Kuehn, Citation2000; Ismail et al., Citation2003; Kulp & Kuehn, Citation2010; Mirlashari et al., Citation2001; Schwechheimer & Kuehn, Citation2015; Schwechheimer et al., Citation2013; Yaron et al., Citation2000). The diverse functions performed by OMVs are conferred by the different cargoes such as DNA (Deatherage et al., Citation2009; Renelli et al., Citation2004), RNA (Bernadac et al., Citation1998; Ghosal et al., Citation2015; Koeppen et al., Citation2016; Sjostrom et al., Citation2015), toxins (Bielaszewska et al., Citation2017; Horstman & Kuehn, Citation2000; Lindmark et al., Citation2009; Zakharzhevskaya et al., Citation2017) and many different proteins from the bacterial outer membrane (OM), periplasm and cytoplasm (Kaparakis-Liaskos & Ferrero, Citation2015) carried by OMVs. Important periplasmic components entrapped by OMVs include alkaline phosphatase, phospholipase C, proelastase, protease and peptidoglycan (PG) hydrolase (Li et al., Citation1998). Intensive proteomics analysis of OMVs produced from different bacterial species such as Myxococcus xanthus (Berleman et al., Citation2014; Kahnt et al., Citation2010), H. pylori (Mullaney et al., Citation2009), P. aeruginosa (Choi et al., Citation2011), Campylobacter jejuni (Jang et al., Citation2014), Neisseria meningitides (Lappann et al., Citation2013), P. syringae (Kulkarni et al., Citation2014), Aggregatibacter actinomycetemcomitans (Kieselbach et al., Citation2015), V. cholera (Altindis et al., Citation2014) and E. coli Nissle (Aguilera et al., Citation2014) have revealed novel insights into the protein cargo carried by these vesicles.
In this review, we focus on the OMVs produced by gram-negative bacteria. We provide an overview of the OMV structure, various factors regulating their production and their role in modulating host immune response. In light of the importance of the diverse cargoes carried by OMVs, we discuss the advances in the high-throughput detection of these OMVs and application in vaccine development.
OMVs: genesis from bacterial outer membrane and their structure
Outer membrane vesicles are derived from bacterial outer membranes entrapping the periplasmic content along with fragments of PG and nucleic acids occasionally. The bacterial OM is unique for its asymmetrical distribution of lipid moieties, wherein the exterior leaflet is enriched in lipopolysaccharides (LPS), and the inner leaflet predominantly contains glycerophospholipid (see ) (Roier et al., Citation2016; Schwechheimer & Kuehn, Citation2015). Endotoxin or lipid-A, a component of LPS, decorates the OM and is responsible for the immune modulation during pathogenic invasions (Raetz & Whitfield, Citation2002). During the vesicle formation, the asymmetry of the OM is retained in OMVs. The spherical structure of OMVs provides a higher surface-to-volume ratio, therefore allowing the residence of LPS in high density. LPS plays a major role in host-pathogen interplay and modulation of host immunity.
Figure 1. (A) Structure of the gram-negative bacterial cell envelope. The envelope consists of two lipid bilayers and a peptidoglycan layer. The bacterial outer membrane (OM) is unique for its asymmetrical distribution of lipid moieties, wherein the exterior leaflet is enriched in lipopolysaccharides (LPS), and the inner leaflet predominantly contains glycerophospholipid. The inner membrane (IM) is symmetrical in the glycerophospholipid composition in both the leaflets and contains many complex protein assemblies like the YrbDE, Tol component of the Tol-Pal system and NlpA. The region between IM and OM is called as the periplasmic space (PS). The periplasm contains the peptidoglycan (PG) layer along with other protein assemblies such as VacJ/YrbC, Tol B and Pal component of the Tol-Pal system, and Lipoma preferred partner (Lpp). The rest of the Yrb complex components (YrbBF) are juxta-membrane from the cytoplasmic face of IM. (B) The building blocks of the LPS layer. An LPS moiety is composed of the inner and outer core oligosaccharides followed by variable length O-antigens covalently linked to the outer-core. The inner core an LPS moiety contains l-glycerol-d-manno-heptopyranose (l-d-Hep) and Kdo as the major components while the outer core contains d-glucose as the major component linked with l-d-Hep and d-galactose monomers (see text for more details).
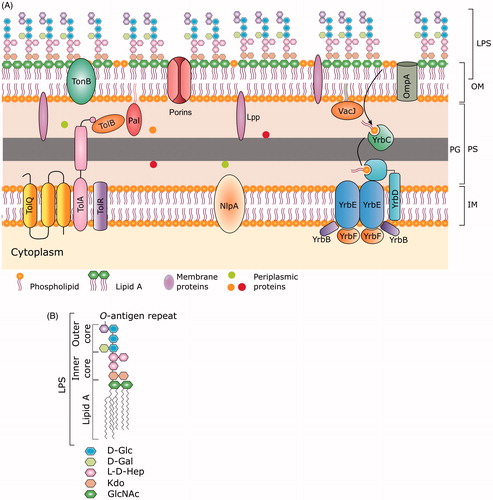
Lipopolysaccharides is synthesized on the inner membrane (IM) by the “Raetz-pathway” which is a nine-step process (Raetz & Whitfield, Citation2002; Whitfield & Trent, Citation2014). The enzymes involved in LPS synthesis extend between the IM and OM of the bacterial cell wall. LPS synthesis is initiated by a group of Lpx enzymes, present on the cytoplasmic face of the IM by the formation of a precursor called kdo2-lipid A (see ). Kdo2-lipid A is modified by the addition of core-oligosaccharide (genesis of LPS), and this is followed by flipping to the periplasmic face. The major population of lipid A moieties are further modified by ligation of O-antigen units of various lengths (see ). Finally, the LPS moiety is exported to the OM [reviewed in Whitfield & Trent (Citation2014), Raetz & Whitfield (Citation2002)]. Out of these steps, the ligation of O-antigen confers variability to the structure of LPS (see ), and these differential modifications cater to the need of the bacterium to adapt to its local environment (Kulp & Kuehn, Citation2010; Sohlenkamp & Geiger, Citation2016). For example, OMVs produced by P. aeruginosa in biofilms are different in their composition with respect to both lipids and protein (Metruccio et al., Citation2016). LPS synthesis is conserved in gram-negative bacteria, and there are no enzyme homologs in eukaryotes. Complete lack of such pathways in eukaryotes renders various steps in the LPS synthesis pathway as a potent drug target.
shows the schematic representation of the structure of a typical OMV () and its formation from bacterial OM (). The composition of the lipid bilayer of OMVs retains the asymmetry observed in bacterial OM, with complex lipids in the outermost layer mounted on a uniform glycerophospholipid inner layer. Recently, a few examples of double bilayer OMVs have been observed in Bacillus anthracis (Rivera et al., Citation2010), Shewanella vesiculosa M7T (Perez-Cruz et al., Citation2013), Ahrensia kielensis, and Pseudoalteromonas marina (Hagemann et al., Citation2013) whose detailed discussion is not in the scope of this review.
Figure 2. The structure (A) and production of OMVs from gram-negative bacteria (B). The composition of the lipid bilayer of OMVs retains the asymmetry observed in bacterial OM, with complex lipids in outermost layer mounted on a uniform phospholipid inner layer. The outermost layer of OMVs consists of the LPS layer (made up of lipid A, LPS core, and O-antigen) derived from the bacterial OM (see for details of bacterial OM). OMVs host a large repertoire of cargoes such as nucleic acids, enzymes, toxins, fragments of peptidoglycans, and fluid from the periplasmic space. (B) Formation of OMV starts with the bulging of the outer membrane at very small region followed by progress in the expansion of the membrane. The increased expansion is established with the spherical structure of blebs. Eventually vesicle pinches off at the joint neck and gets separated from the cell.
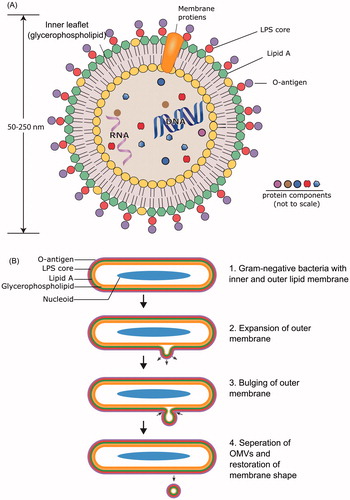
Proteomics analysis of different OMVs has revealed integral OM proteins, lipoproteins, periplasmic proteins, integral IM proteins and cytoplasmic proteins (Aguilera et al., Citation2014; Altindis et al., Citation2014; Berleman et al., Citation2014; Choi et al., Citation2011; Jang et al., Citation2014; Kahnt et al., Citation2010; Kieselbach et al., Citation2015; Kulkarni et al., Citation2014; Lappann et al., Citation2013; Mullaney et al., Citation2009). In addition to proteins, linear and circular DNA are also entrapped inside the OMVs produced from P. aeruginosa (Deatherage et al., Citation2009; Kadurugamuwa & Beveridge, Citation1995; Renelli et al., Citation2004), A. kielensis, P. marina (Hagemann et al., Citation2014) and E. coli O157:H7 (Deatherage et al., Citation2009). Recently, OMV-associated RNA has been derived from the enteric gram-negative bacteria E. coli K-12 (Ghosal et al., Citation2015), P. aeruginosa (Koeppen et al., Citation2016) and V. cholerae (Sjostrom et al., Citation2015). A large repertoire of toxins such as cytolethal distending toxin from A. actinomycetemcomitans (Rompikuntal et al., Citation2012), heat-labile enterotoxin (LT) from Enterotoxigenic E. coli (ETEC) (Horstman & Kuehn, Citation2000), Shiga toxin from enterohemorrhagic E. coli (EHEC) (Bielaszewska et al., Citation2017; Kolling & Matthews, Citation1999), and lethal and edema factor toxins from Bacillus anthracis (Rivera et al., Citation2010) are also encapsulated in the OMVs.
Outer membrane vesicles are produced by the expansion and bulging of the OM followed by pinching of the OM to form spherical vesicles. Different inducers of OMV production are discussed in the next section.
Regulatory mechanisms of OMV formation
Various mechanisms of production of OMVs have been proposed in the last four decades (Schwechheimer & Kuehn, Citation2015). provides an overview of the different mechanisms involved in OMV production. Production of OMVs is regulated at different physiological stages of bacterial cell growth. Membrane proteins like Lpp and OmpA, located in the periplasmic space (see ), span the gap between the OM and PG. Loss of association between OM and PG by a reduction in synthesis of Lpp or OmpA, or alteration in PG structure (reducing the binding of these proteins to PG layer), enhances OMV formation (Moon et al., Citation2012; Schwechheimer & Kuehn, Citation2015; Schwechheimer et al., Citation2014). This suggests that the function of these proteins as crosslinkers between OM and PG restrict the initiation of bulging of OM to produce OMVs (Moon et al., Citation2012; Schwechheimer & Kuehn, Citation2015; Schwechheimer et al., Citation2014). Interestingly, an IM lipoprotein gene nlpA (see ) deletion was found to reduce OMV production. However, the deletion of ompA or lpp genes enhanced the production of OMVs (Schwechheimer & Kuehn, Citation2015; Schwechheimer et al., Citation2014). Stress inducing factors like osmotic-shock brought about by high saline conditions, dehydration caused by long-chain alcohol, heat-shock (Baumgarten et al., Citation2012; McBroom & Kuehn, Citation2007), antibiotic stress (Maredia et al., Citation2012) and accumulation of misfolded proteins or components of PG (Hayashi et al., Citation2002; Nakae, Citation1975) are potent inducers of OMV production. Short fragments of PG are produced from interrupted biosynthesis or imbalance between synthesis and degradation (Hayashi et al., Citation2002). Local accumulation of these unwanted products interacts with the membrane and causes bulging that eventually turns into vesicles. Stress induced vesiculation provides benefits to the resident bacteria. For example, hyper-vesiculating E. coli mutants survive better against ethanol stress or the OM-damaging antimicrobial peptide polymyxin B (McBroom & Kuehn, Citation2007). Another proposed function of OMV production is to shed overproduced LPS load to maintain the membrane integrity (Bonnington & Kuehn, Citation2016), which has been reviewed earlier (Schwechheimer & Kuehn, Citation2015).
Table 1. Regulatory mechanisms of OMV formation.
A gram-negative facultative anaerobe, Shewanella oneidensis, shows hyper-vesiculation upon deletion of the degQ gene (Ojima et al., Citation2015). The degQ gene product is a periplasmic protease involved in the degradation of periplasmic proteins. Deletion of degQ gene leads to protein accumulation in the periplasmic space, which in turn enhances OMV formation.
The Tol-Pal system (see ) is an IM protein complex involved in the uptake of iron siderophores, type-A colicins and single stranded DNA phages (Letain & Postle, Citation1997). The Tol-Pal system consists of 5 proteins TolA, TolB, TolR, TolQ, and Pal. The tolA, tolR and tolQ genes are part of an operon, and their deletion causes a significant increase in vesiculation (Bernadac et al., Citation1998). This suggests the important role of TolA, TolQ, and TolR in regulating OMV formation. In the case of Serratia marcescens, a gram-negative opportunistic pathogen, the production of OMV is temperature-regulated. Higher amount of OMV is produced at 22 or 30 °C whereas reduction in production is observed at 37 °C (McMahon et al., Citation2012).
Cells growing at a lower temperature (∼22 or 30 °C) produce higher levels of OMVs compared to the cells grown at 37 °C. Enhancement in OMV production under thermal stress was due to reduced production of Enterobacterial common antigen (ECA), a glycerophospholipid found in the outer leaflet of OM. This reduction in the ECA was linked to RcsB phosphorelay signaling. Thermal stress causes RcsB signaling activation and reduced transcription of the ECA gene. Eventually, reduced membrane integrity leads to increased OMV production (McMahon et al., Citation2012).
Another proposed mechanism for inducing OMV production involves the phospholipid transporter VacJ-Yrb ABC (ATP-binding cassette) system located in the bacterial IM (see ). Low levels of VacJ-Yrb components were shown to increase OMV production by increased accumulation of curvature‐inducing phospholipids in the bacterial OM (Roier et al., Citation2016).
Lipid-A modifications play an important role in OMV production. The enzyme lipid-A deacylase called PagL modifies lipid-A by deacylation. The accumulation of deacylated lipid-A on the outer leaflet creates positive curvature leading to increased vesiculation (Elhenawy et al., Citation2016).
High-throughput detection of OMVs
Outer membrane vesicles were first discovered and characterized by transmission-electron microscopy (Chatterjee & Das, Citation1967, Citation1966; Work et al., Citation1966). In the past, OMVs were purified by filtration, ultracentrifugation, and characterized by their protein or lipid content. In these processes, the quantitative detection of OMV size and number was difficult to achieve with high precision. In view of the knowledge gathered from proteomics analysis of OMVs produced by a large group of bacteria and their relevance in immune modulation (see later), new methodologies of detection and characterization of OMVs are required. Recently, a novel method has been used for determination of the size and concentration of extracellular vesicles using tunable resistive pulse sensing (Vogel et al., Citation2016) which employs extrapolation of scanning ion occlusion spectroscopy (SIOS) which is based on the property of scalable membrane pores (i.e. screening by stretchability of membrane pores of different sizes) to analyze nano-sized particles (Roberts et al., Citation2010; Sowerby et al., Citation2007) (see ). As the particles of different size pass through the membrane pore, it induces changes in the current across the membrane by interfering with the open pore conductivity, which can be detected with high sensitivity and accuracy. The stretchable pores allow detection of a selective range of nano-sized OMVs. The data generated from the measurements can be used for high-throughput determination of vesicle size and number.
Figure 3. Quantitative high-throughput detection of OMVs using scanning ion occlusion spectroscopy (SIOS). Real-time scanning of the conductivity across the pore at different stretches and different pore size allows the discrimination of individual OMV population in a large disperse mixture. (A) Small and (B) large vesicles passing through the pore across the fluid chamber from a to b generate different conductivity signals. Larger vesicles require greater stretching of the pore to increase its diameter. This change in the pore size changes the strength of the current across the fluid chamber and is recorded as a signal of larger amplitude compared to smaller vesicles.
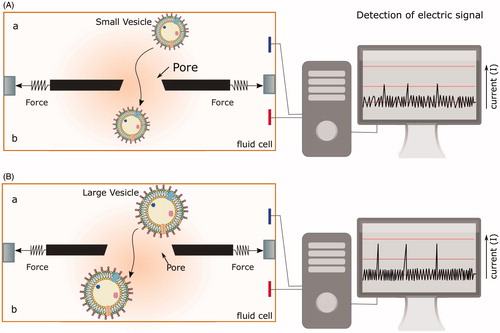
Mechanism of OMV entry into host cells
Outer membrane vesicles from various mucosal pathogens are majorly internalized by epithelial cells in the host, and this entry is mainly driven by two endocytic pathways: clathrin-mediated endocytosis, and the lipid raft-mediated pathway (Canas et al., Citation2016). summarizes the different pathways of entry of OMVs into the host cell. Vesicles from enterohemorrhagic E. coli, H. pylori, A. actinomycetemcomitans and Brucella abortus, enter host cells predominantly via clathrin-mediated endocytosis (Bielaszewska et al., Citation2013; Canas et al., Citation2016; Cheng et al., Citation2014; O'Donoghue & Krachler, Citation2016; Olofsson et al., Citation2014). Along with clathrin, dynamin has also been shown to play an important role in OMV internalization in the case of E. coli O104:H4 entry (Bielaszewska et al., Citation2017; Kunsmann et al., Citation2015). Lipid rafts are dynamic membrane microdomains rich in lipids like cholesterol and sphingolipids, and proteins such as caveolin. Vesicles from ETEC. coli, H. influenza (Kaparakis-Liaskos & Ferrero, Citation2015), P. gingivalis (Furuta et al., Citation2009), Moraxella catharralis (Schaar et al., Citation2011; Vidakovics et al., Citation2010), C. jejuni (Elmi et al., Citation2012) and P. aeruginosa are internalized through a lipid raft-mediated pathway (Bomberger et al., Citation2009; Furuta et al., Citation2009; Kesty et al., Citation2004). Lipid rafts enriched in GM1 act as molecular beacons for adhering to OMVs from ETEC and the bound complexes are internalized via lipid raft-mediated endocytosis (Ellis & Kuehn, Citation2010; Johnson et al., Citation2009; Horstman & Kuehn, Citation2002; Kesty & Kuehn, Citation2004).
Figure 4. Different modes of OMV entry into the host cell. OMVs can enter the host cells via two major pathways: clathrin-mediated endocytosis, and the lipid raft-mediated pathway. Lipid rafts are dynamic membrane microdomains rich in lipids like cholesterol and sphingolipids, and proteins such as caveolin. Pinocytosis and phagocytosis are other two general pathways involved in uptake of OMVs. The figure provides representative examples of different bacteria that have been shown to enter the host cell exploiting at least one of the above-mentioned pathways.
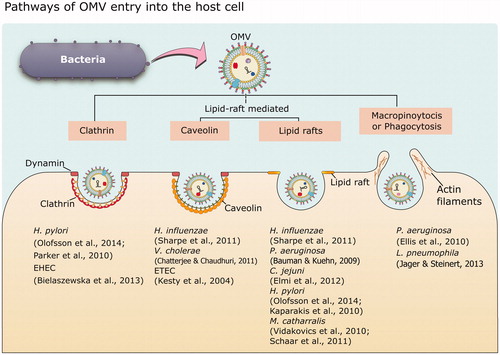
Bulk uptake of OMVs has been proposed through actin‐driven, ruffled protrusions from the cell membrane (Kaparakis-Liaskos & Ferrero, Citation2015; Kunsmann et al., Citation2015). Inhibition of actin polymerization by cytochalasin D or wiskostatin has been observed to reduce the entry of OMVs from P. aeruginosa into the epithelial cells lining the airway tract (Bomberger et al., Citation2009). Interestingly, a host factor called bactericidal/permeability-increasing protein (BPI) present on the surface of epithelial cells helps in regulating the internalization of OMV, e.g. in the case of N. meningitides OMVs, expression of BPI enhances the entry of OMVs into the host cells (Elsbach & Weiss, Citation1993).
The different pathways involved in OMV entry have been delineated by using a large repertoire of specific inhibitors/binders for the different components of each pathway, e.g. dynasore for dynamin, chlorpromazine for clathrin, filipin III, wortmannin, nystatin for lipid rafts and cytochalasin D for pinocytosis (Amano et al., Citation2010; Bauman & Kuehn, Citation2009; Bomberger et al., Citation2009; Canas et al., Citation2016; Olofsson et al., Citation2014; O'Donoghue & Krachler, Citation2016).
Host immune-modulation by OMVs and its potential application as vaccine
Outer membrane vesicles are known to carry cargoes such as endotoxins and RNA (discussed above), and these engage in dynamic interplay with the host immune system (Kuehn & Kesty, Citation2005). We discuss the host immune response of ETEC, Legionella pneumophila, N. meningitides and P. aeruginosa as representative examples in this review.
Enterotoxigenic E. coli colonizes the small intestine, where they produce heat-labile (LT, thermolabile) and/or heat-stable enterotoxins (ST, thermostable) (Kesty et al., Citation2004; Roy et al., Citation2011; Taxt et al., Citation2010). LT loaded OMVs are released into the intestine where they bind to the GM1 ganglioside receptors located on the host intestinal cell (Johnson et al., Citation2009; Spangler, Citation1992). This binding triggers the internalization of the OMV with toxin into the cell cytoplasm. LT acts through the ADP-ribosylation of the Gs subunit of adenylate cyclase, which results in an increase in cAMP levels. cAMP then activates protein kinase A (PKA), which phosphorylates the R domain of cystic fibrosis transmembrane conductance regulator (CFTR). CFTR is an essential component of epithelial Cl− transport systems. Phosphorylation of CFTR manifests as an increase in chloride and water efflux into the intestinal lumen leading to watery diarrhea. The pro-inflammatory response of LT-associated OMV purified from ETEC showed a significant immunogenic response. However, the response was slower compared to soluble LT (Chutkan & Kuehn, Citation2011). OMVs uptake by caveolin pathways and LT internalization by GM1 may lead to differences in the efficiency of the intracellular processing of LT and therefore the difference in response (Chutkan & Kuehn, Citation2011).
Legionella pneumophila¸ a causative agent of Legionnaire’s pneumonia, is a human- and amoebae-resident gram-negative bacterial pathogen. In humans, it infects alveolar macrophages (Jager et al., Citation2015) and propagates within the phagosome (Fields et al., Citation2002) by preventing phagosome maturation and fusion with the lysosomes (Fernandez-Moreira et al., Citation2006). L. pneumophila inhibits phagosome–lysosome fusion by secreting effector proteins into the cytoplasm via the type IV secretion system. This is followed by further modifications which transform the resident phagosome compartment into a vacuole, thereby enabling a protective replication niche (Ge and Shao, Citation2011; Hubber and Roy, Citation2010; Isberg et al., Citation2009). Recent reports show that OMVs produced from L. pneumophila have pro-inflammatory action on macrophages and epithelial cells (Galka et al., Citation2008; Jager et al., Citation2015). This is supported by the proteomics analysis of L. pneumophila OMVs which revealed many virulence factors among the 70 OMV-associated proteins identified (Jager et al., Citation2015).
Neisseria meningitides, another gram-negative pathogen, causes a severe human disease called meningitis. This infection compromises the protective membranes covering the brain and spinal cord leading to symptoms such as stiff neck, high fever, sensitivity to light, confusion, headaches, and vomiting (WHO, Citation2017). N. meningitides is an extracellular pathogen which is capable of inducing pyroptosis, a process that requires LPS interaction with caspase-11. Interestingly, the LPS released from OMVs encapsulated in endosomes interacts with caspase-11 in the cytosol. This interaction activates caspase-11 followed by acute inflammatory response and eventually sepsis (Vanaja et al., Citation2016).
Recently, miRNA-sized small RNAs (msRNAs) have been identified in OMVs secreted by P. aeruginosa. The msRNAs are expressed from bacterial cells and are packed into OMVs. Interestingly, these msRNAs could regulate the host gene expression and thereby modulate host immune response (Koeppen et al., Citation2016). This study has motivated identification of msRNA targets in eukaryotic genomes using bioinformatics tools (Choi et al., Citation2017).
Immunogenicity of OMVs has been a subject of an experimental vaccine and has culminated in many promising results against a number of bacterial infections (Acevedo et al., Citation2014; Asensio et al., Citation2011; Gaillard et al., Citation2014; Leitner et al., Citation2015; Mitra et al., Citation2016; Norheim et al., Citation2015; Park et al., Citation2011; Price et al., Citation2016; Roberts et al., Citation2008; van der Pol et al., Citation2015). Small size, immunogenic properties, and non-replicative behavior of OMVs make them a good choice for vaccination. OMVs have been tested for their ability to enhance host immunity and provide protection against pathogens. Recently, OMVs purified from a genetically engineered nonpathogenic strain expressing desired glycans of interest, were used for vaccination of mice and chickens to confer protection against Streptococcus pneumonae and C. jejuni infection, respectively (Price et al., Citation2016). Higher yield (∼60%) of bioengineered OMVs has been achieved by deletion of the tolA gene (part of the Tol-Pal system described earlier) in E. coli compared to its wild type strain (Bernadac et al., Citation1998). Similar enhancement in OMV production from Shigella boydii, a dysentery causative pathogen, has been observed by deletion of the tolA gene. Interestingly, immunization by OMVs purified from ΔtolA S. boydii provided enhanced protection against lethal dosage of S. boydii than offered by wild-type OMVs (Mitra et al., Citation2016).
A promising use of OMVs as a vaccine has been reported against pertussis (whooping cough), caused by bacterial pathogen Bordetella pertussis, which has a record of causing the deaths of ∼195,000 children annually (WHO, Citation2017). Intranasal immunization of mice with OMVs secreted by engineered B. bronchiseptica expressing the pagL gene (involved in OM modification, see ) to enhance OMV production, displayed reduced toxicity with a lower induction of proinflammatory cytokines. Exposure to engineered OMVs conferred around nine months of protection against Bordetella infection with a higher clearance of various B. pertussis isolates (Asensio et al., Citation2011; Gaillard et al., Citation2014).
Immunization by OMVs against Edwardsiella tarda infection holds great economic significance in the light of the loss caused by this infection in aquaculture globally. E. tarda often infects fishes, eels, and flounder causing Edwardsiellosis. The infection with E. tarda leads to exophthalmia, the loss of pigmentation, swelling of the abdominal surface, petechial hemorrhage on skin and fin, and rectal hernia. In addition, suppurative hepatitis, suppurative interstitial nephritis, and inflammation in the spleen are the primary histopathological diagnosis (Park et al., Citation2012). E. tarda infection can be transmitted through fecal contamination to amphibians, reptiles, and mammals (including humans) causing gastroenteritis and diarrhea (Vandepitte et al., Citation1983). Earlier studies have shown that E. tarda OM proteins can induce an immune response and are effective in the development of protection against E. tarda infection (Castro et al., Citation2008; Kawai et al., Citation2004; Kwon et al., Citation2006). This potential was realized in providing immunization to olive flounder using OMVs purified from E. tarda (Park et al., Citation2011).
Conclusions
Our understanding of OMVs has come a long way since their discovery in the ∼1960s. The urgency to understand the molecular details of OMV structure and function comes from the emerging dovetailed relationship between OMVs and biofilms (Wang et al., Citation2015). The merit for the urgency derives from the mammoth danger posed by microbial biofilms toward equipment damage, product contamination, energy losses and chronic infections (Wolcott et al., Citation2010; Yang et al., Citation2012). In this review, we summarize the genesis of OMVs from the host bacterial cell wall and present an overview of the different cues and genetic factors that induce OMV secretion. We discuss a new high-throughput technique to detect, quantitate and characterize the OMVs. We provide a brief outline of different pathways of entry of OMV into the host cells followed by host immune modulation by internalized OMVs, taking a few representative examples. OMVs have been clinically isolated from human blood and other external secretions like saliva and urine (Cheng et al., Citation2014). OMVs’ ability to elicit an immunogenic response has been utilized for vaccine development by engineering pathogenic bacteria to secrete OMVs with desired immunogenic properties. Taken together, exploration of the different aspects of OMVs is a rapidly growing field, and we present some of the recent insights and advances in our understanding of OMVs along with its application in the area of vaccine development.
Acknowledgements
D.A. thanks the Max-Planck-Institut für terrestrische Mikrobiologie for the award of a post-doctoral fellowship. Ar. C. gratefully acknowledges the support from Fulbright-Nehru post-doctoral fellowship. We acknowledge Patrick D. Ellis Fisher for proof reading the manuscript.
Disclosure statement
No potential conflict of interest was reported by the authors.
References
- Acevedo R, Fernandez S, Zayas C, et al. 2014. Bacterial outer membrane vesicles and vaccine applications. Front Immunol 5:121.
- Aguilera L, Toloza L, Gimenez R, et al. 2014. Proteomic analysis of outer membrane vesicles from the probiotic strain Escherichia coli Nissle 1917. Proteomics 14:222–229.
- Altindis E, Fu Y, Mekalanos JJ. 2014. Proteomic analysis of Vibrio cholerae outer membrane vesicles. Proc Natl Acad Sci U S A 111:E1548–E1556.
- Amano A, Takeuchi H, Furuta N. 2010. Outer membrane vesicles function as offensive weapons in host-parasite interactions. Microbes Infect 12:791–798.
- Asensio CJ, Gaillard ME, Moreno G, et al. 2011. Outer membrane vesicles obtained from Bordetella pertussis Tohama expressing the lipid A deacylase PagL as a novel acellular vaccine candidate. Vaccine 29:1649–1656.
- Bauman SJ, Kuehn MJ. 2009. Pseudomonas aeruginosa vesicles associate with and are internalized by human lung epithelial cells. BMC Microbiol 9:26.
- Baumgarten T, Sperling S, Seifert J, et al. 2012. Membrane vesicle formation as a multiple-stress response mechanism enhances Pseudomonas putida DOT-T1E cell surface hydrophobicity and biofilm formation. Appl Environ Microbiol 78:6217–6224.
- Berleman JE, Allen S, Danielewicz MA, et al. 2014. The lethal cargo of Myxococcus xanthus outer membrane vesicles. Front Microbiol 5:474.
- Bernadac A, Gavioli M, Lazzaroni JC, et al. 1998. Escherichia coli tol-pal mutants form outer membrane vesicles. J Bacteriol 180:4872–4878.
- Bielaszewska M, Ruter C, Bauwens A, et al. 2017. Host cell interactions of outer membrane vesicle-associated virulence factors of enterohemorrhagic Escherichia coli O157: Intracellular delivery, trafficking and mechanisms of cell injury. PLoS Pathog 13:e1006159.
- Bielaszewska M, Ruter C, Kunsmann L, et al. 2013. Enterohemorrhagic Escherichia coli hemolysin employs outer membrane vesicles to target mitochondria and cause endothelial and epithelial apoptosis. PLoS Pathog 9:e1003797.
- Bomberger JM, Maceachran DP, Coutermarsh BA, et al. 2009. Long-distance delivery of bacterial virulence factors by Pseudomonas aeruginosa outer membrane vesicles. PLoS Pathog 5:e1000382.
- Bonnington KE, Kuehn MJ. 2016. Outer membrane vesicle production facilitates LPS remodeling and outer membrane maintenance in Salmonella during environmental transitions. MBio 7.
- Brown L, Wolf JM, Prados-Rosales R, et al. 2015. Through the wall: extracellular vesicles in Gram-positive bacteria, mycobacteria and fungi. Nat Rev Microbiol 13:620–630.
- Canas MA, Gimenez R, Fabrega MJ, et al. 2016. Outer membrane vesicles from the probiotic Escherichia coli Nissle 1917 and the commensal ECOR12 enter intestinal epithelial cells via clathrin-dependent endocytosis and elicit differential effects on DNA damage. PLoS One 11:e0160374.
- Castro N, Toranzo AE, Nunez S, et al. 2008. Development of an effective Edwardsiella tarda vaccine for cultured turbot (Scophthalmus maximus). Fish Shellfish Immunol 25:208–212.
- Chatterjee D, Chaudhuri K. 2011. Association of cholera toxin with Vibrio cholerae outer membrane vesicles which are internalized by human intestinal epithelial cells. FEBS Lett 585:1357–1362.
- Chatterjee SN, Das J. 1966. Secretory activity of Vibrio cholerae as evidenced by electron microscopy. In Electron Microscopy. Tokyo: Maruzen Co. Ltd.**
- Chatterjee SN, Das J. 1967. Electron microscopic observations on the excretion of cell-wall material by Vibrio cholerae. J Gen Microbiol 49:1–11.
- Cheng L, Sharples RA, Scicluna BJ, et al. 2014. Exosomes provide a protective and enriched source of miRNA for biomarker profiling compared to intracellular and cell-free blood. J Extracell Vesicles 3.
- Choi DS, Kim DK, Choi SJ, et al. 2011. Proteomic analysis of outer membrane vesicles derived from Pseudomonas aeruginosa. Proteomics 11:3424–3429.
- Choi JW, Kim SC, Hong SH, et al. 2017. Secretable Small RNAs via outer membrane vesicles in periodontal pathogens. J Dent Res 96:458–466.
- Chutkan H, Kuehn MJ. 2011. Context-dependent activation kinetics elicited by soluble versus outer membrane vesicle-associated heat-labile enterotoxin. Infect Immun 79:3760–3769.
- Deatherage BL, Lara JC, Bergsbaken T, et al. 2009. Biogenesis of bacterial membrane vesicles. Mol Microbiol 72:1395–1407.
- Elhenawy W, Bording-Jorgensen M, Valguarnera E, et al. 2016. LPS remodeling triggers formation of outer membrane vesicles in Salmonella. MBio 7.
- Ellis TN, Kuehn MJ. 2010. Virulence and immunomodulatory roles of bacterial outer membrane vesicles. Microbiol Mol Biol Rev 74:81–94.
- Ellis TN, Leiman SA, Kuehn MJ. 2010. Naturally produced outer membrane vesicles from Pseudomonas aeruginosa elicit a potent innate immune response via combined sensing of both lipopolysaccharide and protein components. Infect Immun 78:3822–3831.
- Elmi A, Watson E, Sandu P, et al. 2012. Campylobacter jejuni outer membrane vesicles play an important role in bacterial interactions with human intestinal epithelial cells. Infect Immun 80:4089–4098.
- Elsbach P, Weiss J. 1993. The bactericidal/permeability-increasing protein (BPI), a potent element in host-defense against gram-negative bacteria and lipopolysaccharide. Immunobiology 187:417–429.
- Fernandez-Moreira E, Helbig JH, Swanson MS. 2006. Membrane vesicles shed by Legionella pneumophila inhibit fusion of phagosomes with lysosomes. Infect Immun 74:3285–3295.
- Fields BS, Benson RF, Besser RE. 2002. Legionella and Legionnaires’ disease: 25 years of investigation. Clin Microbiol Rev 15:506–526.
- Furuta N, Tsuda K, Omori H, et al. 2009. Porphyromonas gingivalis outer membrane vesicles enter human epithelial cells via an endocytic pathway and are sorted to lysosomal compartments. Infect Immun 77:4187–4196.
- Gaillard ME, Bottero D, Errea A, et al. 2014. Acellular pertussis vaccine based on outer membrane vesicles capable of conferring both long-lasting immunity and protection against different strain genotypes. Vaccine 32:931–937.
- Galka F, Wai SN, Kusch H, et al. 2008. Proteomic characterization of the whole secretome of Legionella pneumophila and functional analysis of outer membrane vesicles. Infect Immun 76:1825–1836.
- Ge J, Shao F. 2011. Manipulation of host vesicular trafficking and innate immune defence by Legionella Dot/Icm effectors. Cell Microbiol 13:1870–1880.
- Ghosal A, Upadhyaya BB, Fritz JV, et al. 2015. The extracellular RNA complement of Escherichia coli. Microbiologyopen
- Hagemann S, Stoger L, Kappelmann M, et al. 2014. DNA-bearing membrane vesicles produced by Ahrensia kielensis and Pseudoalteromonas marina. J Basic Microbiol 54:1062–1072.
- Hagemann S, Wohlschlaeger J, Bertram S, et al. 2013. Loss of Survivin influences liver regeneration and is associated with impaired Aurora B function. Cell Death Differ 20:834–844.
- Hayashi J, Hamada N, Kuramitsu HK. 2002. The autolysin of Porphyromonas gingivalis is involved in outer membrane vesicle release. FEMS Microbiol Lett 216:217–222.
- Horstman AL, Kuehn MJ. 2000. Enterotoxigenic Escherichia coli secretes active heat-labile enterotoxin via outer membrane vesicles. J Biol Chem 275:12489–12496.
- Horstman AL, Kuehn MJ. 2002. Bacterial surface association of heat-labile enterotoxin through lipopolysaccharide after secretion via the general secretory pathway. J Biol Chem 277:32538–32545.
- Hubber A, Roy CR. 2010. Modulation of host cell function by Legionella pneumophila type IV effectors. Annu Rev Cell Dev Biol 26:261–283.
- Isberg RR, O'Connor TJ, Heidtman M. 2009. The Legionella pneumophila replication vacuole: making a cosy niche inside host cells. Nat Rev Microbiol 7:13–24.
- Ismail S, Hampton MB, Keenan JI. 2003. Helicobacter pylori outer membrane vesicles modulate proliferation and interleukin-8 production by gastric epithelial cells. Infect Immun 71:5670–5675.
- Jager J, Steinert M. 2013. Enrichment of outer membrane vesicles shed by Legionella pneumophila. Methods Mol Biol 954:225–230.
- Jager J, Keese S, Roessle M, et al. 2015. Fusion of Legionella pneumophila outer membrane vesicles with eukaryotic membrane systems is a mechanism to deliver pathogen factors to host cell membranes. Cell Microbiol 17:607–620.
- Jang KS, Sweredoski MJ, Graham RL, et al. 2014. Comprehensive proteomic profiling of outer membrane vesicles from Campylobacter jejuni. J Proteomics 98:90–98.
- Johnson AM, Kaushik RS, Francis DH, et al. 2009. Heat-labile enterotoxin promotes Escherichia coli adherence to intestinal epithelial cells. J Bacteriol 191:178–186.
- Kadurugamuwa JL, Beveridge TJ. 1995. Virulence factors are released from Pseudomonas aeruginosa in association with membrane vesicles during normal growth and exposure to gentamicin: a novel mechanism of enzyme secretion. J Bacteriol 177:3998–4008.
- Kahnt J, Aguiluz K, Koch J, et al. 2010. Profiling the outer membrane proteome during growth and development of the social bacterium Myxococcus xanthus by selective biotinylation and analyses of outer membrane vesicles. J Proteome Res 9:5197–5208.
- Kaparakis M, Turnbull L, Carneiro L, et al. 2010. Bacterial membrane vesicles deliver peptidoglycan to NOD1 in epithelial cells. Cell Microbiol 12:372–385.
- Kaparakis-Liaskos M, Ferrero RL. 2015. Immune modulation by bacterial outer membrane vesicles. Nat Rev Immunol 15:375–387.
- Kawai K, Liu Y, Ohnishi K, et al. 2004. A conserved 37 kDa outer membrane protein of Edwardsiella tarda is an effective vaccine candidate. Vaccine 22:3411–3418.
- Kesty NC, Kuehn MJ. 2004. Incorporation of heterologous outer membrane and periplasmic proteins into Escherichia coli outer membrane vesicles. J Biol Chem 279:2069–2076.
- Kesty NC, Mason KM, Reedy M, et al. 2004. Enterotoxigenic Escherichia coli vesicles target toxin delivery into mammalian cells. Embo J 23:4538–4549.
- Kieselbach T, Zijnge V, Granstrom E, et al. 2015. Proteomics of Aggregatibacter actinomycetemcomitans outer membrane vesicles. PLoS One 10:e0138591.
- Koeppen K, Hampton TH, Jarek M, et al. 2016. A novel mechanism of host-pathogen interaction through sRNA in bacterial outer membrane vesicles. PLoS Pathog 12:e1005672.
- Kolling GL, Matthews KR. 1999. Export of virulence genes and Shiga toxin by membrane vesicles of Escherichia coli O157:H7. Appl Environ Microbiol 65:1843–1848.
- Kuehn MJ, Kesty NC. 2005. Bacterial outer membrane vesicles and the host-pathogen interaction. Genes Dev 19:2645–2655.
- Kulkarni HM, Swamy Ch V, Jagannadham MV. 2014. Molecular characterization and functional analysis of outer membrane vesicles from the antarctic bacterium Pseudomonas syringae suggest a possible response to environmental conditions. J Proteome Res 13:1345–1358.
- Kulp A, Kuehn MJ. 2010. Biological functions and biogenesis of secreted bacterial outer membrane vesicles. Annu Rev Microbiol 64:163–184.
- Kunsmann L, Ruter C, Bauwens A, et al. 2015. Virulence from vesicles: Novel mechanisms of host cell injury by Escherichia coli O104:H4 outbreak strain. Sci Rep 5:13252.
- Kwon SR, Nam YK, Kim SK, et al. 2006. Protection of tilapia (Oreochromis mosambicus) from edwardsiellosis by vaccination with Edwardsiella tarda ghosts. Fish Shellfish Immunol 20:621–626.
- Lappann M, Otto A, Becher D, et al. 2013. Comparative proteome analysis of spontaneous outer membrane vesicles and purified outer membranes of Neisseria meningitidis. J Bacteriol 195:4425–4435.
- Leitner DR, Lichtenegger S, Temel P, et al. 2015. A combined vaccine approach against Vibrio cholerae and ETEC based on outer membrane vesicles. Front Microbiol 6:823.
- Letain TE, Postle K. 1997. TonB protein appears to transduce energy by shuttling between the cytoplasmic membrane and the outer membrane in Escherichia coli. Mol Microbiol 24:271–283.
- Li ZS, Clarke AJ, Beveridge TJ. 1998. Gram-negative bacteria produce membrane vesicles which are capable of killing other bacteria. J Bacteriol 180:5478–5483.
- Lindmark B, Rompikuntal PK, Vaitkevicius K, et al. 2009. Outer membrane vesicle-mediated release of cytolethal distending toxin (CDT) from Campylobacter jejuni. BMC Microbiol 9:220.
- Maredia R, Devineni N, Lentz P, et al. 2012. Vesiculation from Pseudomonas aeruginosa under SOS. ScientificWorldJournal 2012:402919
- McBroom AJ, Kuehn MJ. 2007. Release of outer membrane vesicles by gram-negative bacteria is a novel envelope stress response. Mol Microbiol 63:545–558.
- McBroom AJ, Johnson AP, Vemulapalli S, et al. 2006. Outer membrane vesicle production by Escherichia coli is independent of membrane instability. J Bacteriol 188:5385–5392.
- McMahon KJ, Castelli ME, Garcia Vescovi E, et al. 2012. Biogenesis of outer membrane vesicles in Serratia marcescens is thermoregulated and can be induced by activation of the Rcs phosphorelay system. J Bacteriol 194:3241–3249.
- Metruccio MM, Evans DJ, Gabriel MM, et al. 2016. Pseudomonas aeruginosa outer membrane vesicles triggered by human mucosal fluid and lysozyme can prime host tissue surfaces for bacterial adhesion. Front Microbiol 7:871.
- Mirlashari MR, Hoiby EA, Holst J, et al. 2001. Outer membrane vesicles from Neisseria meningitidis: effects on tissue factor and plasminogen activator inhibitor-2 production in human monocytes. Thromb Res 102:375–380.
- Mitra S, Sinha R, Mitobe J, et al. 2016. Development of a cost-effective vaccine candidate with outer membrane vesicles of a tolA-disrupted Shigella boydii strain. Vaccine 34:1839–1846.
- Moon DC, Choi CH, Lee JH, et al. 2012. Acinetobacter baumannii outer membrane protein A modulates the biogenesis of outer membrane vesicles. J Microbiol 50:155–160.
- Mullaney E, Brown PA, Smith SM, et al. 2009. Proteomic and functional characterization of the outer membrane vesicles from the gastric pathogen Helicobacter pylori. Proteomics Clin Appl 3:785–796.
- Nakae T. 1975. Outer membrane of Salmonella typhimurium: reconstitution of sucrose-permeable membrane vesicles. Biochem Biophys Res Commun 64:1224–1230.
- Norheim G, Sanders H, Mellesdal JW, et al. 2015. An OMV vaccine derived from a capsular group B Meningococcus with constitutive feta expression: preclinical evaluation of immunogenicity and toxicity. PLoS One 10:e0134353.
- O'Donoghue EJ, Krachler AM. 2016. Mechanisms of outer membrane vesicle entry into host cells. Cell Microbiol 18:1508–1517.
- Ojima Y, Nguyen MH, Yajima R, et al. 2015. Flocculation of Escherichia coli cells in association with enhanced production of outer membrane vesicles. Appl Environ Microbiol 81:5900–5906.
- Olofsson A, Nygard Skalman L, Obi I, et al. 2014. Uptake of Helicobacter pylori vesicles is facilitated by clathrin-dependent and clathrin-independent endocytic pathways. MBio 5:e00979-14.
- Park SB, Aoki T, Jung TS. 2012. Pathogenesis of and strategies for preventing Edwardsiella tarda infection in fish. Vet Res 43:67–78.
- Park SB, Jang HB, Nho SW, et al. 2011. Outer membrane vesicles as a candidate vaccine against edwardsiellosis. PLoS One 6:e17629
- Parker H, Chitcholtan K, Hampton MB, et al. 2010. Uptake of Helicobacter pylori outer membrane vesicles by gastric epithelial cells. Infect Immun 78:5054–5061.
- Perez-Cruz C, Carrion O, Delgado L, et al. 2013. New type of outer membrane vesicle produced by the Gram-negative bacterium Shewanella vesiculosa M7T: implications for DNA content. Appl Environ Microbiol 79:1874–1881.
- Price NL, Goyette-Desjardins G, Nothaft H, et al. 2016. Glycoengineered outer membrane vesicles: a novel platform for bacterial vaccines. Sci Rep 6:24931,
- Raetz CR, Whitfield C. 2002. Lipopolysaccharide endotoxins. Annu Rev Biochem 71:635–700.
- Renelli M, Matias V, Lo RY, et al. 2004. DNA-containing membrane vesicles of Pseudomonas aeruginosa PAO1 and their genetic transformation potential. Microbiology 150:2161–2169.
- Rivera J, Cordero RJ, Nakouzi AS, et al. 2010. Bacillus anthracis produces membrane-derived vesicles containing biologically active toxins. Proc Natl Acad Sci U S A 107:19002–19007.
- Roberts GS, Kozak D, Anderson W, et al. 2010. Tunable nano/micropores for particle detection and discrimination: scanning ion occlusion spectroscopy. Small 6:2653–2658.
- Roberts R, Moreno G, Bottero D, et al. 2008. Outer membrane vesicles as acellular vaccine against pertussis. Vaccine 26:4639–4646.
- Roier S, Zingl FG, Cakar F, et al. 2016. A novel mechanism for the biogenesis of outer membrane vesicles in gram-negative bacteria. Nat Commun 7:10515
- Rompikuntal PK, Thay B, Khan MK, et al. 2012. Perinuclear localization of internalized outer membrane vesicles carrying active cytolethal distending toxin from Aggregatibacter actinomycetemcomitans. Infect Immun 80:31–42.
- Roy K, Hamilton DJ, Munson GP, et al. 2011. Outer membrane vesicles induce immune responses to virulence proteins and protect against colonization by enterotoxigenic Escherichia coli. Clin Vaccine Immunol 18:1803–1808.
- Salkinoja-Salonen M, Nurmiaho EL. 1978. The effect of lipopolysaccharide composition on the ultrastructure of Pseudomonas aeruginosa. J Gen Microbiol 105:23–28.
- Schaar V, de Vries SP, Perez Vidakovics ML, et al. 2011. Multicomponent Moraxella catarrhalis outer membrane vesicles induce an inflammatory response and are internalized by human epithelial cells. Cell Microbiol 13:432–449.
- Schwechheimer C, Kuehn MJ. 2015. Outer-membrane vesicles from gram-negative bacteria: biogenesis and functions. Nat Rev Microbiol 13:605–619.
- Schwechheimer C, Kulp A, Kuehn MJ. 2014. Modulation of bacterial outer membrane vesicle production by envelope structure and content. BMC Microbiol 14:324
- Schwechheimer C, Sullivan CJ, Kuehn MJ. 2013. Envelope control of outer membrane vesicle production in gram-negative bacteria. Biochemistry 52:3031–3040.
- Sharpe SW, Kuehn MJ, Mason KM. 2011. Elicitation of epithelial cell-derived immune effectors by outer membrane vesicles of nontypeable Haemophilus influenzae. Infect Immun 79:4361–4369.
- Sjostrom AE, Sandblad L, Uhlin BE, et al. 2015. Membrane vesicle-mediated release of bacterial RNA. Sci Rep 5:15329
- Sohlenkamp C, Geiger O. 2016. Bacterial membrane lipids: diversity in structures and pathways. FEMS Microbiol Rev 40:133–159.
- Sowerby SJ, Broom MF, Petersen GB. 2007. Dynamically resizable nanometre-scale apertures for molecular sensing. Sens Actuat B-Chem 123:325–330.
- Spangler BD. 1992. Structure and function of cholera toxin and the related Escherichia coli heat-labile enterotoxin. Microbiol Rev 56:622–647.
- Taxt A, Aasland R, Sommerfelt H, et al. 2010. Heat-stable enterotoxin of enterotoxigenic Escherichia coli as a vaccine target. Infect Immun 78:1824–1831.
- van der Pol L, Stork M, van der Ley P. 2015. Outer membrane vesicles as platform vaccine technology. Biotechnol J 10:1689–1706.
- Vanaja SK, Russo AJ, Behl B, et al. 2016. Bacterial outer membrane vesicles mediate cytosolic localization of LPS and Caspase-11 activation. Cell 165:1106–1119.
- Vandepitte J, Lemmens P, de Swert L. 1983. Human Edwardsiellosis traced to ornamental fish. J Clin Microbiol 17:165–167.
- Vidakovics ML, Jendholm J, Morgelin M, et al. 2010. B cell activation by outer membrane vesicles-a novel virulence mechanism. PLoS Pathog 6:e1000724
- Vogel R, Coumans FA, Maltesen RG, et al. 2016. A standardized method to determine the concentration of extracellular vesicles using tunable resistive pulse sensing. J Extracell Vesicles 5:31242.
- Wang W, Chanda W, Zhong M. 2015. The relationship between biofilm and outer membrane vesicles: a novel therapy overview. FEMS Microbiol Lett 362:fnv117.
- Whitfield C, Trent MS. 2014. Biosynthesis and export of bacterial lipopolysaccharides. Annu Rev Biochem 83:99–128.
- WHO. 2017. Meningococcal disease. Available from: http://www.who.int/csr/disease/meningococcal/en/.
- WHO. 2017. Pertussis. Available from: http://www.who.int/immunization/topics/pertussis/en/.
- Wolcott RD, Rhoads DD, Bennett ME, et al. 2010. Chronic wounds and the medical biofilm paradigm. J Wound Care 19:45–46. 48-50, 52-3.
- Work E, Knox KW, Vesk M. 1966. The chemistry and electron microscopy of an extracellular lipopolysaccharide from Escherichia coli. Ann N Y Acad Sci 133:438–449.
- Yang L, Liu Y, Wu H, et al. 2012. Combating biofilms. FEMS Immunol Med Microbiol 65:146–157.
- Yaron S, Kolling GL, Simon L, et al. 2000. Vesicle-mediated transfer of virulence genes from Escherichia coli O157:H7 to other enteric bacteria. Appl Environ Microbiol 66:4414–4420.
- Zakharzhevskaya NB, Tsvetkov VB, Vanyushkina AA, et al. 2017. Interaction of Bacteroides fragilis toxin with outer membrane vesicles reveals new mechanism of its secretion and delivery. Front Cell Infect Microbiol 7:2.