ABSTRACT
The domestic yak was regarded as a valuable model to explore the molecular mechanism of high-altitude animal adaptation, including the extreme tolerance of starvation and cold stress, which is closely associated with specifically seasonal deposition and metabolism of subcutaneous adipose tissue. However, the key regulators for these processes remain largely unknown. Here, the yak adipogenesis model in vitro was established and liver kinase b1 (LKB1) was regarded as a key negative regulator involved in yak adipocytes lipid accumulation. First, the mRNA level of LKB1 in adipose tissue of yaks from the cold season is significantly higher than that of yaks from the warm season. Interestingly, overexpression of LKB1 significantly inhibited adipocytes lipid accumulation. Consistently, LKB1 overexpression dramatically suppressed mRNA levels of adipogenic transcriptional factors, including C/EBPα, C/EBPβ and PPARγ, and subsequently significantly decreased levels of lipid accumulation related genes (FABP4 and FAS) compared with those of control. In contrast, overexpression of LKB1 promoted thermogenic and lipolysis related genes in yak adipocyte, including PGC1α, PPARα, UCP1, ATGL, HSL and LPL. Therefore, these results would provide new insights to decipher the network for domestic yak adipose deposition, and LKB1 might be as a new molecular target for animal environmental-resistant breeding.
1. Introduction
The domestic yak (Bos grunniens), as an iconic symbol of high altitude, is highly adapted to living and can thrive at altitudes between 2000 and 5000 m above sea level (Zhang et al. Citation2018). The yak not only provides the necessary resources for Tibetans and other nomadic pastoralists in high-altitude environments, including meat, milk, transportation, dung for fuel and hides for tented accommodation (Hu et al. Citation2012; Qiu et al. Citation2012), but also is regarded as a precious model to explore the molecular mechanism of high-altitude species adaption to extreme environment (Lan et al. Citation2020). The yaks grow with seasonal cycles of strong in summer, fatty in autumn, thin in winter, and tired in spring due to the seasonal variations in the temperature and quantity of natural herbage (Zhang et al. Citation2020). In detail, yak deposited adipose tissue to store maximum energy as lipid droplets on lush pastures during the warmer season (May to September), while the lipid droplets were catabolized to protect from starvation and cold stress during the long cold season (October to April) (Zhang et al. Citation2020). Previous anatomical studies found that adipose tissue might play dominant roles for yaks undergoing these cycles and possess specific characteristics of adipose tissue development, fat deposition and lipid metabolism compared with low-altitude species (Ou Citation1990). Although greatly abundant reports revealed the regulatory networks of adipocyte differentiation and lipid metabolism in mouse or human in normal physiology status (Rosen and Spiegelman Citation2014), the regulators involved in adipose deposition and metabolism, contributing to the adaptability of high-altitude species to extreme environment, remain largely unknown. Also, this area is recently invoked as a particularly fascinating topic for animal environmental-resistant breeding. Thus, the establishment of an adipogenic cell model from high-altitude species in vitro and subsequent exploration of the molecular mechanisms modulating adipogenesis and metabolism are urgent, which would provide the essential material to elucidate the molecular mechanism of adipose tissues development for plateau animals.
When people talk about fat, they usually refer to the two classic types of fat, namely white adipose tissue (WAT) and brown adipose tissue (BAT) (Chu and Gawronska-Kozak Citation2017). Of them, BAT mainly consumes fat and converts energy into heat through mitochondrial uncoupling protein 1 (UCP1), which plays an important role in adaptive thermogenesis. On the contrary, WAT mainly stores energy in the form of triglycerides (TG), leading to excessive expansion of white adipocytes, which in turn induce inflammation through paracrine and endocrine pathways (Saely et al. Citation2012; Chu and Tao Citation2017). The liver kinase b1 (LKB1), commonly named as serine/threonine kinase 11 (Stk11), is initially identified as a tumour suppressor mutated in Peutz–Jeghers syndrome (Hemminki et al. Citation1998). LKBI was reported to be involved in various biological processes, such as cellular energy metabolism (Nakada et al. Citation2010), skeletal muscle development (Shan et al. Citation2014), peripheral neuropathy (Xiong et al. Citation2017) and cancer differentiation and metastasis (Ji et al. Citation2007). Research show miR-424 promotes bovine adipogenesis through an unconventional post-transcriptional regulation of STK11 (Wang et al. Citation2020a, Citation2020b). Previously, knockout (KO) mouse mediated by Fabp4-Cre revealed that ablation of Lkb1 exhibited a reduced amount of white adipose tissue (WAT), postnatal growth retardation and early death before weaning (Zhang et al. Citation2013). However, adiponectin-Cre specifically lacks Lkb1 in WAT and brown adipose tissue (BAT), which induced BAT amplification, but not WAT (Shan et al. Citation2016). This is due to the mTOR pathway playing an active role in regulating fat mass and adipocyte size, whereas loss of Lkb1 enhances mTOR signaling only by reducing phosphorylation levels of AMPK in brown fat (Wang et al. Citation2017). These studies indicated that Lkb1 is involved in animal adipose deposition and metabolism, but the bona fide roles are distinct depending on adipose depots, physiologic status and cell models. For high-altitude species, the living environment is extreme cold, and it was reported that the deposition content and metabolic capability of adipose tissue were regarded as an important factor contributing to their cold adaption (Xie Citation2019). Moreover, given that the LKB1 expression in adipose tissue from cold-season yaks was significantly higher than that of warm-season yaks in a preliminary experiment. Thus, the LKB1 sequence of yak was first cloned by our group (Lei et al. Citation2019); however, its characteristics and exact function in yaks adipogenesis and metabolism were still unclear.
Here, the yak adipogenesis model in vitro was first constructed and then the expression patterns of yak LKB1 in adipose tissues and during adipogenic differentiation were measured. Functionally, overexpression of LKB1 mediated by adenovirus was carried out to explore its function at morphological and molecular levels by Oil red O staining and qPCR, respectively. Following these experiments, an adipocytes model utilizing to exploration adaption of high-altitude animals from the aspect of adipose deposition would be provided, and the position of LKB1 in the function of yak adipocyte adipogenesis shall be illuminated.
2. Materials and methods
2.1. Animals and sampling
The <7-day-old yak calves and 4-year-old adult yaks were purchased from Qinglin Town Yak Farm in Jinchuan County, Aba Tibetan and Qiang autonomous prefecture, Sichuan Province. All calves were sacrificed by CO2 asphyxiation to collect inguinal subcutaneous adipose tissue for isolation of preadipocytes. The adult yaks were slaughtered to sample subcutaneous adipose tissue at the end of warm (30 September) and cold seasons (30 April). The animal procedure was conducted in accordance with the Institutional Animal Care and Use Committee, Southwest Minzu University (Chengdu, Sichuan, China), and the experimental animal handling licence number SYXK 2019-216. Also, all the animal experiments complied with the requirements of the directory of the Ethical Treatment of Experimental Animals of China.
2.2. Isolation and adipogenic induction of domestic yak preadipocytes
Yak preadipocytes were isolated using collagenase digestion (Khan et al. Citation2019; Wang et al. Citation2020a, Citation2020b) and followed by density separation as in our previous research with minor changes (Xiong et al. Citation2018; Xu et al. Citation2020). Briefly, the fresh yak subcutaneous adipose tissue was minced into cubes with a size of ∼1 mm3 and digested in 1.5 mg/mL collagenase type I (Sigma, C0130) at 37°C for ∼1 h. The digestions were then terminated with DMEM containing 10% fetal bovine serum (FBS) and filtered through 100 µm filters to remove connective tissues and undigested trunks of tissues. Cells were then centrifuged at 1500 g for 5 min to separate the cells in the sediment and lipid-containing adipocytes in the floating layer. The freshly isolated preadipocytes were seeded and cultured in growth medium containing DMEM, 20% FBS, 1% penicillin/streptomycin (P/S) at 37°C with 5% CO2 for 12 h. Subsequently, the cells were washed with PBS 2–3 times, followed by feeding with fresh medium every 2 days.
The preadipocytes with 100% confluence were induced by induction medium (IM) containing DMEM, 10% FBS, 2.85 µM insulin (Sigma, I5523), 0.3 µM dexamethasone (DEXA, Sigma, D4902), 1 µM rosiglitazone (Sigma, R2408), and 0.63 mM 3-isobutyl-methylxanthine (IBMX, Sigma, 17018) for 3 days, and then were differentiated by differentiation medium (DM) containing DMEM, 10% FBS, 200 nM insulin and 10 nM T3 for 6 days until adipocytes mature. The medium was changed every 3 days during this adipogenic procedure.
2.3. Oil red O staining
The Oil red O staining was performed as previously described (Xiong et al. Citation2018). Briefly, cultured cells were washed with PBS 2–3 times and fixed with 4% formaldehyde for 15 min at room temperature. Then the cells were stained using the Oil Red O working solutions containing 6 ml Oil Red O stock solution (5 g/L in isopropanol) and 4 mL ddH2O for 20 min. After staining, the cells were washed with 60% isopropanol in PBS and pictured. Oil red O dye was extracted from stained adipocytes with 100% isopropanol, and the Oil red signal was quantified by measuring the optical density at 490 nm (OD 490).
2.4. Bodipy staining
For bodipy staining, the differentiated adipocytes were removed the DM and incubated by bodipy (final concentration: 2 μM) and Hoechst33342 (final concentration: 10 µg/mL) diluted in DM for 45 min. Then cells were washed with PBS 3 times, added fresh DM and took pictures. Fluorescent images were captured using a ZEISS LSM 800 confocal microscope. Images for control and treatment cells were captured using identical parameters and both groups’ images were adjusted identically in Image J.
2.5. Adenovirus amplification and infection
The adenovirus with yak LKB1 insertion was generated using the AdMax system as reported (Zhu et al. Citation2018), which has the advantages of high transfection efficiency, strong stability and high safety, compared with plasmid transfection direct (Zhang and Godbey Citation2006). Briefly, the CDS of LKB1 was cloned, inserted into the pHBAd plasmid, and verified by enzyme digestion and sequencing. The positive plasmid containing yak LKB1 was named as pHBAd-LKB1. Then, HEK293A cells (50%–60% confluent) in 6 cm culture dishes were co-transfected 4 µg backbone plasmid (pBHGlox(delta)E1, 3Cre) with 2 µg pHBAd-LKB1 or 2 µg pHBAd empty plasmid using Lipofectamine 2000 (Life Technologies) according to manufacturer’s protocol. After 2 weeks, the two strains of recombinant adenovirus were collected by three freezes–thaw–vortex cycles, named as LKB1-OE and Control, respectively. Two more round infected HEK293A cells were adapted to amplify the recombinant virus and the titres were determined by the expression of GFP. Adenovirus purification by CsCl2 grade ultra-centrifuge based on described procedure (Mueller et al. Citation2012). For overexpression, 1.5 × 105 cells were added per well in a 24-well plate and were infected by 10 µL (1 × 1010 PFU/ml) of the control and LKB1-OE adenovirus at 50% confluence. After incubation for 8 h, the adenovirus was changed by fresh growth medium. The cells were collected at 48 h post-infection to monitor the overexpression efficiency and at day 9 post-induction to assess the influence of adipogenic differentiation for the yak LKB1 gene.
2.6. Total RNA extraction and quantitative real-time PCR (qPCR)
Total RNA was extracted from adipocytes or adipose tissue using Trizol reagent according to the manufacturer’s instructions. RNA was treated with RNase-free DNase I to remove genomic DNA. The purity and concentration of total RNA were measured by Nanodrop 3000 (Thermo Fisher). Ratios of absorption (260/280 nm) of all samples were between 1.8 and 2.0. Then 3 μg of total RNA were reversed transcribed using random primers and Moloney murine leukaemia virus reverse transcriptase. qPCR was carried out with a Bio-Rad CFX96 PCR System using SYBR Green Master Mix and gene-specific primers (Table S1). The 2−ΔΔCT method was used to analyse the relative changes of gene expression normalized against 18S rRNA as the internal control.
2.7. Bioinformatics analysis
Nucleotide and acid sequence were analysed using the NCBI BLAST module and the DNAManv8.0.8.798 software for homology comparison. Besides, the LKB1 protein domain analysis using ProtPram online programme (https://web.expasy.org/protparam/) software.
2.8. Statistical analysis
The data are presented as means ± S.E.M. from independent experiments in one experiment. Comparisons were made by unpaired Student’s t-test using SPSS 17.0 software (SPSS Science, Chicago, IL, U.S.A.). Effects were considered significant at P < 0.05.
3. Results
3.1. Establishment of yak preadipocytes differentiation into a mature adipocyte model
Preadipocytes, isolated from adipose tissue, were first discovered by Rodbell and Jones (Citation1966), which had the ability to differentiate into mature adipocytes (Fu et al. Citation2013). Given that the absence of yak immortalized preadipocytes, the primary preadipocytes from yak subcutaneous adipose tissues were isolated through the avenue of collagenase Type I digestion. As shown in (A), the morphology of attached cells in wells resembled that of fibroblasts, with shapes of fusiform or triangle at 24 h post-seeded. It was noticeable observation that the proliferation capacity of yak preadipocytes presented a logarithmic increase trend, reaching to ∼50% of confluence at 72 h post-seeded ((B)). After seeding for 96 h, the cells completely covered culturing wells, reaching to 100% confluence ((C)), which then were subjected to the adipogenic induced procedure. This process was monitored by bodipy dye with detail, a green fluorescence probe being capable of uniquely labelling of neutral lipids ((D–G)). At day 0, the preadipocytes were not labelled by bodipy dye ((D)). After 3 days post-induction, a small number of cells with tiny lipid droplets surrounded nucleus in the cytoplasm ((E)). Subsequently, it was an obvious observation that more and more cells were loaded with lipid droplets during the stage of differentiation compared with those of the induction stage, in which the cells were maintained by differentiation medium ((F,G)). Furthermore, the lipid droplets in adipocytes were becoming larger with the elongation of differentiation maintenance ((F,G)). Specifically, some adipocytes were filled with a uniquely large lipid droplet at the terminal stage of adipogenesis, of which the nucleus moved to the edge of adipocytes ((G)). Consistent with the above morphology observation, the expression of delta-like non-canonical notch ligand 1 (DLK1), a marker gene of preadipocytes, dramatically decreased upon stimulation by induction medium and tended to be a decreasing trend during adipogenesis ((H)). In the process of adipocyte differentiation, C/EBPα and PPARγ are the core transcriptional regulators of adipocyte differentiation, and they can interact to promote adipocyte differentiation (Lane et al. Citation1999; Wang et al. Citation2020a, Citation2020b). As a secretory factor of adipocytes, ADIPOQ also gradually increases its expression with the differentiation of adipocytes, thereby improving insulin resistance (Hu et al. Citation1996). During the differentiation process of yak primary preadipocytes, these three genes all showed a gradual upward trend ((I–K)), elevating their mRNA levels of ∼7- ((I)), ∼6- ((J)) and ∼6000-fold changes ((K)) at day 9 to those of day 0 in contrast, respectively. Altogether, these data demonstrated that primary preadipocytes were capable of differentiation into mature adipocytes and the adipogenic differentiation model in yak was constructed correctly.
Figure 1. The establishment of yak adipogenesis model in vitro. (A,B) The morphology of isolated preadipocytes at 24 h (A) and 72 h (B) post-seeded. (C) The morphology of preadipocytes, reaching to 100% confluence, at which were treated by induction medium (IM). (D–G) The bodipy staining of adipocytes at day 0 (D), day 3 (E), day 6 (F) and day 9 (G) after adipogenic induction, blue fluorescence labelling of nucleus and green fluorescence labelling of lipid droplets, scale bar: 100 µm. Red arrows were presentative of mature adipocytes with uniquely large lipid droplets. (H–K) The mRNA levels of DLK1 (H), C/EBPα (I), PPARγ (J) and ADIPOQ (K) during preadipocyte adipogenic differentiation. N = 6, the number of samples are biological replicates. Error bars: S.E.M.
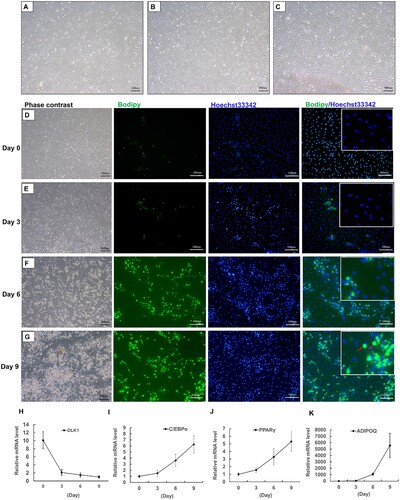
3.2. Expression patterns of LKB1 in yak adipose tissues and during preadipocytes adipogenesis
Previous studies have reported that there is a huge difference in the adaptability of yak to the environment compared with ordinary cattle. The adaptability of yak to the plateau environment may be related to genetic variation, blood oxygen, iron ion content and cell metabolism. These potential genetic variations may cause LKB1 to play a unique biological role in the production of yak fat cells (Ji et al. Citation2021). Thus, its sequence had been cloned by our group (Lei et al. Citation2019), and its characteristic was analysed by bioinformatics software. The nucleotide and amino acid sequence of LKB1 among species were performed homology alignment and data demonstrated that yak LKB1 nucleotide sequence had 78.10%, 84.28%, 84.44%, 86.83%, 86.94%, 90.17%, 90.74%, 90.89%, 92.65%, 97.80%, 97.87%, 99.70% of homology similarity to that of chicken, mouse, rat, human, macaque, cat, camel, pig, sperm whale, sheep, goat and cattle, respectively (Figure S1(A,D)). Consistently, yak LKB1 protein was totally identical to that of cattle, while the lowest homology similarity to that of mouse presented only 87.7% in contrast (Figure S1B). Moreover, protein domain analysis revealed that yak LKB1 protein contained three domains of nuclear localization signal (NLS) in the N-terminal (1st–54th), protein kinase domain (PDK) in the middle region (55th–309th) and posttranslational modification sites in the C-terminal (310th-438th) (Figure S1E). Specifically, the C-terminal sequence of LKB1 is highly variable among the aforementioned species (Figure S1C), which was involved in the regulation of protein interaction dynamically and the induction of LKB1 translocation between cytoplasm and nucleus (Song et al. Citation2008; Xie et al. Citation2009; Liang et al. Citation2015; Song et al. Citation2019). These implied that a range of different functions could be triggered by LKB1 in fat deposition among various species. Therefore, the significance of researching the bona fide regulatory role of LKB1 in yak adipogenesis and metabolism is obvious.
Previously, it was reported dramatical significance of adipose tissue deposition content (Ou Citation1990) and gene expression profiles between warm- and cold-season yaks (Xie Citation2019). Thus, the mRNA level of LKB1 in yak subcutaneous adipose tissue from these two seasons was detected, which is significantly higher in cold-season yaks with a thin layer of subcutaneous fat than that of warm-season yaks with a thick layer of subcutaneous fat ((A)). Next, the aforementioned sequentially adipogenic adipocytes samples were utilized to measure the LKB1 expression in yak preadipocytes adipogenesis and data showed that the mRNA level of LKB1 gradually tended to increase during this biological process, with ∼10-fold change at day 9 post-induction to that of day 0 in contrast ((B)). Since the terminal differentiation stage of preadipocyte is forming mature adipocytes, and accumulating a large number of lipid droplets (Ali et al. Citation2013). Therefore, these data alluded to the LKB1 with key roles in yak adipose deposition and lipid metabolism.
Figure 2. The expression patterns of LKB1 in yak adipose tissues and during adipogenesis. (A) The mRNA level of LKB1 in subcutaneous adipose tissue from warm- (W) and cold-season (C) yaks. (B) The mRNA levels of LKB1 during preadipocytes adipogenic differentiation. N = 6, the number of samples are biological replicates. Error bars: S.E.M., **P < 0.01.
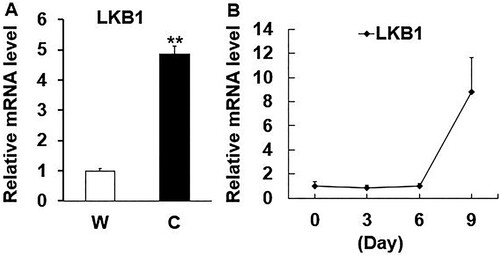
3.3. Gain-of-function LKB1 inhibits the domestic yak adipocyte lipid accumulation
To explore exact roles of LKB1 in yak adipogenesis, its gain-of-function mediated by adenovirus was performed according to the above adipocyte culturing procedure. Two strains of control and LKB1-OE adenovirus were, respectively, used to infect preadipocytes with 100% confluence, and highly infected efficiency was observed in both treatments, manifested by GFP positive cells ((A)). Consistently, the mRNA level of LKB1 increased significantly in the LKB1-OE group with ∼7-fold change of overexpression efficiency, in comparison with that of control ((B)). At day 9 post adipogenic induction, both groups were carried out Oil red O staining and it was noticeably observed that overexpression of LKB1 inhibited adipocyte differentiation, indicated by less Oil red O signal marked cells in the LKB1-OE group than that of control ((C)). In line with this, quantitative analysis of Oil red O dye showed that overexpression of LKB1 exhibited a significant decrease of the absorbance value at 490 nm to that of control in contrast ((D)), indicating the fewer lipid droplets in the LKB1-OE group. Altogether, these data demonstrated that overexpression of LKB1 suppresses the domestic yak adipocyte lipid accumulation.
Figure 3. Overexpression of LKB1 inhibits yak adipocyte lipid accumulation. (A) The GFP expression images in preadipocytes at 48 h post-infection by control and LKB1 overexpressed adenovirus captured by fluorescence microscope. (B) The overexpression efficiency of LKB1 in cells at 72 h post-infection detected by qPCR. (C) The Oil red O staining images of control and LKB1-OE adipocyte at day 9 post adipogenic induction. (D) The lipid content statistical analysis through measuring the absorbance of Oil red O signal at 490 nm. N = 6, the number of samples are biological replicates. Error bars: S.E.M., *P < 0.05. Scale bar: 100 μm.
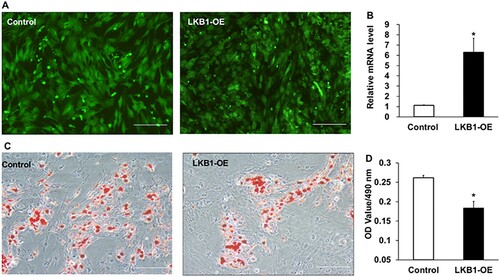
3.4. Overexpression of LKB1 downregulates mRNA levels of adipogenic genes in the domestic yak
In principle, the process of adipogenesis was contributed by the efficiency of preadipocyte differentiation into adipocyte and mature adipocytes lipid accumulation (Sarjeant and Stephens Citation2012). It was first coordinated by multiple transcriptional factors initiation of adipogenic differentiation, including CCAAT enhancer-binding protein beta (C/EBPβ), C/EBPα and PPARγ (Mota de Sa et al. Citation2017). qPCR analysis showed that overexpression of LKB1 dramatically downregulated both mRNA levels of C/EBPβ, C/EBPα and PPARγ, decreasing their levels by ∼40%, ∼70% and ∼50% to counterparts of the control in contrast ((A–C)), respectively. Subsequently, these transcription factors drive the expression of genes related to lipid accumulation. Fatty acid-binding protein 4 (FABP4), as a lipid molecular chaperone, participates in the transportation and targeting of fatty acids within cells, and can also regulate lipid synthesis by affecting genes related to cholesterol metabolism (Dou et al. Citation2020). FABP4 can interact with PPARγ and affect the formation of fat (Garin-Shkolnik et al. Citation2014). Fatty acid synthase (FAS) is an important rate-limiting enzyme for fatty acid regeneration. It catalyses the production of acetyl-CoA to produce long-chain fatty acids in the cytoplasm (Ronnett et al. Citation2005). Affect lipid synthesis, mutations in FAS can promote the browning of white fat, thereby reducing obesity (Choi et al. Citation2020). Interestingly, LKB1 overexpression extremely decreased the mRNA level of FABP4, with a ∼70% decrease to that of control ((D)). Similarity, the mRNA level of FAS had ∼50% lower than the counterpart of control ((E)). Additionally, it was extensively reported that adipose tissue not only stored excess energy as triglycerides, but also was regarded as paracrine/endocrine organ to produce adipokines, such as adiponectin (Adipoq), a well-known adipose tissue-specific adipokine (McGown et al. Citation2014; Tumminia et al. Citation2019). It was found that ∼80% mRNA level of ADIPOQ is lower in the LKB1-OE group than that of control ((F)). Hence, overexpression of LKB1 downregulates the expression of adipogenic genes in yak adipocytes.
Figure 4. Overexpression of LKB1 downregulates adipogenic genes in yaks. (A–F) The mRNA levels of C/EBPβ (A), C/EBPα (B), PPARγ (C), FABP4 (D), FAS (E) and ADIPOQ (F) in control and LKB1 overexpressed adipocytes. N = 6, the number of samples are biological replicates. Error bars: S.E.M., *P < 0.05, **P < 0.01.
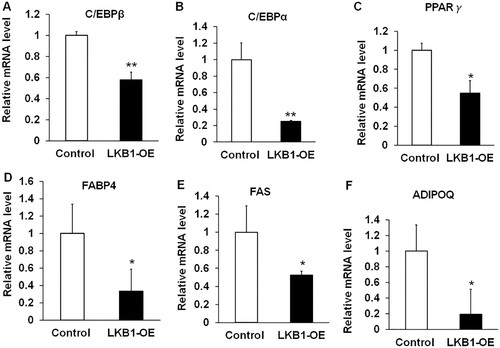
3.5. Overexpression of LKB1 upregulates thermogenic and lipolysis-related genes in the domestic yak
Given higher expression level of LKB1 in yak adipose tissue from the cold season, it was reported that yak subcutaneous adipose tissues with elevation expression levels of thermogenic and catabolic genes responded to cold stimulation in high-altitude areas (Xie Citation2019). Therefore, genes related to thermogenesis and lipolysis were detected in adipocytes overexpressing LKB1. UCP1 is a marker gene for classic brown fat cells or beige fat cells (Chu et al. Citation2014). When BAT is stimulated by thermogenesis, the UCP1 channel, located in the inner mitochondrial membrane, is opened to drive mitochondrial respiration to form H+ concentration, which prevents the formation of ATP and releases energy in the form of heat (Silva and Rabelo Citation1997). PPARα is highly expressed in the liver and brown adipose tissue, mainly by affecting the expression of the genes Acox1 and Ehhadh of the peroxisome β-oxidation rate-limiting enzyme, and then affecting the synthesis of fatty acids, which is dependent on carnitine palm transferase-1 (CPT-1) (Tang et al. Citation2020). PPARγ co-activator α (PGC1α) acts on mitochondria to activate the expression of UCP1 and induce thermogenesis (Queiroz et al. Citation2021). In this study, LKB1-OE significantly upregulated the mRNA levels of these thermogenic genes, including PGC1α, PPARα and UCP1, with ∼3-, ∼2.5- and ∼3-fold changes compared to the control, respectively ((A–C)). Previously research indicated that UCP1 is a marker gene of classical brown adipocytes or beige adipocytes, which catabolize and utilize the lipid droplets to produce heat against cold stress (Chouchani et al. Citation2019). Interestingly, the lipolysis genes, including adipose triglyceride lipase (ATGL) ((D)), hormone-sensitive lipase (HSL) ((E)) and lipoprotein lipase (LPL) ((F)), all were obviously upregulated in LKB1-OE cells, compared to those of control ((D–F)). These data suggested that overexpression of LKB1 in yak adipocytes promotes the expression of thermogenic and lipolysis-related genes.
Figure 5. Overexpression of LKB1 upregulates thermogenic and lipolysis-related genes in yaks. (A–F) The mRNA levels of PGC1α (A), PPARα (B), UCP1 (C), ATGL (D), HSL (E) and LPL (F) in control and LKB1 overexpressed adipocytes. N = 6, the number of samples are biological replicates. Error bars: S.E.M., * P < 0.05.
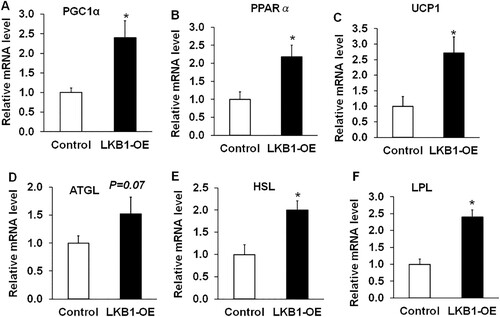
4. Discussion
Understanding the regulators regulating adipose tissue deposition and metabolism for high-altitude species is a particularly fascinating topic. In this study, we establish an efficient yak adipogenic adipogenesis model in vitro utilizing primary preadipocytes and report that LKB1 is a negative regulator for yak adipocyte lipid accumulation. Overexpression of LKB1 reduces lipid accumulation. Interestingly, gain-of-function LKB1 downregulates adipogenic genes and upregulates thermogenic and lipolysis-related genes. This research expands the growing knowledge of the regulatory network in yak adipogenesis and metabolism.
Yaks have numerous anatomical and physiological traits that equip them for life at high altitudes, such as high energy metabolism and seasonal fat deposition (Qiu et al. Citation2012; Zhou et al. Citation2017). Specifically, the yaks stored thick subcutaneous adipose tissue in the warm season and subsequently utilized fat against starvation and cold stress, which partly led to yaks with high tolerance to extreme environment adaption (Yang et al. Citation2017; Xie Citation2019). Thus, exploration of regulators involved in adipose tissue deposition in plateau animals would give new cues to environmental-resistant breeding. Additionally, it was recently reported that mouse or human responded to external prolonged cold stimulation in a manner of non-shivering heat production by brown adipocytes or beige adipocytes (Chouchani et al. Citation2019). The yaks live in high-altitude environment and survival during the long winters, even at −30∼40 degrees, which lead to forming specifically genetic mechanism to adapt to cold stimulation (Ji et al. Citation2020). Thus, the yak was also gradually regarded as a valuable animal material to explore the adaptive thermogenesis, which is separated into shivering and non-shivering forms, governed by skeletal muscle and adipose tissue, respectively (Chouchani et al. Citation2019). However, yak has a lower level of domestication, a higher acute sense of smell and stronger vigilance than other domestic animals (Wiener et al. Citation2003), which limits the application of yak as an animal model in vivo. Therefore, the alternative is the establishment of a yak adipocyte model in vitro.
In this work, we established the yak subcutaneous adipocyte adipogenesis model correctly. The isolated subcutaneous adipose tissue primary preadipocytes from calves (<7 days old) were capable of differentiation into mature adipocytes loaded by several large lipid droplets at day 9 post adipogenic induction, which was induced by a cocktail induction medium supplemented with IBMX, dexamethasone, insulin and rosiglitazone and then maintained in differentiation medium supplemented with insulin and T3. Also, the yak adipocytes expressed thermogenic specific genes in this procedure. Therefore, the established yak adipocytes could be a precious model to explore the function of adipogenesis and adaptive thermogenesis in mammals.
Gain-of-function LKB1 inhibits yak adipocyte differentiation and lipid accumulation, which were reported to be associated with adipocyte hyperplasia and hypertrophy, manifested by increasing adipocytes numbers and adipocyte lipid accumulation (Cohen and Spiegelman Citation2016). At the molecular level, the adipogenic differentiation involves a highly regulated and coordinated cascade of transcription factors that together lead to the establishment of the differentiated state (Rosen Citation2005). In the presence of the hormonal cocktail cues, committed preadipocytes express C/EBPβ and, in turn, induce the expression of C/EBPa and PPARγ (Rosen Citation2005; Mota de Sa et al. Citation2017). According to Oil red O staining, overexpression of LKB1 decreased the mRNA level of C/EBPβ, C/EBPa and PPARγ. Moreover, upon preadipocyte undergoing the differentiated state, the aforementioned transcriptional factors target fatty acid and triglyceride-related synthases, such as FABP4 and FAS (Sarjeant and Stephens Citation2012). We found that overexpressed LKB1 also inhibited mRNA levels of FABP4 and FAS, which were identified as the direct targets of C/EBPa (Mota de Sa et al. Citation2017). Thus, it is speculated that gain-of-function LKB1 suppresses yak adipogenesis possibly involved in stages of differentiation state determination and subsequently lipid accumulation.
Noticeably, overexpression of LKB1 inhibits yak adipogenic genes and lipid accumulation, but its expression tends to be an increasing trend during adipogenesis. This elevation of LKB1 expression level at the late stage of adipogenesis might be associated with its regulation on the thermogenesis of yak adipocytes. Two lines of evidence supported this explanation. First, the mRNA level of LKB1 was significantly higher in the subcutaneous adipose tissue from cold-season yaks than that of adipose tissue from warm-season, and Xie reported that yak subcutaneous adipose tissue exhibited a higher level of thermogenic genes in cold stimulation than that in warm environment (Xie Citation2019). Consistently, gain-of-function LKB1 upregulated thermogenic genes, including PGC1α, PPARα and UCP1. Of these, UCP1 was identified as the marker gene of classical brown or beige adipocytes (Ikeda and Yamada Citation2020). Beige adipocytes were founded scattered within subcutaneous white adipose tissue in response to prolonged cold exposure (Kulterer et al. Citation2020), drug stimulation (such as β-adrenergic agonist) (Burl et al. Citation2018) or genetic regulator (such as Notch1 and transferrin receptor 1) (Bi et al. Citation2014; Li et al. Citation2020). Studies have reported that the kinase activity of LKB1 is essential for lipid storage and controls the lipolysis pathway in the adipose tissue. The lipolysis defect caused by the LKB1 mutant can be rescued by expressing constitutively active SIK3 in the adipose tissue. LKB1 and SIK3 regulate the gene expression of Brummer to regulate lipid storage. Brummer is the Drosophila homolog of human fat triglyceride lipase (ATGL) and is a key lipolytic gene. This indicates that LKB1 may play an important role in regulating lipid metabolism (Choi et al. Citation2015). Hence, the elevation of LKB1 of cold-season yaks might result in beige adipocytes formation in the subcutaneous adipose tissue against extreme hypothermia stimulation.
Overall, yak LKB1 plays a distinct function in adipocytes lipid accumulation and metabolism in comparison with mouse. In yaks, gain-of-function LKB1 inhibits subcutaneous adipocyte lipid content, which is attributed to the inhibition of yak adipogenic differentiation and promotion of thermogenic capacity. Conversely, deletion of LKB1 in vivo promotes the expansion of brown fat only, but does not influence the white adipose deposition (Shan et al. Citation2016). This difference might be explained by yak subcutaneous adipocytes with characteristics of brown or beige adipocytes. In line with this speculation, the thermogenic genes could be detected in yak adipocytes and the Ct value is ∼27 by qPCR. In addition, it’s reported that ablation of LKB1 only reduces the phosphorylation level of AMPK (p-AMPK) in brown adipocytes, a central metabolic sensor (Shackelford and Shaw Citation2009), but not in white adipocytes (Shan et al. Citation2016; Xiong et al. Citation2017). Although AMPK is a classical substrate in mouse brown adipocytes and other cell types (Deng et al. Citation2019; Hollstein et al. Citation2019), the C-terminus of yak LKB1 protein sequence is variable in different species, which is associated with the regulation of protein interaction dynamically, inducing its translocation between cytoplasm and nucleus and subsequently performing its function (Song et al. Citation2008; Xie et al. Citation2009; Liang et al. Citation2015; Song et al. Citation2019). Thus, the molecular mechanism existing in the yak’s adipocytes needs to be verified in further study.
5. Conclusion
In conclusion, the yak adipogenic differentiation model is established in this study. We reveal the expression patterns of LKB1 in adipose tissues and during adipogenesis and report that gain-of-function yak LKB1 inhibits yak adipocytes lipid accumulation and promotion of thermogenic genes expression. These results not only provide a valuable adipocyte model for elucidation of high-altitude species adipogenesis and adaptive thermogenesis but expand the growing knowledge of the regulatory network in yak fat deposition and metabolism.
TAAR_2042001_Supplementarymaterial
Download Zip (6.6 MB)Disclosure statement
No potential conflict of interest was reported by the author(s).
References
- Ali AT, Hochfeld WE, Myburgh R, Pepper MS. 2013. Adipocyte and adipogenesis. Eur J Cell Biol. 92(6-7):229–236.
- Bi P, Shan T, Liu W, et al. 2014. Inhibition of Notch signaling promotes browning of white adipose tissue and ameliorates obesity. Nat Med. 20(8):911–918.
- Burl RB, Ramseyer VD, Rondini EA, Pique-Regi R, Lee YH, Granneman JG. 2018. Deconstructing Adipogenesis induced by beta3-Adrenergic receptor activation with single-cell expression profiling. Cell Metab. 28(2):300–309.e304.
- Choi EW, Lee M, Song JW, Kim K, Lee J, Yang J, Lee SH, Kim IY, Choi JH, Seong JK. 2020. Fas mutation reduces obesity by increasing IL-4 and IL-10 expression and promoting white adipose tissue browning. Sci Rep. 10(1):12001.
- Choi S, Lim DS, Chung J. 2015. Feeding and fasting signals converge on the LKB1-SIK3 pathway to regulate lipid metabolism in drosophila. PLoS Genet. 11(5):e1005263.
- Chouchani ET, Kazak L, Spiegelman BM. 2019. New advances in adaptive thermogenesis: UCP1 and beyond. Cell Metab. 29(1):27–37.
- Chu DT, Gawronska-Kozak B. 2017. Brown and brite adipocytes: same function, but different origin and response. Biochimie. 138:102–105.
- Chu DT, Malinowska E, Gawronska-Kozak B, Kozak LP. 2014. Expression of adipocyte biomarkers in a primary cell culture models reflects preweaning adipobiology. J Biol Chem. 289(26):18478–18488.
- Chu DT, Tao Y. 2017. Human thermogenic adipocytes: a reflection on types of adipocyte, developmental origin, and potential application. J Physiol Biochem. 73(1):1–4.
- Cohen P, Spiegelman BM. 2016. Cell biology of fat storage. Mol Biol Cell. 27(16):2523–2527.
- Deng Y, Jin F, Li X, et al. 2019. Sauchinone suppresses FceRI-mediated mast cell signaling and anaphylaxis through regulation of LKB1/AMPK axis and SHP-1-Syk signaling module. Int Immunopharmacol. 74:105702.
- Dou HX, Wang T, Su HX, Gao DD, Xu YC, Li YX, Wang HY. 2020. Exogenous FABP4 interferes with differentiation, promotes lipolysis and inflammation in adipocytes. Endocrine. 67(3):587–596.
- Fu S, Luan J, Xin M, Wang Q, Xiao R, Gao Y. 2013. Fate of adipose-derived stromal vascular fraction cells after co-implantation with fat grafts: evidence of cell survival and differentiation in ischemic adipose tissue. Plast Reconstr Surg. 132(2):363–373.
- Garin-Shkolnik T, Rudich A, Hotamisligil GS, Rubinstein M. 2014. FABP4 attenuates PPARgamma and adipogenesis and is inversely correlated with PPARgamma in adipose tissues. Diabetes. 63(3):900–911.
- Hemminki A, Markie D, Tomlinson I, et al. 1998. A serine/threonine kinase gene defective in Peutz-Jeghers syndrome. Nature. 391(6663):184–187.
- Hollstein PE, Eichner LJ, Brun SN, et al. 2019. The AMPK-related kinases SIK1 and SIK3 mediate key tumor-suppressive effects of LKB1 in NSCLC. Cancer Discov. 9(11):1606–1627.
- Hu E, Liang P, Spiegelman BM. 1996. Adipoq is a novel adipose-specific gene dysregulated in obesity. J Biol Chem. 271(18):10697–10703.
- Hu Q, Ma T, Wang K, Xu T, Liu J, Qiu Q. 2012. The Yak genome database: an integrative database for studying yak biology and high-altitude adaption. BMC Genomics. 13:600.
- Ikeda K, Yamada T. 2020. UCP1 dependent and independent thermogenesis in Brown and Beige Adipocytes. Front Endocrinol (Lausanne). 11:498.
- Ji H, Ramsey MR, Hayes DN, et al. 2007. LKB1 modulates lung cancer differentiation and metastasis. Nature. 448(7155):807–810.
- Ji QM, Xin JW, Chai ZX, et al. 2020. A chromosome-scale reference genome and genome-wide genetic variations elucidate adaptation in yak. Mol Ecol Resour.
- Ji QM, Xin JW, Chai ZX, et al. 2021. A chromosome-scale reference genome and genome-wide genetic variations elucidate adaptation in yak. Mol Ecol Resour. 21(1):201–211.
- Khan R, Raza SHA, Junjvlieke Z, Xiaoyu W, Garcia M, Elnour IE, Hongbao W, Linsen Z. 2019. Function and transcriptional regulation of bovine TORC2 gene in Adipocytes: roles of C/EBP, XBP1, INSM1 and ZNF263. Int J Mol Sci. 20(18):4338.
- Kulterer OC, Niederstaetter L, Herz CT, Haug AR, Bileck A, Pils D, Kautzky-Willer A, Gerner C, Kiefer FW. 2020. The presence of active brown adipose tissue determines cold-induced energy expenditure and oxylipin profiles in humans. J Clin Endocrinol Metab. 105:7.
- Lan D, Ji W, Xiong X, Liang Q, Yao W, Mipam TD, Zhong J, Li J. 2020. Population genome of the newly discovered Jinchuan yak to understand its adaptive evolution in extreme environments and generation mechanism of the multirib trait. Integr Zool. 16(5):685–695.
- Lane MD, Tang QQ, Jiang MS. 1999. Role of the CCAAT enhancer binding proteins (C/EBPs) in adipocyte differentiation. Biochem Biophys Res Commun. 266(3):677–683.
- Lei Z, Bai X, Lin Y, Li J, Zi X, Xiong X, Xiong Y. Coding region cloning of yak (Bos grunniens) Lkb1 gene and its expression analysis in skeletal muscle. J Agric Biotechnol. 27(01):71–79. 2019.
- Li J, Pan X, Pan G, et al. 2020. Transferrin receptor 1 regulates thermogenic capacity and cell fate in Brown/Beige Adipocytes. Adv Sci Weinh. 7(12):1903366.
- Liang HJ, Chai RC, Li X, Kong JG, Jiang JH, Ma J, Vatcher G, Yu AC. 2015. Astrocytic exportin-7 responds to ischemia through mediating LKB1 translocation from the nucleus to the cytoplasm. J Neurosci Res. 93(2):253–267.
- McGown C, Birerdinc A, Younossi ZM. 2014. Adipose tissue as an endocrine organ. Clin Liver Dis. 18(1):41–58.
- Mota de Sa P, Richard AJ, Hang H, Stephens JM. 2017. Transcriptional regulation of Adipogenesis. Compr Physiol. 7(2):635–674.
- Mueller C, Ratner D, Zhong L, Esteves-Sena M, Gao G. 2012. Production and discovery of novel recombinant adeno-associated viral vectors. Curr Protoc Microbiol. 14:11.
- Nakada D, Saunders TL, Morrison SJ. 2010. Lkb1 regulates cell cycle and energy metabolism in haematopoietic stem cells. Nature. 468(7324):653–658.
- Ou Y. 1990. Seasonal changes in some traits of yak (review). Ecol Demost Anim. 3:35–41.
- Qiu Q, Zhang G, Ma T, et al. 2012. The yak genome and adaptation to life at high altitude. Nat Genet. 44(8):946–949.
- Queiroz AL, Lessard SJ, Ouchida AT, et al. 2021. The MicroRNA miR-696 is regulated by SNARK and reduces mitochondrial activity in mouse skeletal muscle through Pgc1alpha inhibition. Mol Metab. 51:101226.
- Rodbell M, Jones AB. 1966. Metabolism of isolated fat cells. 3. The similar inhibitory action of phospholipase C (Clostridium perfringens alpha toxin) and of insulin on lipolysis stimulated by lipolytic hormones and theophylline. J Biol Chem. 241(1):140–142.
- Ronnett GV, Kim EK, Landree LE, Tu Y. 2005. Fatty acid metabolism as a target for obesity treatment. Physiol Behav. 85(1):25–35
- Rosen ED. 2005. The transcriptional basis of adipocyte development. Prostagland Leukot Essent Fatty Acids. 73(1):31–34.
- Rosen ED, Spiegelman BM. 2014. What we talk about when we talk about fat. Cell. 156(1–2):20–44.
- Saely CH, Geiger K, Drexel H. 2012. Brown versus white adipose tissue: a mini-review. Gerontology. 58(1):15–23.
- Sarjeant K, Stephens JM. 2012. Adipogenesis. Cold Spring Harb Perspect Biol. 4(9):a008417.
- Shackelford DB, Shaw RJ. 2009. The LKB1-AMPK pathway: metabolism and growth control in tumour suppression. Nat Rev Cancer. 9(8):563–575.
- Shan T, Xiong Y, Zhang P, et al. 2016. Lkb1 controls brown adipose tissue growth and thermogenesis by regulating the intracellular localization of CRTC3. Nat Commun. 7:12205.
- Shan T, Zhang P, Liang X, Bi P, Yue F, Kuang S. 2014. Lkb1 is indispensable for skeletal muscle development, regeneration, and satellite cell homeostasis. Stem Cells. 32(11):2893–2907.
- Silva JE, Rabelo R. 1997. Regulation of the uncoupling protein gene expression. Eur J Endocrinol. 136(3):251–264.
- Song P, Xie Z, Wu Y, Dong Y, Zou MH. 2019. Withdrawal: protein kinase Czeta-dependent LKB1 serine 428 phosphorylation increases LKB1 nucleus export and apoptosis in endothelial cells. J Biol Chem. 294(37):13831.
- Song P, Xie Z, Wu Y, Xu J, Dong Y, Zou MH. 2008. Protein kinase Czeta-dependent LKB1 serine 428 phosphorylation increases LKB1 nucleus export and apoptosis in endothelial cells. J Biol Chem. 283(18):12446–12455.
- Tang S, Wu F, Lin X, Gui W, Zheng F, Li H. 2020. The effects of new selective PPARalpha agonist CP775146 on systematic lipid metabolism in obese mice and Its potential mechanism. J Diabetes Res. 2020:4179852.
- Tumminia A, Vinciguerra F, Parisi M, Graziano M, Sciacca L, Baratta R, Frittitta L. 2019. Adipose tissue, obesity and adiponectin: role in endocrine cancer risk. Int J Mol Sci. 20:12.
- Wang G, Wu B, Zhang L, Cui Y, Zhang B, Wang H. 2020a. Laquinimod prevents Adipogenesis and obesity by down-regulating PPAR-gamma and C/EBPalpha through activating AMPK. ACS Omega. 5(36):22958–22965.
- Wang L, Zhang S, Zhang W, Cheng G, Khan R, Junjvlieke Z, Li S, Zan L. 2020b. miR-424 promotes bovine Adipogenesis through an unconventional post-transcriptional regulation of STK11. Front Genet. 11:145.
- Wang Y, Paulo E, Wu D, Wu Y, Huang W, Chawla A, Wang B. 2017. Adipocyte liver kinase b1 suppresses beige adipocyte renaissance through class IIa histone deacetylase 4. Diabetes. 66(12):2952–2963.
- Wiener G, Jianlin H, Ruijun L, Wiener G, Jianlin H, Ruijun L. 2003. The yak in relation to its environment.
- Xie F. 2019. The expression difference of genes related to brown adipose tissue of Yak and comparison of fatty acid composition in different seasons [Master]. Qinghai University. 83 pp.
- Xie Z, Dong Y, Zhang J, Scholz R, Neumann D, Zou MH. 2009. Identification of the serine 307 of LKB1 as a novel phosphorylation site essential for its nucleocytoplasmic transport and endothelial cell angiogenesis. Mol Cell Biol. 29(13):3582–3596.
- Xiong Y, Page JC, Narayanan N, et al. 2017. Peripheral neuropathy and Hindlimb paralysis in a mouse model of adipocyte-specific knockout of Lkb1. EBioMedicine. 24:127–136.
- Xiong Y, Yue F, Jia Z, Gao Y, Jin W, Hu K, Zhang Y, Zhu D, Yang G, Kuang S. 2018. A novel brown adipocyte-enriched long non-coding RNA that is required for brown adipocyte differentiation and sufficient to drive thermogenic gene program in white adipocytes. Biochim Biophys Acta Mol Cell Biol Lipids. 1863(4):409–419.
- Xu Q, Lin Y, Wang Y, Bai W, Zhu J. 2020. Knockdown of KLF9 promotes the differentiation of both intramuscular and subcutaneous preadipocytes in goat. Biosci Biotechnol Biochem. 84(8):1594–1602.
- Yang C, Ding X, Qian J, Wu X, Liang C, Bao P, Long R, Yan P. 2017. The progress of anato mical research of yaks adapt to Qinghai-Tibet Plateau environment. Chin J. Anim Sci. 3:18–24.
- Zhang W, Wang Q, Song P, Zou MH. 2013. Liver kinase b1 is required for white adipose tissue growth and differentiation. Diabetes. 62(7):2347–2358.
- Zhang X, Godbey WT. 2006. Viral vectors for gene delivery in tissue engineering. Adv Drug Deliv Rev. 58(4):515–534.
- Zhang Y, Guo X, Pei J, Chu M, Ding X, Wu X, Liang C, Yan P. 2020. CircRNA expression profile during Yak adipocyte differentiation and screen potential circRNAs for adipocyte differentiation. Genes. 11:4.
- Zhang Y, Wu X, Liang C, Bao P, Ding X, Chu M, Jia C, Guo X, Yan P. 2018. MicroRNA-200a regulates adipocyte differentiation in the domestic yak Bos grunniens. Gene. 650:41–48.
- Zhou JW, Zhong CL, Liu H, et al. 2017. Comparison of nitrogen utilization and urea kinetics between yaks (Bos grunniens) and indigenous cattle (Bos taurus). J Anim Sci. 95(10):4600–4612.
- Zhu HH, Wang XT, Sun YH, He WK, Liang JB, Mo BH, Li L. 2018. Pim1 overexpression prevents apoptosis in cardiomyocytes after exposure to hypoxia and oxidative stress via upregulating cell autophagy. Cell Physiol Biochem. 49(6):2138–2150.