Abstract
The diversity of actions of the glucocorticoid stress hormones among individuals and within organs, tissues and cells is shaped by age, gender, genetics, metabolism, and the quantity of exposure. However, such factors cannot explain the heterogeneity of responses in the brain within cells of the same lineage, or similar tissue environment, or in the same individual. Here, we argue that the stress response is continuously updated by synchronized neural activity on large-scale brain networks. This occurs at the molecular, cellular and behavioral levels by crosstalk communication between activity-dependent and glucocorticoid signaling pathways, which updates the diversity of responses based on prior experience. Such a Bayesian process determines adaptation to the demands of the body and external world. We propose a framework for understanding how the diversity of glucocorticoid actions throughout brain networks is essential for supporting optimal health, while its disruption may contribute to the pathophysiology of stress-related disorders, such as major depression, and resistance to therapeutic treatments.
1. Introduction
Glucocorticoid hormones, such as cortisol and corticosterone, are secreted and used in a temporal- and organ-specific manner in response to internal and external stressors. They are ligands for the glucocorticoid receptor (GR) and the mineralocorticoid receptor (MR). Upon glucocorticoid binding, the receptors undergo structural alterations, revealing protein surfaces that interact with effectors of the signaling responses (Bledsoe et al., Citation2002; Li et al., Citation2005). The diversity of responses originates from a continuous exchange between the receptors and their diverse effector complexes, for which availability in time and space is defined by the environmental context. Agonist binding to GR and MR stabilizes an active conformational state, in which structural changes induce the exchange of chaperone complexes for (transcriptionally) active machinery. These exchanges involve newly exposed surfaces that are dynamic and controlled by the timing and demands of the cellular environment (Weikum et al., Citation2017). The ultradian and circadian modes of glucocorticoid secretions support programmed rhythms of conformational changes in both the GR and MR receptors (Kalafatakis et al., Citation2016), but glucocorticoid-independent signals contingent to the environmental context are also involved (Galliher-Beckley et al., Citation2011; Hapgood et al., Citation2016).
Cellular history of excitation and exposure to stress mediators may define the accessibility of ligands (glucocorticoids, nucleic acids, and co-regulators) to the core functional units. For the rapid non-genomic effects, the interactors of GR and MR are ill-defined. For transcriptional effects, the basic effector mechanism can be conceptually reduced to the ligand, the receptor, the DNA, and the transcriptional coregulators that interact directly with the receptors (Monczor et al., Citation2019). Of note, while the stress-induced glucocorticoid signal is predicted to mainly affect GR occupancy (Reul & de Kloet, Citation1985), GR and MR share many of their DNA binding sites (van Weert et al., Citation2017) and potential target genes, including often used readouts for receptor activity such as the Fkbp5 gene (van Weert et al., Citation2019). This implies that GR/MR signaling prior to a stressor will interact with stress-induced GR signaling. GR/MR and other nuclear receptors also share an important part of their coregulator repertoire (Broekema et al., Citation2018; Meijer et al., Citation2005). This points to intrinsic interactions between both GR and MR, and between glucocorticoids and other steroid signaling pathways. However, these may be considered stable over the time frame of hours, or often longer periods of time.
In contrast, the cellular biochemical state at the time of exposure to glucocorticoids is defining the context-dependent responses (). The post-translational modifications occurring on the glucocorticoid receptors are important allosteric determinants contributing to context-dependence (Faresse, Citation2014; Liberman et al., Citation2014). The range of effectors available for the different conformational states may depend on the organ, the cell type, the history of stress exposure, the ongoing cellular activity, and the subcellular distribution of GRs and MRs. At the synapse, GR and MR convey glucocorticoid-elicited effects on neurotransmission within seconds that are inconsistent with genomic actions (Groeneweg et al., Citation2011). These effects can be initiated at the plasma membrane or in the cytoplasm via non-canonical signaling pathways that interact with the slow genomic effects to organize long-term adaptive responses (Joels et al., Citation2013). Within the cytosol, the hormone-activated receptors can interact with protein partners to signal in non-genomic ways (Gutierrez-Mecinas et al., Citation2011). The nucleus and the mitochondrion are privileged locations for GR and MR, where they can bind to regulatory DNA elements (Du et al., Citation2009). These may be considered as ligands for GR and MR, affecting the conformation of the receptors via their DNA binding domain (Lapp et al., Citation2019; Meijsing et al., Citation2009). The availability of these “DNA ligands” is conferred by the interaction of glucocorticoid-dependent and glucocorticoid-independent signals that converge on chromatin (Bartlett et al., Citation2019). The binding of “RNA ligands” has also been described outside the nucleus, in P-granules where glucocorticoid receptors accelerate mRNAs turnover rates via a nuclear activated GR-mediated genomic action (Ishmael et al., Citation2011). The transcriptional effects are consistent with the rapid import of GR and MR into the mitochondria and the nucleus upon binding of glucocorticoids (Conway-Campbell et al., Citation2012; Lapp et al., Citation2019).
Figure 1. Dynamic allosteric forms of GR allow its diversity of responses. (A) Context-dependent parameters influence in time and space the availability of the various proteinous, chemical, and nucleic acid ligands of GR. (B) BDNF is an example of an activity-dependent factor that provides molecular and cellular context to GR signaling and diversity of responses. In this example, context A (but not B) and the presence of BDNF will induce the phosphorylation of GR and thus induce a differential response. (C) A principal component analysis of most regulated gene sets in cortical neurons stimulated with BDNF or dexamethasone (Dex) alone or in combination (BDNF + Dex). The transcriptional gene network elicited by coincident signals is distinct from the sum of individual gene modules (ref. Lambert et al., Citation2013). PC: principal component.
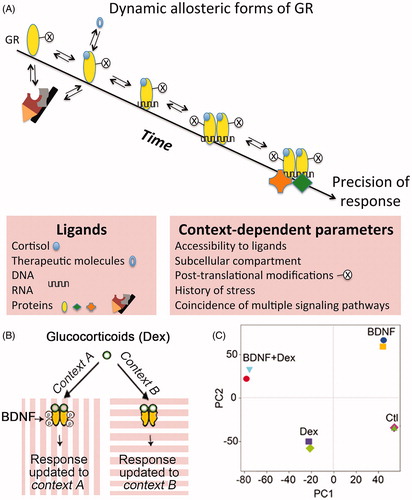
Although glucocorticoids are known to produce differential changes in gene expression among individuals, within different tissues and even from cells of the same individual, a recent meta-analysis identified a core set of genes systematically regulated in the same direction (Kan et al., Citation2018). Such a gene module apparently represents constitutively available DNA binding sites, and its regulation upon ligand exposure depends on the availability of the constitutive effector complexes to determine the route of glucocorticoid signaling (Mahfouz et al., Citation2016; Meijer et al., Citation2019). By contrast, the diversity of actions to the other sets of glucocorticoid-responsive genes involves glucocorticoid-independent factors (e.g. cell-specific chromatin landscape, subcellular local presence of RNA and protein coregulatory factors based on contextual cellular activity and biochemical makeup) to determine alternative routes of glucocorticoid signaling restricted in time and by the state of cellular activity (Clayton et al., Citation2019).
First, we discuss how the pairing of glucocorticoid signaling with neuronal activity tailors responsivity in brain cells, and unifies reactions of neurons that operate in functional networks. We then explore how the misalignment between activity-dependent and glucocorticoid-dependent actions may derail adaptation to stress, and promote the development of stress-related diseases. Finally, we provide a framework to understand how glucocorticoid-independent signals have the potential to increase the precision of glucocorticoid actions during stress by adopting specific allosteric states of GR and MR.
2. A molecular perspective
The precision of the glucocorticoid actions depends on multiple factors that are determined by specific cellular and physiological contexts, and integrated over time (Arango-Lievano & Jeanneteau, Citation2016). This section will explore how glucocorticoid receptors integrate multiple coincident signals via dynamic allosteric changes. Here, the term “allosteric” refers to the conformational changes on GR and MR triggered by the specific cellular and physiological contexts – not to confuse with orthosteric conformational changes brought by ligand binding- that define the conformational modulation required to pick the effector complexes that guide an appropriate route of glucocorticoid signaling. Although the conformational modifications of GR are known to convey gene, cell or physiological context information, it remains unclear whether each allosteric state corresponds to a unique response, whether allosteric states are dynamic and reversible, whether contextual signals can secure a specific allosteric state and finally, how these combinatorial effects are restricted in time.
2.1. Detection of coincidence signaling
Transcription factors respond to changes of neuronal activity (Yap & Greenberg, Citation2018). Such regulatory mechanisms may also be shared by ligand-dependent transcription factors like the GR and MR. Classic studies have paved the way for a detailed understanding of how these receptors are switched-on by cognate and synthetic ligands in different cell types. However, little is known about the impact of cellular experience on GR and MR activities. Coincident neuronal activity could interfere with many attributes of the glucocorticoid receptor signaling, including ligand availability, ligand binding, transport across compartments, post-translational modifications, and transcriptional activity (Arango-Lievano et al., Citation2015b). Post-translational modifications of the ligand binding domain and N-terminal transactivation domain, dynamic and reversible in nature, are likely associated with particular conformational transition states of glucocorticoid receptors (Weikum et al., Citation2017). For example, site-specific phosphorylation in the transcriptional activation domain of GR is sufficient to fold its disordered structure, producing new receptor surfaces for docking new protein partners in a context-specific manner (Khan et al., Citation2017). GR and MR are targeted by post-translational modifications at distinct sites involved in all aspects of glucocorticoid actions (Faresse, Citation2014; Liberman et al., Citation2014; Vandevyver et al., Citation2014). Some of these modifications are directed by non-steroid factors organized by prior experience and by ongoing cellular activity that can overlap in time with the glucocorticoid signaling.
Glucocorticoid-independent site-specific phosphorylation of the glucocorticoid receptors occurs in response to various physiological contexts (e.g. KCL-induced depolarization, oxidative stress, sensory stimulation, motor learning, enriched housing environment, antidepressant treatment). These contexts depend, in part, on the secretion of the activity-dependent brain-derived neurotrophic factor (BDNF), a neurotrophic factor playing key roles in neuronal plasticity ( and Arango-Lievano et al., Citation2015a, Citation2019). These sites are conserved among humans, mice, and rats and the phosphorylation was experimentally confirmed in each species (Arango-Lievano & Jeanneteau, Citation2016; Jeanneteau et al., Citation2019). A caspase-1 cleavage site resides near one of the BDNF-dependent phosphorylation sites in GR that is responsible for glucocorticoid resistance in humans with acute lymphoblastic leukemia suggests that healthy responses to glucocorticoids are in part determined by the conformational surfaces in the GR N-terminal domain and its dynamics to contextual post-translational modifications (Paugh et al., Citation2015). Interaction between contextual signals and glucocorticoid signaling per se was tested by co-stimulation of neurons with BDNF and glucocorticoids, which extended the duration of phosphorylation at these sites compared to a stimulation with BDNF only (Lambert et al., Citation2013). This can be explained by the activation of GR kinases (e.g. Erk1/2, [extracellular signal-regulated kinase 1/2], JNK [c-Jun N terminal kinase], P38) via the BDNF pathway and by the deactivation of the GR phosphatase PP5 [protein phosphatase 5] through the glucocorticoid pathway (Arango-Lievano et al., Citation2015a). Other kinases (e.g. CDK5 [cyclin-dependent kinase 5], GSK3 [Glycogen Synthase Kinase-3]) and phosphatases (e.g. PP1 [protein phosphatase-1]) involved in the phosphorylation of GR sites (Galliher-Beckley & Cidlowski, Citation2009; Ismaili & Garabedian, Citation2004) are common to many signaling pathways modulated during behavioral experience and neuronal activity (Yap & Greenberg, Citation2018).
Mutational analyses of phosphorylation sites in GR revealed the dependency between the BDNF and the glucocorticoid pathways for controlling the molecular determinants that dock specific effector complexes with the cAMP response element-binding protein 1 (CREB1), histone deacetylase 2 (HDAC2) and DNA (Lambert et al., Citation2013). Previous studies indicated the importance of such a coincidence-based interaction between the Erk1/2 kinase pathway and the glucocorticoid pathway to cope with stress on the molecular, cellular and behavioral scales (Arango-Lievano et al., Citation2015a; Chen et al., Citation2012a; Gutierrez-Mecinas et al., Citation2011; Revest et al., Citation2014). Accordingly, although BDNF-dependent GR phosphorylation occurred in the cytoplasm, its phospho-isoforms were detected in the nucleus, on the chromatin, in mitochondria as well as in synaptosomes. These findings explain the target-gene specific potentiation of GR actions that is observed after BDNF co-treatment (Lambert et al., Citation2013). It also defines GR as a detector of coincidence between the glucocorticoid and the BDNF pathways with the aim to link the external pressure of stress in the environment to the internal cellular state of activity. The subsequent unifying response is thus restrained within the temporal domain of GR phosphorylation (Arango-Lievano et al., Citation2015a). The biochemical half-life of GR phosphorylation ranges from minutes to hours – similarly, ligand-dependent activation of the GR may range from approximately 30 minutes (for ultradian peaks) to hours or days, depending on acute or chronic stressors (Russell et al., Citation2015). Overlaps of timing between glucocorticoid-dependent and glucocorticoid-independent signals are expected to determine the diversity and precision of responses within cells and among individuals, which for example is the case during learning and memory (Arango-Lievano & Jeanneteau, Citation2016; Schwabe et al., Citation2012).
The human Bdnf gene has a polymorphism (substitution of valine (Val) to methionine (Met) at codon 66 [Val66Met]), which affects BDNF trafficking and diminishes the activity-dependent release of BDNF in the brain (Egan et al., Citation2003). Homozygote carriers of the BDNF-Met66 allele reduce GR phosphorylation (Arango-Lievano et al., Citation2019), and under basal non-stressed conditions, display stress-related behavioral impairments in mice (Chen et al., Citation2006; Notaras et al., Citation2016, Citation2017; Soliman et al., Citation2010; Yu et al., Citation2012) and human homo and heterozygotes (de Assis & Gasanov, Citation2019; Shalev et al., Citation2009). Further deletion of BDNF-dependent GR phosphorylation sites in the context of the BDNF-Val66Met polymorphism failed to alter stress-induced plasticity in mice (Arango-Lievano et al., Citation2019). This indicates that site-specific GR phosphorylation is important in stress regulation and stress-related behaviors, acting downstream of activity-dependent BDNF signaling in vivo.
Other post-translational modifications likely influenced by experience and neuronal activity such as acetylation, ubiquitination, sumoylation, and nitrosylation were implicated in the regulation of GR and MR turnover, transport and binding to corticosterone and DNA (Arango-Lievano et al., Citation2015b; Weikum et al., Citation2017). However, none of these post-translational modifications in GR were altered by BDNF stimulation of neuronal cells (Arango-Lievano & Jeanneteau, Citation2016). Consistently, mutations of BDNF-dependent phosphorylation sites did not interfere with GR turnover, transport, corticosterone binding, or DNA binding (Arango-Lievano & Jeanneteau, Citation2016). This suggests that phosphorylation affords some level of contextual specificity that other modifications do not. The allosterically induced context-dependent conformational states of GR, acquired by the post-translational modifications, do not necessarily set overall responsiveness, but may instead increase the precision of response to serve cellular and organismal demands. They may also involve co-regulators of the steroid receptors to fine tune responses, as exemplified by the cell state dependent phosphorylation of the SRC2/GRIP1 effector of GR and MR (Rollins et al., Citation2017). More studies are necessary to understand the significance of GR and MR post-translational modifications and their dynamics, as well as their consequences on physiological stress responses and stress-related behaviors (Garabedian et al., Citation2017).
2.2. Epigenomic priming
The diversity of genome-wide occupancy of GR in different cell types is dictated by chromatin loci that were already opened or accessible de novo upon stimulation with glucocorticoids (John et al., Citation2011). This may be similar for MR that binds to DNA already exposed to transcriptional complexes of cell fate determination and plasticity like NeuroD and GR (Mifsud & Reul, Citation2016; van Weert et al., Citation2017). Most GRs interact with already-open chromatin before receptor activation while de novo opening of the chromatin was reported at a minority of loci (Burd et al., Citation2012). Interactions between GR and local chromatin occur directly and indirectly through the recruitment of histone/DNA-modifying enzymes (Bartlett et al., Citation2019). At resting state, most “potential sites” for GR binding in the genome reside in the inaccessible chromatin. Accessibility to these loci is gained by various stimuli coincident to glucocorticoid stimulation (e.g. neuronal activity, growth factors, neurotransmitters, steroid hormones) that create an opportunity for GR binding and gene regulation (Klemm et al., Citation2019). Opening the chromatin to expose new epigenomic landscapes before the nuclear translocation of GR, influences the frequency of GR–DNA binding events. Indeed, experience, neuronal activity, and other conditioning factors can change the frequency of transcription factor binding to specific genome loci (Kitagawa et al., Citation2017), acting as potent modifiers of chromatin topology by enzymes working on DNA or histones (Yap & Greenberg, Citation2018). Prolonged exposure to exogenous glucocorticoid is an example of conditioning that can alter future stress responses by changing DNA methylation at target genes (Provencal et al., Citation2019). In the hippocampus, corticosterone regulated the expression of genes that overlapped by only 50% between unstressed controls and animals prior exposed to chronic stress (Polman et al., Citation2013). The social context, like the hierarchical rank in a group of individuals is another example of conditioning that can alter chromatin accessibility and transcription at glucocorticoid-responsive genes (Snyder-Mackler et al., Citation2019). These examples illustrate the interaction of glucocorticoid exposure and behavioral experience on the epigenome, and that stress responsivity may be shaped accordingly.
The persistence of chromatin remodeling at glucocorticoid responsive loci is an adaptive process intended to influence cell responses to subsequent events based on prior experience, like a memory. Methylation of DNA is supposed to serve this function (Bird, Citation2002). For example, methylation of Nr3c1 -the gene encoding GR- in the context of childhood trauma predicts changed physiological reactivity to stress in rodents (Liu et al., Citation1997), and was reported to be associated with major depressive episodes and suicide attempts at adulthood (McGowan et al., Citation2009). To avoid such maladaptive responses, the epigenomic priming of GR responsivity should be reversible (Yang et al., Citation2016). The DNA methylation is linked to changes in histone acetylation and phosphorylation that serve as a multi-layered code of epigenomic regulation used to update new information based on prior experience (Bousiges et al., Citation2013; Crosio et al., Citation2003). Such feed-forward mechanisms can operate in multiple environmental and behavioral contexts to regulate the precision and magnitude of responses to glucocorticoid receptors (Bartlett et al., Citation2019). For example, BDNF signaling promotes nitrosylation of HDAC2, which reduces its enzymatic activity and localization on the chromatin (Nott et al., Citation2008). The interconnectedness of regulatory mechanisms is exemplified by the fact that besides histones, one prominent substrate of HDAC2 is GR, whose deacetylation impairs the binding with protein transcription factors like nuclear factor kappa B (NF-κB) (Ito et al., Citation2006). GR deacetylation by HDAC2 has been implicated in the pathophysiology of glucocorticoid resistance in chronic obstructive pulmonary disease, which is also associated with impaired phosphorylation dynamics of GR by kinases pathways (Barnes, Citation2009, Citation2013). As an additional example of epigenomic regulation, GR-DNA binding frequency is significantly increased if chromatin loci are co-occupied by GR and CCAAT-enhancer-binding proteins (C/EBP), representing a cooperative mechanism involving again BDNF and glucocorticoid pathways (Grontved et al., Citation2013). In general, cell-type-specific gene regulation is supposed to involve the co-occurrence of GR and MR with its machinery and the cell-type-specific chromatin accessibility and DNA methylation (Datson et al., Citation2011; Gertz et al., Citation2013; Pooley et al., Citation2017; van Weert et al., Citation2017). Future ChIP-seq studies shall provide information regarding the genome-wide positioning of GR and MR in distinct cell types, and different physiological contexts.
2.3. Transcription of gene networks
Less than 1% of chromatin loci to which glucocorticoid receptors bind in one cell type, is actually common to other cell types. The heterogeneity in transcriptional patterns that are important for programming the stress response is reduced when comparing cell types issued from the same tissue of the same mouse, and yet meta-analyses emphasize more differences than similarities (Crow et al., Citation2019; Sacta et al., Citation2016). Therefore, studies of particular cell subpopulations, in the context of synchronized neuronal activity or stimulation with activity-dependent factors like BDNF, are necessary to clarify what determines the direction and magnitude of transcriptional regulation by glucocorticoids in the genome. A co-stimulation of neurons with BDNF and the GR synthetic agonist dexamethasone leads to a distinct transcriptome from the one elicited by individual stimuli, and that reflected interactions, rather than merely additive effects between treatments (). Although similar gene modules are consistently repurposed across contexts, some gene clusters are specific to salient experiences, stress, and coincident BDNF and glucocorticoid signaling (Lambert et al., Citation2013; Mukherjee et al., Citation2018). Overlap and dependency between gene expression modules containing DNA-binding elements for activity-dependent transcription factors as well as for GR and MR are likely essential to determine the precision of a stress response gene network tailored to the contextual demands.
GR and MR use their DNA-binding domain zinc fingers to make contact with glucocorticoid responsive elements (GRE). The nature of GRE, in terms of sequence identity or orientation, varies across target genes in the genome and instructs either positive or negative cooperative remodeling of the dimerization motif that facilitates (homo- or hetero) dimers, tetramers or monomers (alone or tethered to another transcription factor) to sit on DNA (Paakinaho et al., Citation2019; Schiller et al., Citation2014; Watson et al., Citation2013). Thus, the interaction between receptors and DNA response elements allosterically stabilizes particular conformational forms that guide the direction of responses (Hudson et al., Citation2013). These events on chromatin are very dynamic, consisting of association and dissociation exchanges within seconds, a time frame compatible with integrating coincident contextual signals at high frequencies (Weikum et al., Citation2017). This integration can be mediated by direct protein–protein interactions between the receptors and other transcription factors but also by indirect effects via inhibitory long noncoding RNAs, or by competition for nuclear coactivator proteins (Rapicavoli et al., Citation2013; Ratman et al., Citation2013). For example, glucocorticoid-activated GRs can be recruited to specific loci in the genome without binding directly to DNA (i.e. via protein–protein interactions with other DNA-bound factors likely responding to signaling elicited by neuronal activity). Both the direct and tethering modes are associated with distinct transcriptional attributes (Vockley et al., Citation2016). However, GR and MR were mostly detected on GRE-dependent motifs in “bulk” hippocampal tissue under resting condition and after stress (Polman et al., Citation2013; Pooley et al., Citation2017; van Weert et al., Citation2017). This implies that the detection of coincidence, via interaction of GR with activity-dependent transcription factors, would take place in small subset of cells – in accordance with the mosaic-like activation of neurons, for example in learning processes (Rao-Ruiz et al., Citation2019).
Intracellular calcium (Ca2+) is a central mediator of transcriptional regulation and is controlled by neuronal activity that could gate GR and MR responsiveness either through the direct or indirect mode of transcriptional regulation (Zhang et al., Citation2009). Several phosphatases, proteases, and cargo transporters operating as Ca2+-sensors are involved in the response to glucocorticoids in multiple cellular compartments. Actions on synaptic neurotransmission and on nuclear gene transcription, which are mediated by GR and MR, require coincident Ca2+ mobilization from the mitochondria and the endoplasmic reticulum (Chameau et al., Citation2007; Harris et al., Citation2019; Mayanagi et al., Citation2008; Simard et al., Citation1999). Mobilization of Ca2+ and cAMP are both required for the nuclear import of the CREB-regulated transcription coactivators (CRTC1/2/3), a family of cofactors for CREB and GR (Altarejos & Montminy, Citation2011; Hill et al., Citation2016). In particular, CRTC2 can integrate BDNF and glucocorticoid signals in the hypothalamus to control the direction and magnitude of transcription at the corticotropin-releasing hormone (Crh) promoter and the response of the hypothalamic-pituitary-adrenocortical (HPA) axis to stressors (Jeanneteau et al., Citation2012). This molecular pathway also regulates glucose metabolism and the energetic adaptation to stress by controlling the expression of the rate-limiting enzymes glucose-6-phosphatase and phosphoenolpyruvate carboxykinase in the liver (Hill et al., Citation2016).
Future studies employing single-cell transcriptomics will be useful in capturing the impact of experience and activity on the refinement of gene expression modules, since it allows the dissection of global gene expression in specific cell types during defined stress environment and behavioral state. This method could lead to the identification of subpopulations of cells activated in certain contexts, such as learning a task or being exposed to psychostimulants. For example, differential gene expression profiles were induced by morphine in astrocytes and oligodendrocytes collected from the same tissue of the same animals (Avey et al., Citation2018). Similarly, the influence of ongoing cell excitation (e.g. during visual stimulation of the retina) can impact the expression of GR regulated gene modules that is consistent with the role of GR on the plasticity of the visual system (Muto et al., Citation2013). In conclusion, experience and activity-dependent control of GR and MR assembly with ligands, DNA and coregulators are essential to precisely update and maintain the diversity of the responses.
3. A cellular perspective
As outlined above, glucocorticoid responsiveness varies significantly across cell types, brain regions, neurocircuits, and physiological states (Chattarji et al., Citation2015; Franco et al., Citation2019; Joels et al., Citation2013). This section will explore how glucocorticoid receptors integrate multiple coincident signals, which are restricted to certain time domains and depend on neuronal experience, to modify cellular functions like migration, differentiation, connectivity, and neurotransmission. Contextual signaling of glucocorticoid receptors is critical for understanding how both insufficient and excessive glucocorticoid signaling can have a similar impact on neuronal functions (Yu et al., Citation2019). However, it remains unclear whether glucocorticoid action on a broad range of subcellular organelles (e.g. mitochondria, nucleus, and synapse) is uniform or on the contrary multiform in time and space. Each site of action is likely to contribute to the overall adaptive program set by glucocorticoids. The following section focuses on the nuclear and synaptic actions but other actions on the mitochondria, the sarcoplasmic reticulum are described elsewhere (Choi et al., Citation2018; Lapp et al., Citation2019; Picard et al., Citation2014).
3.1. At the single-cell level
Long-range signaling from synapses to the nucleus is essential to store information in the epigenome and modify neuronal fate with a novel arsenal of transcripts (Deisseroth et al., Citation2003). Mobilization of Ca2+ at the synapse, via voltage-gated Ca2+ channels, sets in motion a series of Ca2+-sensing mechanisms driving synaptic effectors like nuclear factor of activated T-cells (NF-AT) directly into the nucleus (Hagenston & Bading, Citation2011; Wild et al., Citation2019). Acting as tethering co-activator of GR, NF-AT is expected to control the direction of transcription at selective glucocorticoid-responsive target genes in neurons as in immune cells (Petta et al., Citation2016). Long-range transport of cargoes between synapses and the nucleus is bidirectional, and may serve as tagging systems for promoting synapse-specific plasticity based on prior experience (Frey & Morris, Citation1997). Activity-dependent tags such as the BDNF-activated receptor Tropomyosin Related Tyrosine Kinase-B (TrkB) trap new mRNA transcripts from the soma with the protein synthesis machinery in specific synapses (Lu et al., Citation2011). Long-range transport of signaling endosomes harboring phosphorylated TrkB from synapse to nucleus contributes to an important feedforward mechanism that prepares cellular responsiveness based on prior stimulation (Yamashita et al., Citation2017). GR can bind directly to TrkB (Numakawa et al., Citation2009) but it is unknown whether the phosphorylated isoforms of GR can traffic along with the activated TrkB cargoes (Barford et al., Citation2017). Cis/trans-isomerization of phosphorylated residues on GR increases its persistence overtime by preventing dephosphorylation (Poolman et al., Citation2013). Several cis/trans-isomerases (e.g. peptidyl prolyl isomerases FKBP4, FKBP5, and PIN1) form complexes with GR and MR to regulate their activity, transport and signaling across organelles in the context of stress (Binder, Citation2009; Wochnik et al., Citation2005; Zhang et al., Citation2008). Cis/trans-isomerases of GR and MR complexes are often presented as important modifiers of future responsiveness to glucocorticoids and in diseases of stress sensitivity and responsiveness (Binder et al., Citation2004; Fries et al., Citation2015; Galigniana et al., Citation2012; Ratajczak et al., Citation2015).
Mechanisms of adaptive plasticity utilize immediate early genes as first-line responders to glucocorticoid stimulation to prepare cells for a changing environment based on prior experience. For example, the MAPK specific phosphatases (Mitogen-Activated Protein Kinase Phosphatase 1/6 [MKP1/6]) are induced to terminate coincident growth factor signaling pathways converging on the GR phosphorylation code and the epigenome (Deinhardt & Jeanneteau, Citation2012; Jeanneteau & Deinhardt, Citation2011). Another example is the stress-induced transcription factors NR4A1/2/3, which shuttle in and out of the nucleus and mitochondria to coordinate metabolism and synapses number (Jeanneteau et al., Citation2018). Finally, actin binding proteins such as the Ca2+ sensor caldesmon and the cofilin kinase LIM-kinase 1 (LIMK1) are induced to reorganize the cytoskeleton supporting neuronal migration, differentiation, and connectivity (Fukumoto et al., Citation2009; Mayanagi et al., Citation2008; Morsink et al., Citation2006). Altogether, these responses set in motion the adaptive machinery that updates many attributes of neuronal function to its environment based on prior experience.
The sequence in which an acute glucocorticoid pulse and neuronal activity follow each other further impacts the final response. In vitro, activation of GR prior to, or in conjunction with, cAMP signaling suppresses the transcription at the Crh gene promoter, a repressive effect that failed to develop when cAMP signaling preceded GR activation (van der Laan et al., Citation2009). In vivo, the GR-mediated control of Crh transcription is strikingly opposite in the hypothalamus and the amygdala of the same mouse depending on the behavioral context (Jeanneteau et al., Citation2012; Zalachoras et al., Citation2016). Therefore, the direction and magnitude of glucocorticoid response may depend not only on the intrinsic presence of cell type-specific mediators, but also on its timing with other contextual signals that change cAMP and/or Ca2+ concentrations, like during neuronal spiking activity.
The time-dependence of glucocorticoid effects on synapses would support the opposite effects of glucocorticoids on dendritic spines, reported to be branch-specific and distinct across brain regions and circuits (Arango-Lievano et al., Citation2016; Chattarji et al., Citation2015; Dias-Ferreira et al., Citation2009). Some of these effects rely on the remodeling of a major constituent of dendritic spines, the actin cytoskeleton, by rapid non-genomic and slow genomic glucocorticoid signaling (Stournaras et al., Citation2014). The dynamic rates of formation and elimination of dendritic spines increase throughout the cortex by exogenous glucocorticoids whereas removal of endogenous glucocorticoids from the brain canceled such a turnover (Liston & Gan, Citation2011). This was demonstrated by low-dose injection of dexamethasone, which does not penetrate the brain but suppresses endogenous corticosterone secretion via the pituitary axis (Karssen et al., Citation2005). New thin spines formed upon glucocorticoid exposure are immature and very unstable. Both the membrane-bound GR and MR (mGR and mMR) previously located in the synapse (Johnson et al., Citation2005; Prager et al., Citation2010) regulate spine structure via rapid non-genomic mechanisms upon circadian and experience-dependent fluctuations of corticosterone (Russo et al., Citation2016). However, upon elevation of corticosterone by acute or chronic injections, the nuclear GR and MR also take part in the process of stabilization and elimination of dendritic spines via genomic mechanisms (Liston et al., Citation2013; Liston & Gan, Citation2011). Maintenance of a significant proportion of new spines requires additional coincident contextual signals influencing Ca2+ spike activity to form functional synapses (Cichon & Gan, Citation2015). This means that the circadian/ultradian rhythms of glucocorticoid secretions provide a time-restricted opportunity for persistent remodeling of synaptic connectivity at active dendritic branches whereas other silent dendritic branches, out of behavioral context, are not affected over long time periods (Liston et al., Citation2013).
The consolidation of new dendritic spines formed at the time of learning requires a mechanism of coincidence detection of BDNF and glucocorticoid signaling pathways. It is restricted to the training period and it involves the retention of new glutamatergic AMPA receptors via GR phosphorylation (Arango-Lievano et al., Citation2019). Local dendritic synthesis of AMPA receptors increases their abundance at synapses and strengthens the new synapses associated with learning (Chater & Goda, Citation2014). This raises the threshold for the later induction of synaptic potentiation, which may help to consolidate experience-related information and prevent other context-unrelated information from being stored in these synapses (Sarabdjitsingh et al., Citation2014). Similar effects are anticipated with MR, as both GR and MR regulate synaptic transmission by modifying AMPA receptors (Conboy & Sandi, Citation2010; Groc et al., Citation2008) and the release of glutamate by the pre and or post-synapses (Karst et al., Citation1999; Tasker, Citation2006). Chronic stress exaggerates the elimination of certain pools of dendritic spines, in particular those associated with learning. Such an effect can be reversed by a chronic treatment with antidepressant drugs. Further optogenetic erasure of this particular pool of dendritic spines impaired the physiological and behavioral effects of the antidepressant drugs (Moda-Sava et al., Citation2019), suggesting that information pertaining to behavioral experience can be stored in synaptic maps over extended periods of time (). Precision of glucocorticoid response is tailored to the time frame and brain area involved in contextual behavioral experience via the combinatorial effects of MR on basal firing frequency and spine elimination, and GR on neuronal activity and spine formation/maintenance (Groeneweg et al., Citation2011; Heshmati & Russo, Citation2013; Joels, Citation2008).
Figure 2. Timing of glucocorticoid signaling with neuronal activity impacts the adaptive response of individual cells and in networks. (A) Glucocorticoid-induced plasticity of dendritic spines is influenced in time and space by context-dependent mechanisms across cells and networks. Maps of dendritic spine synapses are reorganized as a function of glucocorticoid (GC) physiological rhythmic secretions and contextual experiences. Optical erasure of one such synaptic map of learning impairs behavioral performance. Boxes show rules of glucocorticoid spine plasticity and interaction with context (Arango-Lievano et al., Citation2015a; Liston et al., Citation2013; Moda-Sava et al., Citation2019). (B) Types of tissue responses to glucocorticoid receptors (GR). Mosaic responses depend on context at stimulation to make GR ligands available in time and space. Such mosaic may represent cellular assemblies in functional network. (C) Phosphorylation is one type of post-translational modification that can modify GR allostery and its availability to ligands in time and space. Glucocorticoid-dependent phosphorylation of GR follows the homogenous model whereas BDNF-dependent phosphorylation of GR follows the network model and is co-expressed with the cellular marker of activity c-fos in pyramidal cortical neurons labeled with thy1-YFP reporter (Ref. Arango-Lievano et al., Citation2019). GC: glucocorticoids; GR: glucocorticoid receptor.
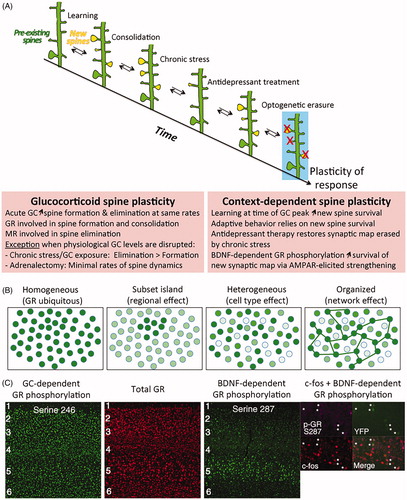
3.2. At the network level
Stress affects large-scale networks in the brain (e.g. executive, default mode, salience, valence, and enteroceptive) to promote vigilance, attention, learning, and adaptation (Chattarji et al., Citation2015; McEwen et al., Citation2015). For example, the salience and the default mode networks are upregulated by stress while, at the same time, the executive control network is downregulated; such effects yet depend on the nature, intensity, and duration of stressors (Clemens et al., Citation2017). The response of large-scale brain networks enables individuals to better reorient attention, detect salient external stimuli based on its enteroceptive state, and deal with the emotional and affective consequences of stress. Glucocorticoid receptors constantly interact with ongoing neural circuit activity of large-scale brain networks to prepare suitable physiological and behavioral response outcomes based on prior experience (Herman et al., Citation2016; Joels et al., Citation2006). The precision of responses to glucocorticoids at the neuronal network level depends on the organization of the circuit and types of cells involved. It also depends on glucocorticoid availability, which can be influenced by tissue- and context-specific expression of corticosterone/cortisol biosynthesis enzymes, like the hydroxysteroid dehydrogenases 1 and 2 (HSD1/2) (Myers et al., Citation2014; Ramamoorthy & Cidlowski, Citation2013). To date, it is not known whether the delivery of effective concentrations of glucocorticoids can be targeted locally to specific cellular microenvironments or even subpopulations of cells in a brain area at a given time (). However, it is well established that the GR/MR ratio varies across brain regions and cell types, and influences the diversity of responses to glucocorticoids in normal physiological and pathological conditions (De Kloet et al., Citation1998; Joels & de Kloet, Citation1994).
Despite GR apparent ubiquitous expression, its phosphorylation and nucleocytoplasmic ratio can vary across brain regions, cell types, and neuronal network depending on the behavioral context (Adzic et al., Citation2009; Mitic et al., Citation2013). During learning, phosphorylation of GR at glucocorticoid-responding sites is uniform throughout the cortex whereas phosphorylation at BDNF-responding sites is increased during the learning period and enriched in specific cortical layers, cell types and co-expressed with c-Fos, a marker of neuronal activity (). Mutation of BDNF-dependent GR phosphorylation sites impairs the formation and stabilization of branch-specific dendritic spines only in the context of learning (Arango-Lievano et al., Citation2019). The stability of dendritic spines is predicted by its size more than by its activity (Quinn et al., Citation2019). Consistently, the head size enlargement of spines, at the pool of new synapses induced by learning, is blocked by the GR antagonist RU486 and by the mutation of BDNF-dependent GR phosphorylation sites, both of which are required for enhancing the post-learning glutamatergic synaptic response. By contrast, the pool of preexisting spines, which are unrelated to learning, is not sensitive to either manipulation. Such findings are consistent with the impairment of long-term potentiation (LTP) at cortical synapses in the context of learning, in mice lacking BDNF-dependent GR phosphorylation sites, and with the absence of impairment in LTP between controls and mutant mice outside the learning context (Arango-Lievano et al., Citation2019). Changes in synapse strengths and spine dynamics during learning acquisition is selective of neuronal networks expressing BDNF-dependent GR phosphorylation and associated with significant improvement of behavioral performance during the consolidation and retention phases (Yu & Zuo, Citation2011). This illustrates how specific contextual events influence GR allosteric forms and its precision of actions across neuronal ensembles that respond to the same behavioral experience, acting like a functional network.
The precision of glucocorticoid action also varies across neuronal networks if the context of stress occurs during the ascending or descending phases of the ultradian glucocorticoid oscillations (Sarabdjitsingh et al., Citation2010a, Citation2010b, Citation2010c). Impairment of glucocorticoid secretion rhythms, by chronic stress exposure, exaggerates the elimination of dendritic spines, most notably the new experience-associated pool but also a tiny fraction of the preexisting pool (Arango-Lievano et al., Citation2019; Chen et al., Citation2017). Some of the consequences of such impaired rhythmicity, along with behavioral alterations, can be reversed by antidepressant treatments and physical exercise through a mechanism requiring BDNF/TrkB and downstream GR phosphorylation event (Arango-Lievano et al., Citation2019; Chen et al., Citation2017). The stress-induced synapse elimination is observed in cortical glutamatergic neurons and parvalbumin interneurons where GR phosphorylation at BDNF-responding sites is significantly enriched and, together with behavioral alterations, was prevented by chemogenetic tools, enriched housing conditions or ketamine used at dosage affording antidepressant effect (Arango-Lievano et al., Citation2015a; Chen et al., Citation2018; Ng et al., Citation2018). Mechanism of action may rely on the experience and activity-dependent co-clustering dynamics of inhibitory synapses and dendritic spines in the cortex (Boivin & Nedivi, Citation2018; Chen et al., Citation2012b). Other mechanisms may involve the retrograde trans-synaptic cannabinoid and opioid signaling to suppress the release of neurotransmitters at hypothalamic glutamatergic and GABAergic synapses in a time-period compatible with the remodeling of synaptic contacts (Di et al., Citation2009; Wamsteeker Cusulin et al., Citation2013). Inhibitory control of excitatory drive by interneurons, including the parvalbumin subclass, is critical to determine the direction and magnitude of responses to stress and glucocorticoids in hypothalamic, amygdalar, hippocampal, and cortical neuronal networks. In the amygdala, both GR and MR are necessary to switch neuronal responses to glucocorticoids from excitatory to inhibitory (Karst et al., Citation2010). In the neurogenic region of the hippocampus, glucocorticoid receptors also regulate the balance of excitation and inhibition with consequences on newborn cells maturation, migration, and integration into new networks (Saaltink & Vreugdenhil, Citation2014). Heterogeneity of neurogenic responses to glucocorticoid oscillations, acting through GR, determines the fate of progenitor cells between quiescence and proliferation by a mechanism involving a crosstalk between GR signaling and DNA methylation on several promoters of genes modulating the cell cycle (Schouten et al., Citation2020).
The dynamic activation and termination of the neuroendocrine HPA axis response to stress requires a fine balance of excitation and inhibition across multiple brain regions and cell types, which condition physiological and behavioral adaptation to environmental demands, based on prior experience (Herman et al., Citation2016). The stress response uses modulators, like noradrenaline, to integrate activity in a network of interconnected forebrain regions including the amygdala, the frontal cortex, the bed nucleus of the stria terminalis, the hypothalamus, and the hippocampus (Herman, Citation2013; Ulrich-Lai & Herman, Citation2009). Tissue and cell type-specific conditional genetic deletion of glucocorticoid receptors further demonstrated that the diversity of glucocorticoid responses on physiological, neuroendocrine and behavioral outcomes depends on the organization and ongoing activity of the neuronal networks involved (Arango-Lievano & Jeanneteau, Citation2016; Arnett et al., Citation2016; Scheimann et al., Citation2018; Whirledge & DeFranco, Citation2018). This might be explained by a Bayesian process used by the brain to update current information based on prior experience through multiple iterations of neuronal network activation intended to minimize the entropy of various sensory states and predict future outcomes based on value coding (Doya et al., Citation2007; Ernst et al., Citation2006).
In conclusion of this part, the mGR and mMR via non-genomic mechanisms, and the genomic GR and MR integrate the physiological glucocorticoid pulse secretions with coincident cellular activity to shape, within time and space, the diversity of responses tailored by ongoing demands for future adaption based on prior experience.
4. A behavioral perspective
Failure to control the HPA axis according to the environmental demands is associated with pathological states (Holsboer & Ising, Citation2010; Kalafatakis et al., Citation2016; Sarabdjitsingh et al., Citation2010a, Citation2010c). The functional dynamics and plasticity of the neurocircuits upstream and downstream of the HPA axis, determine the diversity of stressor-specific responses (Herman et al., Citation2003). Stress-coping strategies facilitate the adaptation to acute environmental challenges and internal demands, but they can also become maladaptive when the price for maintaining physiological stability does not match with the real costs of adaptation, which represents the allostatic load (Picard et al., Citation2014). If chronic in nature, the maladaptive processes can decrease the diversity of glucocorticoid actions across neural networks, reduce the number of stress-coping options and lead to behavioral rigidity and stress-related disorders (Karatsoreos & McEwen, Citation2011; McEwen, Citation1998; ). Therefore, promoting the diversity of glucocorticoid responsivity across brain cells and networks seems essential for maintaining optimal health. This section explores how variations in glucocorticoid-related molecular signaling pathways in neuronal networks of the brain, through gene variants or epigenetic mechanisms, impact stress coping, behavioral adaptation, and stress-related disorders.
Figure 3. Adaptive plasticity through diversity of responses to glucocorticoid hormones underlies individual differences among adaptive trajectories to stress and between resiliency or vulnerability to stress-related disorders. Contextual factors involved in maladaptive stress responses influence the susceptibility to stress-related disorders. Glucocorticoid receptors operate as coincidence detectors between the HPA axis activity and the environmental context to update the precision and maintain diversity of response based on prior experience. For example, the GR employs a diversity of allosteric/orthosteric forms and post-translational modifications across a diversity of chromatin landscapes in multiple cell types and neuronal networks to promote pleiotropic cellular, physiological and behavioral responses based on the variety of demands in the brain and body. Insights from clinical and rodent studies show that the individual variability in susceptibility or resilience to stress-related disorders results from a complex interplay between the perceived stress, context-dependent factors (genetic predisposition, history of stress, ongoing experience) and changes in BDNF and GR signaling pathways across large-scale brain networks. The capacity of an organism to deal with a chronic activation of the HPA axis (increased allostatic load) determines the extent of behavioral, physiological, neuroendocrine and neuronal changes in brain regions regulating cognition, reward, fear, emotionality and social behaviors. GC: glucocorticoids; VTA: ventral tegmental area; Hypoth.: hypothalamus; HPA axis: hypothalamo-pituitary-adrenal axis.
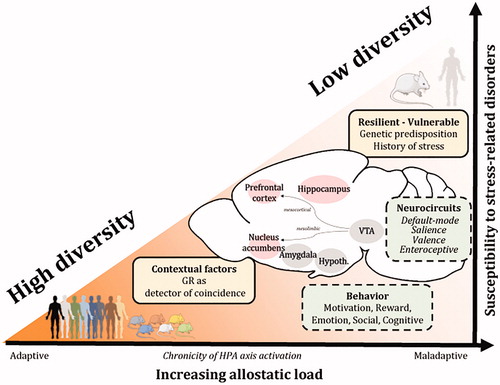
4.1. Stress-coping and behavioral adaptation
The diversity of strategies used to cope with environmental changes may be related to the individual differences in susceptibility to stress exposure. Stress-coping has been defined as the behavioral and physiological efforts to adjust with aversive stimuli or stressors (Lazarus & Folkman, Citation1984). Because the unpredictability of a situation is a stressor, coping may employ a predictive coding through the salience, executive and valence-coding neuronal networks to reduce the uncertainty about the selection of actions based on predicted consequences (Peters et al., Citation2017). More precisely, the neuroanatomical correlates of stress-coping involve the coordinated activities of the enteroceptive network (e.g. insular cortex), the default mode network at resting states, the salience network (e.g. orbitofrontal cortex, anterior cingulate cortex, and the amygdala) and the executive network (e.g. frontostriatal network) (Joels et al., Citation2006). Stress-induced glucocorticoid signaling overlaps with the mesocorticolimbic dopaminergic pathway to bias decision-making of goal-directed behaviors in both humans and animals (Gourley et al., Citation2012; Peters et al., Citation2017; Soares et al., Citation2013). In terms of learning and memory, stress, via glucocorticoid signaling, enhances memory formation when the stressor is relevant and proximal to the learning context, but stress impairs retrieval of learned information when the stressor occurs out-of-context. In particular, stress experienced directly prior to learning may activate a salience network and promote emotional learning, whereas hours after stress, the activity of executive control networks is facilitated through a diversity of responses by glucocorticoid receptors (Joels & Baram, Citation2009; McEwen, Citation2007). The diversity of stress-coping responses depends on the interaction of glucocorticoids with multiple neurotransmitter systems (i.e. dopaminergic, serotoninergic, glutamatergic, and peptidergic) operating in large-scale synaptic networks of selective brain regions. They enable individuals to better reorient attention, detect salient external stimuli, and deal with the emotional and affective consequences of stress.
The coping strategies have been described as either proactive or reactive (also referred to as “active” and “passive” respectively). Proactive stress-coping behaviors in rodents are generally associated with decreased depression-like phenotypes after exposure to chronic stress paradigms (de Boer et al., Citation2017; Wood & Bhatnagar, Citation2015). Mice genetically selected for extremes in aggressive behavior show different coping styles that can predict the susceptibility to chronic social stressors in adulthood (Koolhaas et al., Citation2017; Veenema et al., Citation2004). Studies with rats, selected for low, intermediate and high glucocorticoid responsivity to stress, distinguish subjects based on singular coping strategies in response to various challenges (Huzard et al., Citation2020; Walker et al., Citation2017, Citation2018). Moreover, mouse lines selectively bred for high (HR) vs. low (LR) HPA axis reactivity to a psychological stressor (15-min restraint) show a hyperactive vs. more passive behavioral response in tests assessing stress-coping behaviors. Interestingly, the different coping strategies of HR and LR mice are also associated with distinct alterations in emotional behaviors, cognitive performance, circadian activity patterns, sleep architecture, and neuronal functions resembling the symptomatology seen in patients suffering from the melancholic and atypical subtypes of depression, respectively (Fenzl et al., Citation2011; Heinzmann et al., Citation2014; Knapman et al., Citation2010; Touma et al., Citation2008, Citation2009). Looking at Gene × Environment interaction effects in this mouse model of affective disorders, the genetic predisposition for an increased neuroendocrine stress reactivity in HR mice amplified the deleterious effects of early-life adversity on stress-coping behavior and other relevant depression-like endophenotypes, possibly involving the BDNF signaling pathway (McIlwrick et al., Citation2016, Citation2017).
Although the ability of an organism to cope with environmental challenges depends on brain-wide glucocorticoid receptor signaling (Deroche-Gamonet et al., Citation2003; Tronche et al., Citation1999), different populations of neurons may have unique roles in modulating stress-induced behaviors. To better understand how specific neuronal circuits and brain cell populations can influence stress-related behaviors, we and others have selectively inactivated the GR using the Cre/Lox or the CRISPR/Cas9 gene editing systems (Arango-Lievano & Jeanneteau, Citation2016; Arnett et al., Citation2016; Kellendonk et al., Citation2002; Whirledge & DeFranco, Citation2018). Under basal, non-stressed conditions, the deletion of Nr3c1 in dopamine-receptive neurons, but not in dopamine-releasing neurons, reduces drug-related behaviors without affecting HPA axis activity and anxiety-like and stress-coping behaviors (Ambroggi et al., Citation2009; Barik et al., Citation2013; Parnaudeau et al., Citation2014). Under stressful conditions, it also prevents the development of social avoidance behavior (Barik et al., Citation2013). Together, these studies confirm the importance of cell-type-specific GR signaling in motivational and social reward behaviors (Douma & de Kloet, Citation2020). The cellular specificity could explain the differential modulation of GR activity in different areas of the mesolimbic and corticolimbic networks during reward-directed behaviors (Deroche et al., Citation1995; Gourley et al., Citation2012; Piazza & Le Moal, Citation1996; Swanson et al., Citation2013). The knockdown of GR in the forebrain reduces anxiety-like and active-coping behaviors, and it increases HPA axis activity and impairs the glucocorticoid negative feedback (Boyle et al., Citation2005, Citation2006; Jeanneteau et al., Citation2012; Laryea et al., Citation2013). Yet, the specific deletion of GR in glutamatergic neurons of the forebrain shows region-specific effects: the deletion of GR in the amygdala reduces the fear response while a deletion in the prelimbic prefrontal cortex increases proactive-coping behaviors in males (Hartmann et al., Citation2017; Scheimann et al., Citation2019). The HPA axis activity and the fear response in females, however, are enhanced by GR deletion in the prelimbic cortex, which is not observed in males (Scheimann et al., Citation2019). These findings show that the maintenance of normal HPA axis function and the regulation of stress-coping and anxiety-like behaviors can be ascribed to changes in GR signaling in particular regions of the forebrain, while sex-specific modulation of fear responses can be related to GR in glutamatergic circuits of the amygdala and prefrontal cortex. Although the manipulation of GR signaling in particular subpopulations and subregions of the brain allows a better understanding of the diversity of GR responses across large-scale neuronal networks, these approaches lack temporal precision of GR regulation (Myers et al., Citation2014). More work is needed to understand how each GR allosteric form regulates the expression of target genes involved in cytoskeleton dynamics, mitochondrial functions, and synapse formation mediating the described effects of stress (Arango-Lievano et al., Citation2019; Jeanneteau et al., Citation2018; Liston et al., Citation2013; Swanson et al., Citation2013).
The diversity of GR responses is conditioned, at the time of the event, by the activity of brain-wide neural networks. One example of a molecular mediator involved in orchestrating the diversity of GR behavioral responses is the activity-dependent neurotrophic factor BDNF (Mitre et al., Citation2017). Indeed, BDNF signaling is negatively regulated by glucocorticoids and the effects of glucocorticoids on synaptic plasticity in the brain are regulated by BDNF (Rothman & Mattson, Citation2013). The influence of BDNF on GRs may rely on an activity-dependent release of BDNF. Mouse carriers of the BDNF genetic variant Val66Met exhibit behaviors and stress-response improper under both basal and stressful conditions (Arango-Lievano et al., Citation2019; Autry & Monteggia, Citation2012; Chen et al., Citation2006; Nasca et al., Citation2015; Notaras et al., Citation2016, Citation2017; Soliman et al., Citation2010; Yu et al., Citation2012). Glucocorticoids and BDNF exert complementary roles on behaviors (e.g. avoidance, fear, stress-coping, and impulse control) as well as on synaptic plasticity (Barfield & Gourley, Citation2018; Jeanneteau et al., Citation2019; Jeanneteau & Chao, Citation2013). One mechanism operates via changes of GR phosphorylation and signaling (Arango-Lievano et al., Citation2015a; Lambert et al., Citation2013; McEwen, Citation2015). The interdependent actions of BDNF and GR allow the formation of new memories of contextual fear, the maintenance of procedural memories and stress-coping strategies (Arango-Lievano et al., Citation2019; Blugeot et al., Citation2011; Chen et al., Citation2012a; Gourley et al., Citation2012; Revest et al., Citation2014). This was shown by conditioning GR responses in experience-dependent neuronal networks, while preserving the diversity of GR responses in networks unrelated to the task (Jeanneteau & Chao, Citation2013). Thus, the specific effects of BDNF on GR signaling could produce diverse GR allosteric phospho-isoforms and chromatin landscapes in a mosaic of cell assemblies, which could contribute to a diversity of stress-coping behaviors.
As previously outlined, the individual variability in susceptibility to stress-related disorders results from a complex interplay between stress perception, context-dependent factors (e.g. genetic predisposition, history of stress such as early-life trauma or stressful life events) and changes in BDNF and GR signaling pathways across large-scale brain networks. The capacity of an organism to deal with a chronic activation of the HPA axis and therefore with an increased allostatic load, determines the extent of behavioral, physiological, neuroendocrine, and neuronal damages in brain regions regulating reward, fear, emotionality and social behavior ().
4.2. A disease perspective
A normally well-tolerated stressor can become pathogenic if the glucocorticoid reactivity is inappropriate (Selye, Citation1956). The chronic deviation of the regulatory systems, away from homeostasis, by prolonged exposure to environmental or psychological stressors, can produce maladaptive allostatic loads (Myers et al., Citation2014; Picard et al., Citation2014). For example, in the context of drug abuse, the allostatic state is the deviation of a reward set-point, due to a dysregulation in brain reward mechanisms and stress responses, enhancing the vulnerability to drug-related behaviors (Koob & Le Moal, Citation2001).
4.2.1. At the molecular level
Pathological shifts in key mediators of the allostatic load include dysregulation of the HPA axis, decreased GR responsiveness, dysfunctional immune responses, chronic inflammation, increased oxidative stress and decreased neuronal survival due in part, to a reduction in neurotrophic support (McEwen, Citation2006). Behavioral and physiological functions orchestrated by the brain during stress highly depend on the complementary actions of glucocorticoid and BDNF signaling pathways in the mesolimbic and corticolimbic neural networks. Maintenance of the activity in the reward- and emotion-related networks has been shown to guide behavioral responses that are in line with the costs and benefits of the contextual demands (Vinckier et al., Citation2018). For example, lack of motivation and inflexible maladaptive responses likely develop when prefrontal BDNF-TrkB signaling is compromised and GR-mediated actions are desensitized. On the contrary, sensitization to reward or aversive conditioning results when mesolimbic BDNF-TrkB signaling and GR-mediated responses are upregulated (Barfield & Gourley, Citation2018; Jeanneteau et al., Citation2019). Desynchronization of BDNF-TrkB and glucocorticoid-activated GR signaling pathways, in multiple neural networks, may contribute to increasing the vulnerability to stress-induced disorders. Desensitization of GR viewed as a decrease of ligand binding or a reduction in its ability to act as a detector of coincident BDNF signaling is likely a key determinant in promoting pathological disorders advanced by inappropriate stress responses.
4.2.2. At the network level
It is not fully understood why the stress response can become maladaptive and how it could be reversed. One difference between adaptive and maladaptive pathological responses may involve the duration of exposure to stressors and its context. Indeed, the interaction of the stressor with the activity of large-scale brain networks may predict future outcomes and avoid or reduce uncertainty. Although alterations in BDNF signaling vary from region to region in the brain depending on the stressor paradigms, coherence between several brain regions emerged (for review, see Barfield & Gourley, Citation2018; Jeanneteau et al., Citation2019; McEwen et al., Citation2016). For example, BDNF levels, TrkB activation, GR responsiveness, and glucocorticoid spine plasticity decreased in both the hippocampus and prefrontal cortex, or increased in both the amygdala and ventral tegmental area (VTA) depending on the nature and intensity of the stressor (Chattarji et al., Citation2015). A physical stressor producing depression-like phenotypes and impairing fear responses is associated with significant alterations in BDNF levels and trafficking as well as synaptic plasticity and neurotransmitter release (Biggio et al., Citation2019; Deng et al., Citation2017; Liu et al., Citation2018; Mallei et al., Citation2019; Nasrallah et al., Citation2019; Tornese et al., Citation2019). A social stressor producing depression-like phenotypes and impairing fear responses is associated with elevated BDNF levels, TrkB activity and GR responsiveness as well as synaptic plasticity in the amygdala and the VTA-projections of the nucleus accumbens (Der-Avakian et al., Citation2014; Rattiner et al., Citation2004a, Citation2004b; Wook Koo et al., Citation2016). New insights in the correlated functional and structural changes in both the hippocampus and prefrontal cortex or in both the amygdala and VTA could be gained, for example, by investigating the behavioral, neuroendocrine, and neuronal phenotypes of mice lacking the BDNF-sensitive GR phosphorylation sites in these critical brain areas. Indeed, diversity in responses from large-scale brain networks may influence the GR responsivity to environmental changes potentially leading to pathological states.
4.2.3. Susceptibility vs. Resilience
Similar to humans (Charney, Citation2004), not all animals exposed to chronic stressors develop depression-like phenotypes (i.e. anhedonia, passive coping strategies, or social avoidance). The heterogeneity in individual behaviors may mask the effects of chronic stress on BDNF and brain plasticity. For example, low or high hippocampal BDNF levels predict susceptibility or resilience to develop depression-like symptomatology in response to inescapable chronic stress paradigms (Blugeot et al., Citation2011). Indeed, although both susceptible and resilient animals typically exhibit alterations in HPA axis activity and increased anxiety-like behaviors following exposure to chronic or social defeat stressors, only the susceptible animals display increased depression-like behaviors and show alterations in BDNF signaling and synaptic plasticity in the hippocampus and prefrontal cortex (Akimoto et al., Citation2019; Bergstrom et al., Citation2008; Farhang et al., Citation2014; Mallei et al., Citation2019; Stepanichev et al., Citation2018). Interestingly, both the susceptible and resilient animals to social subordination show alterations in BDNF signaling in the VTA mesolimbic circuitry (Der-Avakian et al., Citation2014). Yet, only susceptible animals had increased BDNF levels in the nucleus accumbens (Krishnan et al., Citation2007), which may be due to enhanced activity-dependent BDNF release from dopaminergic neurons (Krishnan et al., Citation2007). Together, these studies highlight an important role of hippocampal, prefrontal, and accumbal BDNF in the functionality of neural circuits controlling stress adaptation and emotional stability (Taliaz et al., Citation2011). BDNF is a key transducer of antidepressant treatment effects, including the regulation of GR sensitivity, HPA axis reactivity to stress, glutamate neurotransmission, synaptic strength, brain connectivity and behavior (Bjorkholm & Monteggia, Citation2016; Castren & Antila, Citation2017; Castren & Rantamaki, Citation2010; Duman et al., Citation2016; Lee et al., Citation2016). Therefore, impairments in the crosstalk between BDNF and GR signaling pathways may be at the foundation for vulnerability or resiliency to stress-induced pathologies (Castren & Antila, Citation2017; Pittenger & Duman, Citation2008). Further research is needed to establish a better picture of how the vulnerability or resilience to adversity might be predicted by the diversity of BDNF or GR actions.
4.2.4. Gene × Environment interactions
The diversity of stress responses between individuals results from the complex interplay between the genome and the environment (Heim & Binder, Citation2012). Genetic predispositions to stress-related disorders have been related to polymorphisms in genes involved in the feedforward or feedback modulators of glucocorticoid receptors (Derijk, Citation2009). Variations in behavioral profiles depend on the history of gene expression and its dynamics to changes in the environment based on prior experience (Clayton et al., Citation2019). For example, dexamethasone challenge of blood immune cells from healthy subjects or post-traumatic stress disorder patients revealed an altered GR responsivity and signaling in the disease (Breen et al., Citation2019). Similar results were obtained from blood leukocytes of patients with major depression compared to healthy controls (Menke et al., Citation2012). In addition to the mechanisms previously mentioned, the diversity of stress responses likely involves epigenetic mechanisms, which mediate the gene expression changes induced by stressful experiences and the subsequent behavioral responses. For example, in the rat brain, stress generates epigenetic signatures linked to changes in HPA axis activity and emotional phenotypes heritable to subsequent generations (Faraji et al., Citation2017). Moreover, the epigenetic regulation of Nr3c1 by exposure to early-life stress is associated with increased risk for major depression and suicide in humans, and impaired behavioral and neuroendocrine responses in rats (McGowan et al., Citation2009; Suderman et al., Citation2012). In the context of early-life adversity, the epigenetic reprogramming of Nr3c1, and other regulators of stress sensitivity, is stable, persistent, and can be transmitted to the progeny (Franklin et al., Citation2010; Jawaid et al., Citation2018; Nestler, Citation2016). In contrast, alterations in GR expression in the adult brain upon stress exposure are not stable after the cessation of the stressor, thus, making it unlikely to be responsible for chronic depression-like phenotypes (Alt et al., Citation2010; Barfield & Gourley, Citation2018). The susceptibility to stress-related disorders is particularly associated with changes in epigenetic mechanisms such as DNA methylation and histone modifications primarily localized in the Nr3c1, Nr3c2 and Fkbp5 gene, but also in other components of the HPA axis (e.g. the Crh/Crhr1 system and the Arginine Vasopressin/Avpr1b system) (Ebner & Singewald, Citation2017).
5. Conclusion and future directions
Here, we promote the idea that the diversity of glucocorticoid actions, which depends on context, is essential for maintaining the adaptive responsiveness of select brain networks based on prior experience. This diversity is maintained under the control of the HPA axis, including its physiological glucocorticoid rhythms, and under the control of contextual events across cells and networks, both of which converge to make ligands available to GR and MR in time and space. The response diversity owes a lot to the dynamics of glucocorticoid receptor allostery in a living brain. Although there is a significant advance in our understanding of how glucocorticoid receptors change temporarily and spatially, many questions remain unanswered. Studying glucocorticoid functions in isolation is not sufficient to conclude how stress promotes attention, learning, and adaptation through brain-wide neuronal networks. A better understanding of the mechanisms underlying the diversity of glucocorticoid actions orchestrating the physiological and behavioral responses to environmental challenges (e.g. stress…) is needed with cellular precision and temporal resolution. Future brain-wide single cells transcriptomic and epigenomic profiling studies will be instrumental to discover state-specific signatures of (mal)adaptations, predicting disease trajectories and, thus, could be used for supporting early therapeutic interventions. Moreover, experimental manipulations using optogenetic and chemogenetic approaches during stressful events may provide relevant information on the role of prior experience and ongoing context on GR and MR activation. It will also be of interest to use glucocorticoid ligands that have tissue- or cell-type-specific agonists or antagonist effects, like the selective GR modulators (Viho et al., Citation2019). Finally, neurocircuit studies will identify how the enteroceptive, the default mode, the salient and the prediction error networks communicate to encode the logic for bringing about individual behavioral responsiveness, including differential stress-coping strategies. Here, the hypothetical framework of experience and activity-dependent control of glucocorticoid actions is described on the molecular, cellular, and behavioral scales but with a bias on the prototypical ubiquitous GR and on data testing male rodent models. The following sections intend to broaden this perspective across glucocorticoid receptors and gender.
5.1. The importance of the GR-MR balance
The GR and MR are closely related members of the nuclear receptor superfamily. In addition to a high degree of sequence conservation, GR and MR share overlapping hormone specificity, similarities of binding domain affinity and transcriptional activity at common hormone response elements (Pooley et al., Citation2020). However, GR and MR also display specific properties in terms of distribution, molecular action and ligand specificity (Mifsud & Reul, Citation2018; Pearce, Citation1994) as well as activation patterns in response to ultradian and stress-induced stimulation (Conway-Campbell et al., Citation2007; Mifsud & Reul, Citation2016). Several lines of evidence show that GR and MR cooperatively act to regulate gene expression and physiological processes in co-expressing cells (e.g. neurons, adipocytes, osteoblasts, immune cells, etc. For review, see Kanatsou et al., Citation2019; Koning et al., Citation2019). In the brain, the MR is involved in the detection of challenging and stressful situations, and mediates glucocorticoid effects on behavioral flexibility and emotional memory processing; GR complements these MR actions by promoting memory consolidation and behavioral adaptation (for review see (Kanatsou et al., Citation2019)). Such complementary actions of GR and MR are permitted via distinct transcriptional responses involving AP1 family members, such as cJun and cFos (Pearce, Citation1994). Under conditions of elevated circulating glucocorticoids, however, both GR and MR can bind to GRE located within or close to glucocorticoid target genes, including Fkbp5, which is also involved in the regulation of the GR (reviewed in (Mifsud & Reul, Citation2018)).
The interdependent actions of GR and MR also possibly result from their interaction in the nucleoplasm and at chromatin-associated DNA binding sites occurring during the peak of glucocorticoid oscillation and during the normal physiological stress response (Pooley et al., Citation2020). The precise mechanisms by which GR and MR interact at certain GREs are, however, still unclear. In fact, GR and MR have been classically believed to influence gene regulation, in response to pulsatile basal or stress-evoked glucocorticoid secretion, by binding DNA as homodimers or GR-MR heterodimers (Liu et al., Citation1995; Trapp et al., Citation1994) or through GR receptor self-interactions (Pearce, Citation1994). However, recent work shows that the interaction of GR and MR as true 1:1 heterodimers through D-loop dimerization interface is unlikely and rather suggests allosteric flexibility through oligomerization (Pooley et al., Citation2020; Rivers et al., Citation2019).
The significance of such mechanisms of GR and MR interaction for transcriptional regulation is far from being understood and could contribute to the contextual responses to stress. In addition to complementary actions, GR and MR can exert opposing effects within the same cell type. This is best demonstrated by the excitability of hippocampal CA1 neurons, which are stimulated via MR and suppressed by GR activation (Joels & de Kloet, Citation1990). It is also demonstrated on the plasticity of dendritic spines in vivo, which mGR increases via non-genomic induced spine formation whereas genomic GR and MR promote spine consolidation or elimination depending on circadian and experience-dependent fluctuations of corticosterone (Liston et al., Citation2013; Liston & Gan, Citation2011; Russo et al., Citation2016). In view of their very different effects in the hippocampus, GR and MR have been hypothesized to have unique target genes. In this context, recent work has identified a NeuroD family member binding to an additional motif near the GRE, which seems to drive specificity for MR over GR binding at hippocampal binding sites (Polman et al., Citation2013; van Weert et al., Citation2017). Interestingly, NeuroD proteins have been found differentially expressed in postmortem brain tissue of depressed subjects (Labonté et al., Citation2017). The specific transcriptional responses produced by GR and MR, and their contribution to stress-related diseases are, however, still unknown (reviewed in (Koning et al., Citation2019)). A better understanding of the specific contextual GR- and MR-mediated effects on cellular physiology would help to clarify their exact role in stress-related disorders (de Kloet et al., Citation2016; Kanatsou et al., Citation2019) as well as the relationship between MR function and sex-differences in vulnerability to these diseases (Klok et al., Citation2011a, Citation2011b; Vinkers et al., Citation2015).
5.2. A critical role of sex differences
Preclinical research demonstrated significant sex differences in HPA axis function and regulation, both at the level of hypothalamic and pituitary activation and at the level of negative feedback on the HPA axis via GR and its co-chaperones. For example, female rodents consistently display increased basal levels of corticosterone and adrenocorticotrophic hormone, increased CRH sensitivity, higher HPA axis activation in response to physical and psychological stressors and lower negative feedback (for review, see Bangasser & Valentino, Citation2014; Hillerer et al., Citation2019; Kudielka & Kirschbaum, Citation2005; Rincon-Cortes et al., Citation2019). Sex differences in stress reactivity have been largely attributed to the modulation of HPA axis function by gonadal hormones (for review, see Bangasser & Wiersielis, Citation2018; Bourke et al., Citation2012). Sex differences in HPA axis function and regulation have been associated with sexually dimorphic physiological and behavioral stress responses as well as distinct patterns of stress-induced neuronal activity, morphology, connectivity and gene expression patterns in several corticolimbic brain areas (for review, see Bourke et al., Citation2012; Brivio et al., Citation2020). Sex differences in transcriptional profiles are observed for both stress-related genes such as Nr3c1 (encoding the GR), Crh and genes of the oxytocin/arginine-vasopressin pathway, and activity-dependent genes such as Bdnf (reviewed in (Brivio et al., Citation2020)). In contrast to rodent studies, there is a lack of clear evidence for gender differences in cortisol reactivity and measures of autonomic response to stressors in humans. However, women consistently report more distress to stressful experiences as well as more depressive and anxiety-related symptoms than men (for review, see Brivio et al., Citation2020; Hillerer et al., Citation2019). These observations are in line with greater susceptibility toward affective dysfunction in women (Altemus, Citation2006; Solomon & Herman, Citation2009).
The molecular mechanisms driving the described sex differences in stress responsiveness are unclear, but may be brought about, at least partially, from different coping strategies in response to fear-inducing and stressful experiences. In this regard, it has been proposed that the female response to a stressor can take either the traditional form of “fight or flight” or an alternative response pattern of “tend and befriend” (Taylor et al., Citation2000). The evidence for this theoretical framework comes from animal studies that have reported sexually dimorphic expression of stress-coping behaviors and the underlying neurobiological mechanisms (Dalla et al., Citation2008a, Citation2008b, Citation2009; Goel et al., Citation2014; Kent et al., Citation2017; Klein et al., Citation1998)). Sexually dimorphic stress-coping strategies and sex differences in the endocrinological response to stressors at multiple levels may represent key factors in the increased vulnerability of women to develop stress-related disorders such as depression, anxiety and post-traumatic stress disorder (for review, see Bangasser & Wiersielis, Citation2018; Bourke et al., Citation2012; Brivio et al., Citation2020). Yet, additional work is needed to understand the molecular mechanisms involving the contextual GR and MR signaling, which underlie sex differences in stress responses and gender disparities in the epidemiology of affective disorders.
5.3. Important considerations for future directions
Here, we emphasize that glucocorticoids provide contextual signals that interact with ongoing activity signals, like BDNF. Such combination of signals, at a specific moment in time, depends on the molecular, cellular, and behavioral states to induce the effects tailored to neuronal network demands. Elucidating such complexity will greatly improve our understanding of stress adaptation processes. Given the critical role that context plays during the stress responses, several questions arise: what does peripheral injection of glucocorticoid (or intake as mainstay of treatment) mean in the absence of context? Should we re-consider the use of passive modulation of the glucocorticoid system to study behavior? Should we move away from chronic glucocorticoid models of depression? Answers to these questions will require temporal resolution and cellular precision to analyze contextual signaling of GR and MR on the molecular, cellular, and behavioral scales. Future strategies will use the tools from the reverse genetic arsenal to gain control of contextual glucocorticoid actions in engram neurons for multi-category investigations of behavioral dynamics and diseases trajectories.
Disclosure statement
The authors have no conflicts of interest to declare.
Additional information
Funding
References
- Adzic, M., Djordjevic, J., Djordjevic, A., Niciforovic, A., Demonacos, C., Radojcic, M., & Krstic-Demonacos, M. (2009). Acute or chronic stress induce cell compartment-specific phosphorylation of glucocorticoid receptor and alter its transcriptional activity in Wistar rat brain. The Journal of Endocrinology, 202(1), 87–97. https://doi.org/10.1677/JOE-08-0509
- Akimoto, H., Oshima, S., Sugiyama, T., Negishi, A., Nemoto, T., & Kobayashi, D. (2019). Changes in brain metabolites related to stress resilience: Metabolomic analysis of the hippocampus in a rat model of depression. Behavioural Brain Research, 359, 342–352. https://doi.org/10.1016/j.bbr.2018.11.017
- Alt, S. R., Turner, J. D., Klok, M. D., Meijer, O. C., Lakke, E. A., Derijk, R. H., & Muller, C. P. (2010). Differential expression of glucocorticoid receptor transcripts in major depressive disorder is not epigenetically programmed. Psychoneuroendocrinology, 35(4), 544–556. https://doi.org/10.1016/j.psyneuen.2009.09.001
- Altarejos, J. Y., & Montminy, M. (2011). CREB and the CRTC co-activators: Sensors for hormonal and metabolic signals. Nature Reviews. Molecular Cell Biology, 12(3), 141–151. https://doi.org/10.1038/nrm3072
- Altemus, M. (2006). Sex differences in depression and anxiety disorders: Potential biological determinants. Hormones and Behavior, 50(4), 534–538. https://doi.org/10.1016/j.yhbeh.2006.06.031
- Ambroggi, F., Turiault, M., Milet, A., Deroche-Gamonet, V., Parnaudeau, S., Balado, E., Barik, J., van der Veen, R., Maroteaux, G., Lemberger, T., Schütz, G., Lazar, M., Marinelli, M., Piazza, P. V., & Tronche, F. (2009). Stress and addiction: Glucocorticoid receptor in dopaminoceptive neurons facilitates cocaine seeking. Nature Neuroscience, 12(3), 247–249. https://doi.org/10.1038/nn.2282
- Arango-Lievano, M., Borie, A. M., Dromard, Y., Murat, M., Desarmenien, M. G., Garabedian, M. J., & Jeanneteau, F. (2019). Persistence of learning-induced synapses depends on neurotrophic priming of glucocorticoid receptors. Proceedings of the National Academy of Sciences of the United States of America, 116(26), 13097–13106. https://doi.org/10.1073/pnas.1903203116
- Arango-Lievano, M., & Jeanneteau, F. (2016). Timing and crosstalk of glucocorticoid signaling with cytokines, neurotransmitters and growth factors. Pharmacological Research, 113(Pt A), 1–17. https://doi.org/10.1016/j.phrs.2016.08.005
- Arango-Lievano, M., Lambert, W. M., & Jeanneteau, F. (2015b). Molecular biology of glucocorticoid signaling. Advances in Experimental Medicine and Biology, 872, 33–57. https://doi.org/10.1007/978-1-4939-2895-8_2
- Arango-Lievano, M., Lambert, W. M., Bath, K. G., Garabedian, M. J., Chao, M. V., & Jeanneteau, F. (2015a). Neurotrophic-priming of glucocorticoid receptor signaling is essential for neuronal plasticity to stress and antidepressant treatment. Proceedings of the National Academy of Sciences of the United States of America, 112(51), 15737–15742. https://doi.org/10.1073/pnas.1509045112
- Arango-Lievano, M., Peguet, C., Catteau, M., Parmentier, M. L., Wu, S., Chao, M. V., Ginsberg, S. D., & Jeanneteau, F. (2016). Deletion of neurotrophin signaling through the glucocorticoid receptor pathway causes tau neuropathology. Scientific Reports, 6, 37231. https://doi.org/10.1038/srep37231
- Arnett, M. G., Muglia, L. M., Laryea, G., & Muglia, L. J. (2016). Genetic approaches to hypothalamic-pituitary-adrenal axis regulation. Neuropsychopharmacology : Official Publication of the American College of Neuropsychopharmacology, 41(1), 245–260. https://doi.org/10.1038/npp.2015.215
- Autry, A. E., & Monteggia, L. M. (2012). Brain-derived neurotrophic factor and neuropsychiatric disorders. Pharmacological Reviews, 64(2), 238–258. https://doi.org/10.1124/pr.111.005108
- Avey, D., Sankararaman, S., Yim, A. K. Y., Barve, R., Milbrandt, J., & Mitra, R. D. (2018). Single-cell RNA-Seq uncovers a robust transcriptional response to morphine by glia. Cell Reports, 24(13), 3619–3629.e4. https://doi.org/10.1016/j.celrep.2018.08.080
- Bangasser, D. A., & Valentino, R. J. (2014). Sex differences in stress-related psychiatric disorders: Neurobiological perspectives. Frontiers in Neuroendocrinology, 35(3), 303–319. https://doi.org/10.1016/j.yfrne.2014.03.008
- Bangasser, D. A., & Wiersielis, K. R. (2018). Sex differences in stress responses: A critical role for corticotropin-releasing factor. Hormones (Athens, Greece), 17(1), 5–13. https://doi.org/10.1007/s42000-018-0002-z
- Barfield, E. T., & Gourley, S. L. (2018). Prefrontal cortical trkB, glucocorticoids, and their interactions in stress and developmental contexts. Neuroscience & Biobehavioral Reviews, 95, 535–558. https://doi.org/10.1016/j.neubiorev.2018.10.015
- Barford, K., Deppmann, C., & Winckler, B. (2017). The neurotrophin receptor signaling endosome: Where trafficking meets signaling. Developmental Neurobiology, 77(4), 405–418. https://doi.org/10.1002/dneu.22427
- Barik, J., Marti, F., Morel, C., Fernandez, S. P., Lanteri, C., Godeheu, G., Tassin, J. P., Mombereau, C., Faure, P., & Tronche, F. (2013). Chronic stress triggers social aversion via glucocorticoid receptor in dopaminoceptive neurons. Science (New York, N.Y.), 339(6117), 332–335. https://doi.org/10.1126/science.1226767
- Barnes, P. J. (2009). Role of HDAC2 in the pathophysiology of COPD. Annual Review of Physiology, 71, 451–464. https://doi.org/10.1146/annurev.physiol.010908.163257
- Barnes, P. J. (2013). Corticosteroid resistance in patients with asthma and chronic obstructive pulmonary disease. The Journal of Allergy and Clinical Immunology, 131(3), 636–645. https://doi.org/10.1016/j.jaci.2012.12.1564
- Bartlett, A., Lapp, H., & Hunter, R. (2019). Epigenetic mechanisms of the glucocorticoid receptor. Trends in Endocrinology & Metabolism, 30(11), 807–818. https://doi.org/10.1016/j.tem.2019.07.003
- Bergstrom, A., Jayatissa, M. N., Mork, A., & Wiborg, O. (2008). Stress sensitivity and resilience in the chronic mild stress rat model of depression; an in situ hybridization study. Brain Research, 1196, 41–52. https://doi.org/10.1016/j.brainres.2007.12.025
- Biggio, F., Mostallino, M. C., Talani, G., Locci, V., Mostallino, R., Calandra, G., Sanna, E., & Biggio, G. (2019). Social enrichment reverses the isolation-induced deficits of neuronal plasticity in the hippocampus of male rats. Neuropharmacology, 151, 45–54. https://doi.org/10.1016/j.neuropharm.2019.03.030
- Binder, E. B. (2009). The role of FKBP5, a co-chaperone of the glucocorticoid receptor in the pathogenesis and therapy of affective and anxiety disorders. Psychoneuroendocrinology, 34 Suppl 1, S186–S195. https://doi.org/10.1016/j.psyneuen.2009.05.021
- Binder, E. B., Salyakina, D., Lichtner, P., Wochnik, G. M., Ising, M., Pütz, B., Papiol, S., Seaman, S., Lucae, S., Kohli, M. A., Nickel, T., Künzel, H. E., Fuchs, B., Majer, M., Pfennig, A., Kern, N., Brunner, J., Modell, S., Baghai, T., … Muller-Myhsok, B. (2004). Polymorphisms in FKBP5 are associated with increased recurrence of depressive episodes and rapid response to antidepressant treatment. Nature Genetics, 36(12), 1319–1325. https://doi.org/10.1038/ng1479
- Bird, A. (2002). DNA methylation patterns and epigenetic memory. Genes & Development, 16(1), 6–21. https://doi.org/10.1101/gad.947102
- Bjorkholm, C., & Monteggia, L. M. (2016). BDNF – A key transducer of antidepressant effects. Neuropharmacology, 102, 72–79. https://doi.org/10.1016/j.neuropharm.2015.10.034
- Bledsoe, R. K., Montana, V. G., Stanley, T. B., Delves, C. J., Apolito, C. J., McKee, D. D., Consler, T. G., Parks, D. J., Stewart, E. L., Willson, T. M., Lambert, M. H., Moore, J. T., Pearce, K. H., & Xu, H. E. (2002). Crystal structure of the glucocorticoid receptor ligand binding domain reveals a novel mode of receptor dimerization and coactivator recognition. Cell, 110(1), 93–105. https://doi.org/10.1016/S0092-8674(02)00817-6
- Blugeot, A., Rivat, C., Bouvier, E., Molet, J., Mouchard, A., Zeau, B., Bernard, C., Benoliel, J. J., & Becker, C. (2011). Vulnerability to depression: From brain neuroplasticity to identification of biomarkers. The Journal of Neuroscience: the Official Journal of the Society for Neuroscience, 31(36), 12889–12899. https://doi.org/10.1523/JNEUROSCI.1309-11.2011
- Boivin, J. R., & Nedivi, E. (2018). Functional implications of inhibitory synapse placement on signal processing in pyramidal neuron dendrites. Current Opinion in Neurobiology, 51, 16–22. https://doi.org/10.1016/j.conb.2018.01.013
- Bourke, C. H., Harrell, C. S., & Neigh, G. N. (2012). Stress-induced sex differences: Adaptations mediated by the glucocorticoid receptor. Hormones and Behavior, 62(3), 210–218. https://doi.org/10.1016/j.yhbeh.2012.02.024
- Bousiges, O., Neidl, R., Majchrzak, M., Muller, M. A., Barbelivien, A., Pereira de Vasconcelos, A., Schneider, A., Loeffler, J. P., Cassel, J. C., & Boutillier, A. L. (2013). Detection of histone acetylation levels in the dorsal hippocampus reveals early tagging on specific residues of H2B and H4 histones in response to learning. PLoS One, 8(3), e57816. https://doi.org/10.1371/journal.pone.0057816
- Boyle, M. P., Brewer, J. A., Funatsu, M., Wozniak, D. F., Tsien, J. Z., Izumi, Y., & Muglia, L. J. (2005). Acquired deficit of forebrain glucocorticoid receptor produces depression-like changes in adrenal axis regulation and behavior. Proceedings of the National Academy of Sciences of the United States of America, 102(2), 473–478. https://doi.org/10.1073/pnas.0406458102
- Boyle, M. P., Kolber, B. J., Vogt, S. K., Wozniak, D. F., & Muglia, L. J. (2006). Forebrain glucocorticoid receptors modulate anxiety-associated locomotor activation and adrenal responsiveness. The Journal of Neuroscience : The Official Journal of the Society for Neuroscience, 26(7), 1971–1978. https://doi.org/10.1523/JNEUROSCI.2173-05.2006
- Breen, M. S., Bierer, L. M., Daskalakis, N. P., Bader, H. N., Makotkine, I., Chattopadhyay, M., Xu, C., Buxbaum Grice, A., Tocheva, A. S., Flory, J. D., Buxbaum, J. D., Meaney, M. J., Brennand, K., & Yehuda, R. (2019). Differential transcriptional response following glucocorticoid activation in cultured blood immune cells: A novel approach to PTSD biomarker development. Translational Psychiatry, 9(1), 201. https://doi.org/10.1038/s41398-019-0539-x
- Brivio, E., Lopez, J. P., & Chen, A. (2020). Sex differences: Transcriptional signatures of stress exposure in male and female brains. Genes, Brain, and Behavior, 19, e12643.
- Broekema, M. F., Hollman, D. A. A., Koppen, A., van den Ham, H. J., Melchers, D., Pijnenburg, D., Ruijtenbeek, R., van Mil, S. W. C., Houtman, R., & Kalkhoven, E. (2018). Profiling of 3696 nuclear receptor-coregulator interactions: A resource for biological and clinical discovery. Endocrinology, 159(6), 2397–2407. https://doi.org/10.1210/en.2018-00149
- Burd, C. J., Ward, J. M., Crusselle-Davis, V. J., Kissling, G. E., Phadke, D., Shah, R. R., & Archer, T. K. (2012). Analysis of chromatin dynamics during glucocorticoid receptor activation. Molecular and Cellular Biology, 32(10), 1805–1817. https://doi.org/10.1128/MCB.06206-11
- Castren, E., & Antila, H. (2017). Neuronal plasticity and neurotrophic factors in drug responses. Molecular Psychiatry, 22(8), 1085–1095. https://doi.org/10.1038/mp.2017.61
- Castren, E., & Rantamaki, T. (2010). The role of BDNF and its receptors in depression and antidepressant drug action: Reactivation of developmental plasticity. Developmental Neurobiology, 70, 289–297.
- Chameau, P., Qin, Y., Spijker, S., Smit, A. B., Smit, G., & Joëls, M. (2007). Glucocorticoids specifically enhance L-type calcium current amplitude and affect calcium channel subunit expression in the mouse hippocampus. Journal of Neurophysiology, 97(1), 5–14. https://doi.org/10.1152/jn.00821.2006
- Charney, D. S. (2004). Psychobiological mechanisms of resilience and vulnerability: Implications for successful adaptation to extreme stress. The American Journal of Psychiatry, 161(2), 195–216. https://doi.org/10.1176/appi.ajp.161.2.195
- Chater, T. E., & Goda, Y. (2014). The role of AMPA receptors in postsynaptic mechanisms of synaptic plasticity. Frontiers in Cellular Neuroscience, 8, 401. https://doi.org/10.3389/fncel.2014.00401
- Chattarji, S., Tomar, A., Suvrathan, A., Ghosh, S., & Rahman, M. M. (2015). Neighborhood matters: Divergent patterns of stress-induced plasticity across the brain. Nature Neuroscience, 18(10), 1364–1375. https://doi.org/10.1038/nn.4115
- Chen, C. C., Lu, J., Yang, R., Ding, J. B., & Zuo, Y. (2018). Selective activation of parvalbumin interneurons prevents stress-induced synapse loss and perceptual defects. Molecular Psychiatry, 23(7), 1614–1625. https://doi.org/10.1038/mp.2017.159
- Chen, D. Y., Bambah-Mukku, D., Pollonini, G., & Alberini, C. M. (2012a). Glucocorticoid receptors recruit the CaMKIIα-BDNF-CREB pathways to mediate memory consolidation. Nature Neuroscience, 15(12), 1707–1714. https://doi.org/10.1038/nn.3266
- Chen, J. L., Villa, K. L., Cha, J. W., So, P. T., Kubota, Y., & Nedivi, E. (2012b). Clustered dynamics of inhibitory synapses and dendritic spines in the adult neocortex. Neuron, 74(2), 361–373. https://doi.org/10.1016/j.neuron.2012.02.030
- Chen, K., Zhang, L., Tan, M., Lai, C. S., Li, A., Ren, C., & So, K. F. (2017). Treadmill exercise suppressed stress-induced dendritic spine elimination in mouse barrel cortex and improved working memory via BDNF/TrkB pathway. Translational Psychiatry, 7(3), e1069. https://doi.org/10.1038/tp.2017.41
- Chen, Z.-Y., Jing, D., Bath, K. G., Ieraci, A., Khan, T., Siao, C.-J., Herrera, D. G., Toth, M., Yang, C., McEwen, B. S., Hempstead, B. L., & Lee, F. S. (2006). Genetic variant BDNF (Val66Met) polymorphism alters anxiety-related behavior. Science (New York, N.Y.), 314(5796), 140–143. https://doi.org/10.1126/science.1129663
- Choi, G. E., Oh, J. Y., Lee, H. J., Chae, C. W., Kim, J. S., Jung, Y. H., & Han, H. J. (2018). Glucocorticoid-mediated ER-mitochondria contacts reduce AMPA receptor and mitochondria trafficking into cell terminus via microtubule destabilization. Cell Death Dis, 9(11), 1137. https://doi.org/10.1038/s41419-018-1172-y
- Cichon, J., & Gan, W. B. (2015). Branch-specific dendritic Ca(2+) spikes cause persistent synaptic plasticity. Nature, 520(7546), 180–185. https://doi.org/10.1038/nature14251
- Clayton, D. F., Anreiter, I., Aristizabal, M., Frankland, P. W., Binder, E. B., & Citri, A. (2019). The role of the genome in experience-dependent plasticity: Extending the analogy of the genomic action potential. Proc Natl Acad Sci U S A,
- Clemens, B., Wagels, L., Bauchmuller, M., Bergs, R., Habel, U., & Kohn, N. (2017). Alerted default mode: Functional connectivity changes in the aftermath of social stress. Scientific Reports, 7, 40180. https://doi.org/10.1038/srep40180
- Conboy, L., & Sandi, C. (2010). Stress at learning facilitates memory formation by regulating AMPA receptor trafficking through a glucocorticoid action. Neuropsychopharmacology : Official Publication of the American College of Neuropsychopharmacology, 35(3), 674–685. https://doi.org/10.1038/npp.2009.172
- Conway-Campbell, B. L., McKenna, M. A., Wiles, C. C., Atkinson, H. C., de Kloet, E. R., & Lightman, S. L. (2007). Proteasome-dependent down-regulation of activated nuclear hippocampal glucocorticoid receptors determines dynamic responses to corticosterone. Endocrinology, 148(11), 5470–5477. https://doi.org/10.1210/en.2007-0585
- Conway-Campbell, B. L., Pooley, J. R., Hager, G. L., & Lightman, S. L. (2012). Molecular dynamics of ultradian glucocorticoid receptor action. Molecular and Cellular Endocrinology, 348(2), 383–393. https://doi.org/10.1016/j.mce.2011.08.014
- Crosio, C., Heitz, E., Allis, C. D., Borrelli, E., & Sassone-Corsi, P. (2003). Chromatin remodeling and neuronal response: Multiple signaling pathways induce specific histone H3 modifications and early gene expression in hippocampal neurons. Journal of Cell Science, 116(Pt 24), 4905–4914. https://doi.org/10.1242/jcs.00804
- Crow, M., Lim, N., Ballouz, S., Pavlidis, P., & Gillis, J. (2019). Predictability of human differential gene expression. Proceedings of the National Academy of Sciences of the United States of America, 116(13), 6491–6500. https://doi.org/10.1073/pnas.1802973116
- Dalla, C., Antoniou, K., Kokras, N., Drossopoulou, G., Papathanasiou, G., Bekris, S., Daskas, S., & Papadopoulou-Daifoti, Z. (2008a). Sex differences in the effects of two stress paradigms on dopaminergic neurotransmission. Physiology & Behavior, 93(3), 595–605. https://doi.org/10.1016/j.physbeh.2007.10.020
- Dalla, C., Edgecomb, C., Whetstone, A. S., & Shors, T. J. (2008b). Females do not express learned helplessness like males do. Neuropsychopharmacology : Official Publication of the American College of Neuropsychopharmacology, 33(7), 1559–1569. https://doi.org/10.1038/sj.npp.1301533
- Dalla, C., Whetstone, A. S., Hodes, G. E., & Shors, T. J. (2009). Stressful experience has opposite effects on dendritic spines in the hippocampus of cycling versus masculinized females. Neuroscience Letters, 449(1), 52–56. https://doi.org/10.1016/j.neulet.2008.10.051
- Datson, N. A., Polman, J. A., de Jonge, R. T., van Boheemen, P. T., van Maanen, E. M., Welten, J., McEwen, B. S., Meiland, H. C., & Meijer, O. C. (2011). Specific regulatory motifs predict glucocorticoid responsiveness of hippocampal gene expression. Endocrinology, 152(10), 3749–3757. https://doi.org/10.1210/en.2011-0287
- de Assis, G. G., & Gasanov, E. V. (2019). BDNF and Cortisol integrative system – Plasticity vs. degeneration: Implications of the Val66Met polymorphism. Frontiers in Neuroendocrinology, 55, 100784. https://doi.org/10.1016/j.yfrne.2019.100784
- de Boer, S. F., Buwalda, B., & Koolhaas, J. M. (2017). Untangling the neurobiology of coping styles in rodents: Towards neural mechanisms underlying individual differences in disease susceptibility. Neuroscience and Biobehavioral Reviews, 74(Pt B), 401–422. https://doi.org/10.1016/j.neubiorev.2016.07.008
- de Kloet, E. R., Otte, C., Kumsta, R., Kok, L., Hillegers, M. H., Hasselmann, H., Kliegel, D., & Joels, M. (2016). Stress and depression: A crucial role of the mineralocorticoid receptor. Journal of Neuroendocrinology, 28(8). https://doi.org/10.1111/jne.12379
- De Kloet, E. R., Vreugdenhil, E., Oitzl, M. S., & Joels, M. (1998). Brain corticosteroid receptor balance in health and disease. Endocrine Reviews, 19(3), 269–301. https://doi.org/10.1210/er.19.3.269
- Deinhardt, K., & Jeanneteau, F. (2012). More than just an OFF-switch: The essential role of protein dephosphorylation in the modulation of BDNF signaling events. In C. Huang (Ed.), Protein phosphorylation in human health. InTechOpen.
- Deisseroth, K., Mermelstein, P. G., Xia, H., & Tsien, R. W. (2003). Signaling from synapse to nucleus: The logic behind the mechanisms. Current Opinion in Neurobiology, 13(3), 354–365. https://doi.org/10.1016/S0959-4388(03)00076-X
- Deng, J.-H., Yan, W., Han, Y., Chen, C., Meng, S.-Q., Sun, C.-Y., Xu, L.-Z., Xue, Y.-X., Gao, X.-J., Chen, N., Zhang, F.-L., Wang, Y.-M., Shi, J., & Lu, L. (2017). Predictable chronic mild stress during adolescence promotes fear memory extinction in adulthood. Scientific Reports, 7(1), 7857. https://doi.org/10.1038/s41598-017-08017-7
- Der-Avakian, A., Mazei-Robison, M. S., Kesby, J. P., Nestler, E. J., & Markou, A. (2014). Enduring deficits in brain reward function after chronic social defeat in rats: Susceptibility, resilience, and antidepressant response. Biological Psychiatry, 76(7), 542–549. https://doi.org/10.1016/j.biopsych.2014.01.013
- Derijk, R. H. (2009). Single nucleotide polymorphisms related to HPA axis reactivity. Neuroimmunomodulation, 16(5), 340–352. https://doi.org/10.1159/000216192
- Deroche, V., Marinelli, M., Maccari, S., Le Moal, M., Simon, H., & Piazza, P. V. (1995). Stress-induced sensitization and glucocorticoids. I. Sensitization of dopamine-dependent locomotor effects of amphetamine and morphine depends on stress-induced corticosterone secretion. The Journal of Neuroscience : The Official Journal of the Society for Neuroscience, 15(11), 7181–7188. https://doi.org/10.1523/JNEUROSCI.15-11-07181.1995
- Deroche-Gamonet, V., Sillaber, I., Aouizerate, B., Izawa, R., Jaber, M., Ghozland, S., Kellendonk, C., Le Moal, M., Spanagel, R., Schütz, G., Tronche, F., & Piazza, P. V. (2003). The glucocorticoid receptor as a potential target to reduce cocaine abuse. The Journal of Neuroscience : The Official Journal of the Society for Neuroscience, 23(11), 4785–4790. https://doi.org/10.1523/JNEUROSCI.23-11-04785.2003
- Di, S., Maxson, M. M., Franco, A., & Tasker, J. G. (2009). Glucocorticoids regulate glutamate and GABA synapse-specific retrograde transmission via divergent nongenomic signaling pathways. The Journal of Neuroscience : The Official Journal of the Society for Neuroscience, 29(2), 393–401. https://doi.org/10.1523/JNEUROSCI.4546-08.2009
- Dias-Ferreira, E., Sousa, J. C., Melo, I., Morgado, P., Mesquita, A. R., Cerqueira, J. J., Costa, R. M., & Sousa, N. (2009). Chronic stress causes frontostriatal reorganization and affects decision-making. Science (New York, N.Y.), 325(5940), 621–625. https://doi.org/10.1126/science.1171203
- Douma, E. H., & de Kloet, E. R. (2020). Stress-induced plasticity and functioning of ventral tegmental dopamine neurons. Neuroscience and Biobehavioral Reviews, 108, 48–77. https://doi.org/10.1016/j.neubiorev.2019.10.015
- Doya, K., Ishii, S., Pouget, A., & Rao, R. (2007). Bayesian brain probabilistic approaches to neural coding. The MIT Press.
- Du, J., Wang, Y., Hunter, R., Wei, Y., Blumenthal, R., Falke, C., Khairova, R., Zhou, R., Yuan, P., Machado-Vieira, R., McEwen, B. S., & Manji, H. K. (2009). Dynamic regulation of mitochondrial function by glucocorticoids. Proceedings of the National Academy of Sciences of the United States of America, 106(9), 3543–3548. https://doi.org/10.1073/pnas.0812671106
- Duman, R. S., Aghajanian, G. K., Sanacora, G., & Krystal, J. H. (2016). Synaptic plasticity and depression: New insights from stress and rapid-acting antidepressants. Nature Medicine, 22(3), 238–249. https://doi.org/10.1038/nm.4050
- Ebner, K., & Singewald, N. (2017). Individual differences in stress susceptibility and stress inhibitory mechanisms. Current Opinion in Behavioral Sciences, 14, 54–64. https://doi.org/10.1016/j.cobeha.2016.11.016
- Egan, M. F., Kojima, M., Callicott, J. H., Goldberg, T. E., Kolachana, B. S., Bertolino, A., Zaitsev, E., Gold, B., Goldman, D., Dean, M., Lu, B., & Weinberger, D. R. (2003). The BDNF val66met polymorphism affects activity-dependent secretion of BDNF and human memory and hippocampal function. Cell, 112(2), 257–269. https://doi.org/10.1016/S0092-8674(03)00035-7
- Ernst, M., Pine, D. S., & Hardin, M. (2006). Triadic model of the neurobiology of motivated behavior in adolescence. Psychological Medicine, 36(3), 299–312. https://doi.org/10.1017/S0033291705005891
- Faraji, J., Soltanpour, N., Lotfi, H., Moeeini, R., Moharreri, A.-R., Roudaki, S., Hosseini, S. A., Olson, D. M., Abdollahi, A.-A., Soltanpour, N., Mohajerani, M. H., & Metz, G. A. S. (2017). Lack of social support raises stress vulnerability in rats with a history of ancestral stress. Scientific Reports, 7(1), 5277. https://doi.org/10.1038/s41598-017-05440-8
- Faresse, N. (2014). Post-translational modifications of the mineralocorticoid receptor: How to dress the receptor according to the circumstances? Journal of Steroid Biochemistry and Molecular Biology, 143, 334–342. https://doi.org/10.1016/j.jsbmb.2014.04.015
- Farhang, S., Barar, J., Fakhari, A., Mesgariabbasi, M., Khani, S., Omidi, Y., & Farnam, A. (2014). Asymmetrical expression of BDNF and NTRK3 genes in frontoparietal cortex of stress-resilient rats in an animal model of depression. Synapse (New York, N.Y.), 68(9), 387–393. https://doi.org/10.1002/syn.21746
- Fenzl, T., Touma, C., Romanowski, C. P., Ruschel, J., Holsboer, F., Landgraf, R., Kimura, M., & Yassouridis, A. (2011). Sleep disturbances in highly stress reactive mice: Modeling endophenotypes of major depression. BMC Neuroscience, 12, 29. https://doi.org/10.1186/1471-2202-12-29
- Franco, L. M., Gadkari, M., Howe, K. N., Sun, J., Kardava, L., Kumar, P., Kumari, S., Hu, Z., Fraser, I. D. C., Moir, S., Tsang, J. S., & Germain, R. N. (2019). Immune regulation by glucocorticoids can be linked to cell type-dependent transcriptional responses. The Journal of Experimental Medicine, 216(2), 384–406. https://doi.org/10.1084/jem.20180595
- Franklin, T. B., Russig, H., Weiss, I. C., Graff, J., Linder, N., Michalon, A., Vizi, S., & Mansuy, I. M. (2010). Epigenetic transmission of the impact of early stress across generations. Biological Psychiatry, 68(5), 408–415. https://doi.org/10.1016/j.biopsych.2010.05.036
- Frey, U., & Morris, R. G. (1997). Synaptic tagging and long-term potentiation. Nature, 385(6616), 533–536. https://doi.org/10.1038/385533a0
- Fries, G. R., Gassen, N. C., Schmidt, U., & Rein, T. (2015). The FKBP51-glucocorticoid receptor balance in stress-related mental disorders. Current Molecular Pharmacology, 9(2), 126–140. https://doi.org/10.2174/1874467208666150519114435
- Fukumoto, K., Morita, T., Mayanagi, T., Tanokashira, D., Yoshida, T., Sakai, A., & Sobue, K. (2009). Detrimental effects of glucocorticoids on neuronal migration during brain development. Molecular Psychiatry, 14(12), 1119–1131. https://doi.org/10.1038/mp.2009.60
- Galigniana, N. M., Ballmer, L. T., Toneatto, J., Erlejman, A. G., Lagadari, M., & Galigniana, M. D. (2012). Regulation of the glucocorticoid response to stress-related disorders by the Hsp90-binding immunophilin FKBP51. Journal of Neurochemistry, 122(1), 4–18. https://doi.org/10.1111/j.1471-4159.2012.07775.x
- Galliher-Beckley, A. J., & Cidlowski, J. A. (2009). Emerging roles of glucocorticoid receptor phosphorylation in modulating glucocorticoid hormone action in health and disease. IUBMB Life, 61(10), 979–986. https://doi.org/10.1002/iub.245
- Galliher-Beckley, A. J., Williams, J. G., & Cidlowski, J. A. (2011). Ligand-independent phosphorylation of the glucocorticoid receptor integrates cellular stress pathways with nuclear receptor signaling. Molecular and Cellular Biology, 31(23), 4663–4675. https://doi.org/10.1128/MCB.05866-11
- Garabedian, M. J., Harris, C. A., & Jeanneteau, F. (2017). Glucocorticoid receptor action in metabolic and neuronal function. F1000Research, 6, 1208. https://doi.org/10.12688/f1000research.11375.1
- Gertz, J., Savic, D., Varley, K. E., Partridge, E. C., Safi, A., Jain, P., Cooper, G. M., Reddy, T. E., Crawford, G. E., & Myers, R. M. (2013). Distinct properties of cell-type-specific and shared transcription factor binding sites. Molecular Cell, 52(1), 25–36. https://doi.org/10.1016/j.molcel.2013.08.037
- Goel, N., Workman, J. L., Lee, T. T., Innala, L., & Viau, V. (2014). Sex differences in the HPA axis. Comprehensive Physiology, 4(3), 1121–1155. https://doi.org/10.1002/cphy.c130054
- Gourley, S. L., Swanson, A. M., Jacobs, A. M., Howell, J. L., Mo, M., Dileone, R. J., Koleske, A. J., & Taylor, J. R. (2012). Action control is mediated by prefrontal BDNF and glucocorticoid receptor binding. Proceedings of the National Academy of Sciences of the United States of America, 109(50), 20714–20719. https://doi.org/10.1073/pnas.1208342109
- Groc, L., Choquet, D., & Chaouloff, F. (2008). The stress hormone corticosterone conditions AMPAR surface trafficking and synaptic potentiation. Nature Neuroscience, 11(8), 868–870. https://doi.org/10.1038/nn.2150
- Groeneweg, F. L., Karst, H., de Kloet, E. R., & Joels, M. (2011). Rapid non-genomic effects of corticosteroids and their role in the central stress response. The Journal of Endocrinology, 209(2), 153–167. https://doi.org/10.1530/JOE-10-0472
- Grontved, L., John, S., Baek, S., Liu, Y., Buckley, J. R., Vinson, C., Aguilera, G., & Hager, G. L. (2013). C/EBP maintains chromatin accessibility in liver and facilitates glucocorticoid receptor recruitment to steroid response elements. The EMBO Journal, 32(11), 1568–1583. https://doi.org/10.1038/emboj.2013.106
- Gutierrez-Mecinas, M., Trollope, A. F., Collins, A., Morfett, H., Hesketh, S. A., Kersante, F., & Reul, J. M. (2011). Long-lasting behavioral responses to stress involve a direct interaction of glucocorticoid receptors with ERK1/2-MSK1-Elk-1 signaling. Proceedings of the National Academy of Sciences of the United States of America, 108(33), 13806–13811. https://doi.org/10.1073/pnas.1104383108
- Hagenston, A. M., & Bading, H. (2011). Calcium signaling in synapse-to-nucleus communication. Cold Spring Harbor Perspectives in Medicine, 3(11), a004564. https://doi.org/10.1101/cshperspect.a004564
- Hapgood, J. P., Avenant, C., & Moliki, J. M. (2016). Glucocorticoid-independent modulation of GR activity: Implications for immunotherapy. Pharmacology & Therapeutics, 165, 93–113. https://doi.org/10.1016/j.pharmthera.2016.06.002
- Harris, C., Weiss, G. L., Di, S., & Tasker, J. G. (2019). Cell signaling dependence of rapid glucocorticoid-induced endocannabinoid synthesis in hypothalamic neuroendocrine cells. Neurobiology of Stress, 10, 100158. https://doi.org/10.1016/j.ynstr.2019.100158
- Hartmann, J., Dedic, N., Pöhlmann, M. L., Häusl, A., Karst, H., Engelhardt, C., Westerholz, S., Wagner, K. V., Labermaier, C., Hoeijmakers, L., Kertokarijo, M., Chen, A., Joëls, M., Deussing, J. M., & Schmidt, M. V. (2017). Forebrain glutamatergic, but not GABAergic, neurons mediate anxiogenic effects of the glucocorticoid receptor. Molecular Psychiatry, 22(3), 466–475. https://doi.org/10.1038/mp.2016.87
- Heim, C., & Binder, E. B. (2012). Current research trends in early life stress and depression: Review of human studies on sensitive periods, gene-environment interactions, and epigenetics. Experimental Neurology, 233(1), 102–111. https://doi.org/10.1016/j.expneurol.2011.10.032
- Heinzmann, J. M., Kloiber, S., Ebling-Mattos, G., Bielohuby, M., Schmidt, M. V., Palme, R., Holsboer, F., Uhr, M., Ising, M., & Touma, C. (2014). Mice selected for extremes in stress reactivity reveal key endophenotypes of major depression: A translational approach. Psychoneuroendocrinology, 49, 229–243. https://doi.org/10.1016/j.psyneuen.2014.07.008
- Herman, J. P. (2013). Neural control of chronic stress adaptation. Frontiers in Behavioral Neuroscience, 7, 61. https://doi.org/10.3389/fnbeh.2013.00061
- Herman, J. P., Figueiredo, H., Mueller, N. K., Ulrich-Lai, Y., Ostrander, M. M., Choi, D. C., & Cullinan, W. E. (2003). Central mechanisms of stress integration: Hierarchical circuitry controlling hypothalamo-pituitary-adrenocortical responsiveness. Frontiers in Neuroendocrinology, 24(3), 151–180. https://doi.org/10.1016/j.yfrne.2003.07.001
- Herman, J. P., McKlveen, J. M., Ghosal, S., Kopp, B., Wulsin, A., Makinson, R., Scheimann, J., & Myers, B. (2016). Regulation of the hypothalamic-pituitary-adrenocortical stress response. Comprehensive Physiology, 6(2), 603–621. https://doi.org/10.1002/cphy.c150015
- Heshmati, M., & Russo, S. J. (2013). Learning to deal with life’s ups and downs. Nature Neuroscience, 16(6), 658–659. https://doi.org/10.1038/nn.3400
- Hill, M. J., Suzuki, S., Segars, J. H., & Kino, T. (2016). CRTC2 is a coactivator of GR and couples GR and CREB in the regulation of hepatic gluconeogenesis. Molecular Endocrinology (Baltimore, Md.), 30(1), 104–117. https://doi.org/10.1210/me.2015-1237
- Hillerer, K. M., Slattery, D. A., & Pletzer, B. (2019). Neurobiological mechanisms underlying sex-related differences in stress-related disorders: Effects of neuroactive steroids on the hippocampus. Frontiers in Neuroendocrinology, 55, 100796. https://doi.org/10.1016/j.yfrne.2019.100796
- Holsboer, F., & Ising, M. (2010). Stress hormone regulation: Biological role and translation into therapy. Annual Review of Psychology, 61, 81–109. C101–111. https://doi.org/10.1146/annurev.psych.093008.100321
- Hudson, W. H., Youn, C., & Ortlund, E. A. (2013). The structural basis of direct glucocorticoid-mediated transrepression. Nature Structural & Molecular Biology, 20(1), 53–58. https://doi.org/10.1038/nsmb.2456
- Huzard, D., Vouros, A., Monari, S., Astori, S., Vasilaki, E., & Sandi, C. (2020). Constitutive differences in glucocorticoid responsiveness are related to divergent spatial information processing abilities. Stress (Amsterdam, Netherlands), 23(1), 37–13. https://doi.org/10.1080/10253890.2019.1625885
- Ishmael, F. T., Fang, X., Houser, K. R., Pearce, K., Abdelmohsen, K., Zhan, M., Gorospe, M., & Stellato, C. (2011). The human glucocorticoid receptor as an RNA-binding protein: Global analysis of glucocorticoid receptor-associated transcripts and identification of a target RNA motif. Journal of Immunology (Baltimore, Md. : 1950), 186(2), 1189–1198. https://doi.org/10.4049/jimmunol.1001794
- Ismaili, N., & Garabedian, M. J. (2004). Modulation of glucocorticoid receptor function via phosphorylation. Annals of the New York Academy of Sciences, 1024, 86–101. https://doi.org/10.1196/annals.1321.007
- Ito, K., Yamamura, S., Essilfie-Quaye, S., Cosio, B., Ito, M., Barnes, P. J., & Adcock, I. M. (2006). Histone deacetylase 2-mediated deacetylation of the glucocorticoid receptor enables NF-kappaB suppression. The Journal of Experimental Medicine, 203(1), 7–13. https://doi.org/10.1084/jem.20050466
- Jawaid, A., Roszkowski, M., & Mansuy, I. M. (2018). Transgenerational epigenetics of traumatic stress. Progress in Molecular Biology and Translational Science, 158, 273–298. https://doi.org/10.1016/bs.pmbts.2018.03.003
- Jeanneteau, F., Barrère, C., Vos, M., De Vries, C. J. M., Rouillard, C., Levesque, D., Dromard, Y., Moisan, M.-P., Duric, V., Franklin, T. C., Duman, R. S., Lewis, D. A., Ginsberg, S. D., & Arango-Lievano, M. (2018). The stress-induced transcription factor NR4A1 adjusts mitochondrial function and synapse number in prefrontal cortex. The Journal of Neuroscience : The Official Journal of the Society for Neuroscience, 38(6), 1335–1350. https://doi.org/10.1523/JNEUROSCI.2793-17.2017
- Jeanneteau, F., Borie, A., Chao, M., & Garabedian, M. (2019). Bridging the gap between BDNF and glucocorticoid effects on brain networks. Neuroendocrinology, 109(3), 277–284. https://doi.org/10.1159/000496392
- Jeanneteau, F., & Chao, M. V. (2013). Are BDNF and glucocorticoid activities calibrated? Neuroscience, 239, 173–195. https://doi.org/10.1016/j.neuroscience.2012.09.017
- Jeanneteau, F., & Deinhardt, K. (2011). Fine-tuning MAPK signaling in the brain: The role of MKP-1. Communicative and Integrative Biology, 4, 1–3.
- Jeanneteau, F., Lambert, W. M., Ismaili, N., Bath, K. G., Lee, F. S., Garabedian, M. J., & Chao, M. V. (2012). BDNF and glucocorticoids regulate corticotrophin-releasing hormone (CRH) homeostasis in the hypothalamus . Proceedings of the National Academy of Sciences of the United States of America, 109(4), 1305–1310. https://doi.org/10.1073/pnas.1114122109
- Joels, M. (2008). Functional actions of corticosteroids in the hippocampus. European Journal of Pharmacology, 583, 312–321.
- Joels, M., & Baram, T. Z. (2009). The neuro-symphony of stress. Nature Reviews. Neuroscience, 10(6), 459–466. https://doi.org/10.1038/nrn2632
- Joels, M., & de Kloet, E. R. (1990). Mineralocorticoid receptor-mediated changes in membrane properties of rat CA1 pyramidal neurons in vitro. Proceedings of the National Academy of Sciences of the United States of America, 87(12), 4495–4498. https://doi.org/10.1073/pnas.87.12.4495
- Joels, M., & de Kloet, E. R. (1994). Mineralocorticoid and glucocorticoid receptors in the brain. Implications for ion permeability and transmitter systems. Progress in Neurobiology, 43, 1–36.
- Joels, M., Pasricha, N., & Karst, H. (2013). The interplay between rapid and slow corticosteroid actions in brain. European Journal of Pharmacology, 719(1-3), 44–52. https://doi.org/10.1016/j.ejphar.2013.07.015
- Joels, M., Pu, Z., Wiegert, O., Oitzl, M. S., & Krugers, H. J. (2006). Learning under stress: How does it work? Trends in Cognitive Sciences, 10(4), 152–158. https://doi.org/10.1016/j.tics.2006.02.002
- John, S., Sabo, P. J., Thurman, R. E., Sung, M. H., Biddie, S. C., Johnson, T. A., Hager, G. L., & Stamatoyannopoulos, J. A. (2011). Chromatin accessibility pre-determines glucocorticoid receptor binding patterns. Nature Genetics, 43(3), 264–268. https://doi.org/10.1038/ng.759
- Johnson, L. R., Farb, C., Morrison, J. H., McEwen, B. S., & LeDoux, J. E. (2005). Localization of glucocorticoid receptors at postsynaptic membranes in the lateral amygdala. Neuroscience, 136(1), 289–299. https://doi.org/10.1016/j.neuroscience.2005.06.050
- Kalafatakis, K., Russell, G. M., Zarros, A., & Lightman, S. L. (2016). Temporal control of glucocorticoid neurodynamics and its relevance for brain homeostasis, neuropathology and glucocorticoid-based therapeutics. Neuroscience and Biobehavioral Reviews, 61, 12–25. https://doi.org/10.1016/j.neubiorev.2015.11.009
- Kan, M., Shumyatcher, M., Diwadkar, A., Soliman, G., & Himes, B. E. (2018). Integration of transcriptomic data identifies global and cell-specific asthma-related gene expression signatures. AMIA. Annual Symposium Proceedings. AMIA Symposium, 2018, 1338–1347.
- Kanatsou, S., Joels, M., & Krugers, H. (2019). Brain mineralocorticoid receptors and resilience to stress. Vitamins and Hormones, 109, 341–359. https://doi.org/10.1016/bs.vh.2018.11.001
- Karatsoreos, I. N., & McEwen, B. S. (2011). Psychobiological allostasis: Resistance, resilience and vulnerability. Trends in Cognitive Sciences, 15(12), 576–584. https://doi.org/10.1016/j.tics.2011.10.005
- Karssen, A. M., Meijer, O. C., Berry, A., Sanjuan Pinol, R., & de Kloet, E. R. (2005). Low doses of dexamethasone can produce a hypocorticosteroid state in the brain. Endocrinology, 146(12), 5587–5595. https://doi.org/10.1210/en.2005-0501
- Karst, H., Berger, S., Erdmann, G., Schutz, G., & Joels, M. (2010). Metaplasticity of amygdalar responses to the stress hormone corticosterone. Proceedings of the National Academy of Sciences of the United States of America, 107(32), 14449–14454. https://doi.org/10.1073/pnas.0914381107
- Karst, H., de Kloet, E. R., & Joels, M. (1999). Episodic corticosterone treatment accelerates kindling epileptogenesis and triggers long-term changes in hippocampal CA1 cells, in the fully kindled state. The European Journal of Neuroscience, 11(3), 889–898. https://doi.org/10.1046/j.1460-9568.1999.00495.x
- Kellendonk, C., Gass, P., Kretz, O., Schutz, G., & Tronche, F. (2002). Corticosteroid receptors in the brain: Gene targeting studies. Brain Research Bulletin, 57(1), 73–83. https://doi.org/10.1016/S0361-9230(01)00638-4
- Kent, M., Bardi, M., Hazelgrove, A., Sewell, K., Kirk, E., Thompson, B., Trexler, K., Terhune-Cotter, B., & Lambert, K. (2017). Profiling coping strategies in male and female rats: Potential neurobehavioral markers of increased resilience to depressive symptoms. Hormones and Behavior, 95, 33–43. https://doi.org/10.1016/j.yhbeh.2017.07.011
- Khan, S. H., McLaughlin, W. A., & Kumar, R. (2017). Site-specific phosphorylation regulates the structure and function of an intrinsically disordered domain of the glucocorticoid receptor. Scientific Reports, 7(1), 15440. https://doi.org/10.1038/s41598-017-15549-5
- Kitagawa, H., Sugo, N., Morimatsu, M., Arai, Y., Yanagida, T., & Yamamoto, N. (2017). Activity-dependent dynamics of the transcription factor of cAMP-response element binding protein in cortical neurons revealed by single-molecule imaging. The Journal of Neuroscience : The Official Journal of the Society for Neuroscience, 37(1), 1–10. https://doi.org/10.1523/JNEUROSCI.0943-16.2016
- Klein, L. C., Popke, E. J., & Grunberg, N. E. (1998). Sex differences in effects of opioid blockade on stress-induced freezing behavior. Pharmacology, Biochemistry, and Behavior, 61(4), 413–417. https://doi.org/10.1016/S0091-3057(98)00135-X
- Klemm, S., Shipony, Z., & Greenleaf, W. (2019). Chromatin accessibility and the regulatory epigenome. Nature Reviews. Genetics, 20(4), 207–220. https://doi.org/10.1038/s41576-018-0089-8
- Klok, M. D., Alt, S. R., Irurzun Lafitte, A. J., Turner, J. D., Lakke, E. A., Huitinga, I., Muller, C. P., Zitman, F. G., de Kloet, E. R., & Derijk, R. H. (2011a). Decreased expression of mineralocorticoid receptor mRNA and its splice variants in postmortem brain regions of patients with major depressive disorder. Journal of Psychiatric Research, 45(7), 871–878. https://doi.org/10.1016/j.jpsychires.2010.12.002
- Klok, M. D., Giltay, E. J., Van der Does, A. J. W., Geleijnse, J. M., Antypa, N., Penninx, B. W. J. H., de Geus, E. J. C., Willemsen, G., Boomsma, D. I., van Leeuwen, N., Zitman, F. G., de Kloet, E. R., & DeRijk, R. H. (2011b). A common and functional mineralocorticoid receptor haplotype enhances optimism and protects against depression in females. Translational Psychiatry, 1, e62. https://doi.org/10.1038/tp.2011.59
- Knapman, A., Heinzmann, J. M., Hellweg, R., Holsboer, F., Landgraf, R., & Touma, C. (2010). Increased stress reactivity is associated with cognitive deficits and decreased hippocampal brain-derived neurotrophic factor in a mouse model of affective disorders. Journal of Psychiatric Research, 44(9), 566–575. https://doi.org/10.1016/j.jpsychires.2009.11.014
- Koning, A., Buurstede, J. C., van Weert, L., & Meijer, O. C. (2019). Glucocorticoid and mineralocorticoid receptors in the brain: A transcriptional perspective. Journal of the Endocrine Society, 3(10), 1917–1930. https://doi.org/10.1210/js.2019-00158
- Koob, G. F., & Le Moal, M. (2001). Drug addiction, dysregulation of reward, and allostasis. Neuropsychopharmacology : Official Publication of the American College of Neuropsychopharmacology, 24(2), 97–129. https://doi.org/10.1016/S0893-133X(00)00195-0
- Koolhaas, J. M., de Boer, S. F., Buwalda, B., & Meerlo, P. (2017). Social stress models in rodents: Towards enhanced validity. Neurobiology of Stress, 6, 104–112. https://doi.org/10.1016/j.ynstr.2016.09.003
- Krishnan, V., Han, M.-H., Graham, D. L., Berton, O., Renthal, W., Russo, S. J., Laplant, Q., Graham, A., Lutter, M., Lagace, D. C., Ghose, S., Reister, R., Tannous, P., Green, T. A., Neve, R. L., Chakravarty, S., Kumar, A., Eisch, A. J., Self, D. W., … Nestler, E. J. (2007). Molecular adaptations underlying susceptibility and resistance to social defeat in brain reward regions. Cell, 131(2), 391–404. https://doi.org/10.1016/j.cell.2007.09.018
- Kudielka, B. M., & Kirschbaum, C. (2005). Sex differences in HPA axis responses to stress: A review. Biological Psychology, 69(1), 113–132. https://doi.org/10.1016/j.biopsycho.2004.11.009
- Labonté, B., Engmann, O., Purushothaman, I., Menard, C., Wang, J., Tan, C., Scarpa, J. R., Moy, G., Loh, Y.-H E., Cahill, M., Lorsch, Z. S., Hamilton, P. J., Calipari, E. S., Hodes, G. E., Issler, O., Kronman, H., Pfau, M., Obradovic, A. L. J., Dong, Y., … Nestler, E. J. (2017). Sex-specific transcriptional signatures in human depression. Nature Medicine, 23(9), 1102–1111. https://doi.org/10.1038/nm.4386
- Lambert, W. M., Xu, C.-F., Neubert, T. A., Chao, M. V., Garabedian, M. J., & Jeanneteau, F. (2013). Brain-derived neurotrophic factor signaling rewrites the glucocorticoid transcriptome via glucocorticoid receptor phosphorylation . Molecular and Cellular Biology, 33(18), 3700–3714. https://doi.org/10.1128/MCB.00150-13
- Lapp, H. E., Bartlett, A. A., & Hunter, R. G. (2019). Stress and glucocorticoid receptor regulation of mitochondrial gene expression. Journal of Molecular Endocrinology, 62(2), R121–R128. https://doi.org/10.1530/JME-18-0152
- Laryea, G., Schutz, G., & Muglia, L. J. (2013). Disrupting hypothalamic glucocorticoid receptors causes HPA axis hyperactivity and excess adiposity. Molecular Endocrinology (Baltimore, Md.), 27(10), 1655–1665. https://doi.org/10.1210/me.2013-1187
- Lazarus, R., & Folkman, S. (1984). Stress, Appraisal, and Coping. Springer.
- Lee, M.-S., Kim, Y.-H., Park, W.-S., Park, O.-K., Kwon, S.-H., Hong, K. S., Rhim, H., Shim, I., Morita, K., Wong, D. L., Patel, P. D., Lyons, D. M., Schatzberg, A. F., & Her, S. (2016). Temporal variability of glucocorticoid receptor activity is functionally important for the therapeutic action of fluoxetine in the hippocampus. Molecular Psychiatry, 21(2), 252–260. https://doi.org/10.1038/mp.2014.137
- Li, Y., Suino, K., Daugherty, J., & Xu, H. E. (2005). Structural and biochemical mechanisms for the specificity of hormone binding and coactivator assembly by mineralocorticoid receptor. Molecular Cell, 19(3), 367–380. https://doi.org/10.1016/j.molcel.2005.06.026
- Liberman, A. C., Antunica-Noguerol, M., & Arzt, E. (2014). Modulation of the glucocorticoid receptor activity by post-translational modifications. Nuclear Receptor Research, 1, 1–15. https://doi.org/10.11131/2014/101086
- Liston, C., Cichon, J. M., Jeanneteau, F., Jia, Z., Chao, M. V., & Gan, W.-B. (2013). Circadian glucocorticoid oscillations promote learning-dependent synapse formation and maintenance. Nature Neuroscience, 16(6), 698–705. https://doi.org/10.1038/nn.3387
- Liston, C., & Gan, W. B. (2011). Glucocorticoids are critical regulators of dendritic spine development and plasticity in vivo. Proceedings of the National Academy of Sciences of the United States of America, 108(38), 16074–16079. https://doi.org/10.1073/pnas.1110444108
- Liu, D., Diorio, J., Tannenbaum, B., Caldji, C., Francis, D., Freedman, A., Sharma, S., Pearson, D., Plotsky, P. M., & Meaney, M. J. (1997). Maternal care, hippocampal glucocorticoid receptors, and hypothalamic-pituitary-adrenal responses to stress. Science (New York, N.Y.), 277(5332), 1659–1662. https://doi.org/10.1126/science.277.5332.1659
- Liu, W., Wang, J., Sauter, N. K., & Pearce, D. (1995). Steroid receptor heterodimerization demonstrated in vitro and in vivo. Proceedings of the National Academy of Sciences of the United States of America, 92(26), 12480–12484. https://doi.org/10.1073/pnas.92.26.12480
- Liu, W., Xue, X., Xia, J., Liu, J., & Qi, Z. (2018). Swimming exercise reverses CUMS-induced changes in depression-like behaviors and hippocampal plasticity-related proteins. Journal of Affective Disorders, 227, 126–135. https://doi.org/10.1016/j.jad.2017.10.019
- Lu, Y., Ji, Y., Ganesan, S., Schloesser, R., Martinowich, K., Sun, M., Mei, F., Chao, M. V., & Lu, B. (2011). TrkB as a potential synaptic and behavioral tag. The Journal of Neuroscience : The Official Journal of the Society for Neuroscience, 31(33), 11762–11771. https://doi.org/10.1523/JNEUROSCI.2707-11.2011
- Mahfouz, A., Lelieveldt, B. P., Grefhorst, A., van Weert, L. T., Mol, I. M., Sips, H. C., van den Heuvel, J. K., Datson, N. A., Visser, J. A., Reinders, M. J., & Meijer, O. C. (2016). Genome-wide coexpression of steroid receptors in the mouse brain: Identifying signaling pathways and functionally coordinated regions. Proceedings of the National Academy of Sciences of the United States of America, 113(10), 2738–2743. https://doi.org/10.1073/pnas.1520376113
- Mallei, A., Ieraci, A., & Popoli, M. (2019). Chronic social defeat stress differentially regulates the expression of BDNF transcripts and epigenetic modifying enzymes in susceptible and resilient mice. The World Journal of Biological Psychiatry : The Official Journal of the World Federation of Societies of Biological Psychiatry, 20(7), 555–566. https://doi.org/10.1080/15622975.2018.1500029
- Mayanagi, T., Morita, T., Hayashi, K., Fukumoto, K., & Sobue, K. (2008). Glucocorticoid receptor-mediated expression of caldesmon regulates cell migration via the reorganization of the actin cytoskeleton. The Journal of Biological Chemistry, 283(45), 31183–31196. https://doi.org/10.1074/jbc.M801606200
- McEwen, B. S. (1998). Stress, adaptation, and disease. Allostasis and allostatic load. Annals of the New York Academy of Sciences, 840, 33–44. https://doi.org/10.1111/j.1749-6632.1998.tb09546.x
- McEwen, B. S. (2006). Protective and damaging effects of stress mediators: Central role of the brain. Dialogues in Clinical Neuroscience, 8(4), 367–381.
- McEwen, B. S. (2007). Physiology and neurobiology of stress and adaptation: Central role of the brain. Physiological Reviews, 87(3), 873–904. https://doi.org/10.1152/physrev.00041.2006
- McEwen, B. S. (2015). Preserving neuroplasticity: Role of glucocorticoids and neurotrophins via phosphorylation. Proceedings of the National Academy of Sciences of the United States of America, 112(51), 15544–15545. https://doi.org/10.1073/pnas.1521416112
- McEwen, B. S., Bowles, N. P., Gray, J. D., Hill, M. N., Hunter, R. G., Karatsoreos, I. N., & Nasca, C. (2015). Mechanisms of stress in the brain. Nature Neuroscience, 18(10), 1353–1363. https://doi.org/10.1038/nn.4086
- McEwen, B. S., Nasca, C., & Gray, J. D. (2016). Stress effects on neuronal structure: Hippocampus, amygdala, and prefrontal cortex. Neuropsychopharmacology : Official Publication of the American College of Neuropsychopharmacology, 41(1), 3–23. https://doi.org/10.1038/npp.2015.171
- McGowan, P. O., Sasaki, A., D'Alessio, A. C., Dymov, S., Labonte, B., Szyf, M., Turecki, G., & Meaney, M. J. (2009). Epigenetic regulation of the glucocorticoid receptor in human brain associates with childhood abuse. Nature Neuroscience, 12(3), 342–348. https://doi.org/10.1038/nn.2270
- McIlwrick, S., Pohl, T., Chen, A., & Touma, C. (2017). Late-onset cognitive impairments after early-life stress are shaped by inherited differences in stress reactivity. Frontiers in Cellular Neuroscience, 11, 9. https://doi.org/10.3389/fncel.2017.00009
- McIlwrick, S., Rechenberg, A., Matthes, M., Burgstaller, J., Schwarzbauer, T., Chen, A., & Touma, C. (2016). Genetic predisposition for high stress reactivity amplifies effects of early-life adversity. Psychoneuroendocrinology, 70, 85–97. https://doi.org/10.1016/j.psyneuen.2016.04.023
- Meijer, O. C., Buurstede, J. C., & Schaaf, M. J. M. (2019). Corticosteroid receptors in the brain: Transcriptional mechanisms for specificity and context-dependent effects. Cellular and molecular neurobiology, 39(4), 539–549. https://doi.org/10.1007/s10571-018-0625-2
- Meijer, O. C., Kalkhoven, E., van der Laan, S., Steenbergen, P. J., Houtman, S. H., Dijkmans, T. F., Pearce, D., & de Kloet, E. R. (2005). Steroid receptor coactivator-1 splice variants differentially affect corticosteroid receptor signaling. Endocrinology, 146(3), 1438–1448. https://doi.org/10.1210/en.2004-0411
- Meijsing, S. H., Pufall, M. A., So, A. Y., Bates, D. L., Chen, L., & Yamamoto, K. R. (2009). DNA binding site sequence directs glucocorticoid receptor structure and activity. Science (New York, N.Y.), 324(5925), 407–410. https://doi.org/10.1126/science.1164265
- Menke, A., Arloth, J., Pütz, B., Weber, P., Klengel, T., Mehta, D., Gonik, M., Rex-Haffner, M., Rubel, J., Uhr, M., Lucae, S., Deussing, J. M., Müller-Myhsok, B., Holsboer, F., & Binder, E. B. (2012). Dexamethasone stimulated gene expression in peripheral blood is a sensitive marker for glucocorticoid receptor resistance in depressed patients. Neuropsychopharmacology : Official Publication of the American College of Neuropsychopharmacology, 37(6), 1455–1464. https://doi.org/10.1038/npp.2011.331
- Mifsud, K. R., & Reul, J. (2018). Mineralocorticoid and glucocorticoid receptor-mediated control of genomic responses to stress in the brain. Stress (Amsterdam, Netherlands), 21(5), 389–402. https://doi.org/10.1080/10253890.2018.1456526
- Mifsud, K. R., & Reul, J. M. (2016). Acute stress enhances heterodimerization and binding of corticosteroid receptors at glucocorticoid target genes in the hippocampus. Proceedings of the National Academy of Sciences of the United States of America, 113(40), 11336–11341. https://doi.org/10.1073/pnas.1605246113
- Mitic, M., Simic, I., Djordjevic, J., Radojcic, M. B., & Adzic, M. (2013). Gender-specific effects of fluoxetine on hippocampal glucocorticoid receptor phosphorylation and behavior in chronically stressed rats. Neuropharmacology, 70, 100–111. https://doi.org/10.1016/j.neuropharm.2012.12.012
- Mitre, M., Mariga, A., & Chao, M. V. (2017). Neurotrophin signalling: Novel insights into mechanisms and pathophysiology. Clinical Science (London, England : 1979), 131(1), 13–23. https://doi.org/10.1042/CS20160044
- Moda-Sava, R. N., Murdock, M. H., Parekh, P. K., Fetcho, R. N., Huang, B. S., Huynh, T. N., Witztum, J., Shaver, D. C., Rosenthal, D. L., & Alway, E. J. (2019). Sustained rescue of prefrontal circuit dysfunction by antidepressant-induced spine formation. Science, 364(6436), eaat8078.
- Monczor, F., Chatzopoulou, A., Zappia, C. D., Houtman, R., Meijer, O. C., & Fitzsimons, C. P. (2019). A model of glucocorticoid receptor interaction with coregulators predicts transcriptional regulation of target genes. Frontiers in Pharmacology, 10, 214.
- Morsink, M. C., Steenbergen, P. J., Vos, J. B., Karst, H., Joels, M., De Kloet, E. R., & Datson, N. A. (2006). Acute activation of hippocampal glucocorticoid receptors results in different waves of gene expression throughout time. Journal of Neuroendocrinology, 18(4), 239–252. https://doi.org/10.1111/j.1365-2826.2006.01413.x
- Mukherjee, D., Ignatowska-Jankowska, B. M., Itskovits, E., Gonzales, B. J., Turm, H., Izakson, L., Haritan, D., Bleistein, N., Cohen, C., Amit, I., Shay, T., Grueter, B., Zaslaver, A., & Citri, A. (2018). Salient experiences are represented by unique transcriptional signatures in the mouse brain. eLife, 7, e31220. https://doi.org/10.7554/eLife.31220
- Muto, A., Taylor, M. R., Suzawa, M., Korenbrot, J. I., & Baier, H. (2013). Glucocorticoid receptor activity regulates light adaptation in the zebrafish retina. Frontiers in Neural Circuits, 7, 145. https://doi.org/10.3389/fncir.2013.00145
- Myers, B., McKlveen, J. M., & Herman, J. P. (2014). Glucocorticoid actions on synapses, circuits, and behavior: Implications for the energetics of stress. Frontiers in Neuroendocrinology, 35(2), 180–196. https://doi.org/10.1016/j.yfrne.2013.12.003
- Nasca, C., Zelli, D., Bigio, B., Piccinin, S., Scaccianoce, S., Nistico, R., & McEwen, B. S. (2015). Stress dynamically regulates behavior and glutamatergic gene expression in hippocampus by opening a window of epigenetic plasticity. Proceedings of the National Academy of Sciences of the United States of America, 112(48), 14960–14965. https://doi.org/10.1073/pnas.1516016112
- Nasrallah, P., Haidar, E. A., Stephan, J. S., El Hayek, L., Karnib, N., Khalifeh, M., Barmo, N., Jabre, V., Houbeika, R., Ghanem, A., Nasser, J., Zeeni, N., Bassil, M., & Sleiman, S. F. (2019). Branched-chain amino acids mediate resilience to chronic social defeat stress by activating BDNF/TRKB signaling. Neurobiology of Stress, 11, 100170. https://doi.org/10.1016/j.ynstr.2019.100170
- Nestler, E. J. (2016). Transgenerational epigenetic contributions to stress responses: Fact or fiction? PLoS Biology, 14(3), e1002426. https://doi.org/10.1371/journal.pbio.1002426
- Ng, L. H. L., Huang, Y., Han, L., Chang, R. C., Chan, Y. S., & Lai, C. S. W. (2018). Ketamine and selective activation of parvalbumin interneurons inhibit stress-induced dendritic spine elimination. Translational Psychiatry, 8(1), 272. https://doi.org/10.1038/s41398-018-0321-5
- Notaras, M., Du, X., Gogos, J., van den Buuse, M., & Hill, R. A. (2017). The BDNF Val66Met polymorphism regulates glucocorticoid-induced corticohippocampal remodeling and behavioral despair. Translational Psychiatry, 7(9), e1233. https://doi.org/10.1038/tp.2017.205
- Notaras, M., Hill, R., Gogos, J. A., & van den Buuse, M. (2016). BDNF Val66Met genotype determines hippocampus-dependent behavior via sensitivity to glucocorticoid signaling. Molecular Psychiatry, 21(6), 730–732. https://doi.org/10.1038/mp.2015.152
- Nott, A., Watson, P. M., Robinson, J. D., Crepaldi, L., & Riccio, A. (2008). S-Nitrosylation of histone deacetylase 2 induces chromatin remodelling in neurons. Nature, 455(7211), 411–415. https://doi.org/10.1038/nature07238
- Numakawa, T., Kumamaru, E., Adachi, N., Yagasaki, Y., Izumi, A., & Kunugi, H. (2009). Glucocorticoid receptor interaction with TrkB promotes BDNF-triggered PLC-gamma signaling for glutamate release via a glutamate transporter. Proceedings of the National Academy of Sciences of the United States of America, 106(2), 647–652. https://doi.org/10.1073/pnas.0800888106
- Paakinaho, V., Johnson, T. A., Presman, D. M., & Hager, G. L. (2019). Glucocorticoid receptor quaternary structure drives chromatin occupancy and transcriptional outcome. Genome Research, 29(8), 1223–1234. https://doi.org/10.1101/gr.244814.118
- Parnaudeau, S., Dongelmans, M. L., Turiault, M., Ambroggi, F., Delbes, A. S., Cansell, C., Luquet, S., Piazza, P. V., Tronche, F., & Barik, J. (2014). Glucocorticoid receptor gene inactivation in dopamine-innervated areas selectively decreases behavioral responses to amphetamine. Frontiers in Behavioral Neuroscience, 8, 35. https://doi.org/10.3389/fnbeh.2014.00035
- Paugh, S. W., Bonten, E. J., Savic, D., Ramsey, L. B., Thierfelder, W. E., Gurung, P., Malireddi, R. K. S., Actis, M., Mayasundari, A., Min, J., Coss, D. R., Laudermilk, L. T., Panetta, J. C., McCorkle, J. R., Fan, Y., Crews, K. R., Stocco, G., Wilkinson, M. R., Ferreira, A. M., … Evans, W. E. (2015). NALP3 inflammasome upregulation and CASP1 cleavage of the glucocorticoid receptor cause glucocorticoid resistance in leukemia cells. Nature Genetics, 47(6), 607–614. https://doi.org/10.1038/ng.3283
- Pearce, D. (1994). A mechanistic basis for distinct mineralocorticoid and glucocorticoid receptor transcriptional specificities. Steroids, 59(2), 153–159. https://doi.org/10.1016/0039-128X(94)90094-9
- Peters, A., McEwen, B. S., & Friston, K. (2017). Uncertainty and stress: Why it causes diseases and how it is mastered by the brain. Progress in Neurobiology, 156, 164–188. https://doi.org/10.1016/j.pneurobio.2017.05.004
- Petta, I., Dejager, L., Ballegeer, M., Lievens, S., Tavernier, J., De Bosscher, K., & Libert, C. (2016). The interactome of the glucocorticoid receptor and its influence on the actions of glucocorticoids in combatting inflammatory and infectious diseases. Microbiology and Molecular Biology Reviews : MMBR, 80(2), 495–522. https://doi.org/10.1128/MMBR.00064-15
- Piazza, P. V., & Le Moal, M. L. (1996). Pathophysiological basis of vulnerability to drug abuse: Role of an interaction between stress, glucocorticoids, and dopaminergic neurons. Annual Review of Pharmacology and Toxicology, 36, 359–378. https://doi.org/10.1146/annurev.pa.36.040196.002043
- Picard, M., Juster, R. P., & McEwen, B. S. (2014). Mitochondrial allostatic load puts the ‘gluc’ back in glucocorticoids. Nature Reviews. Endocrinology, 10(5), 303–310. https://doi.org/10.1038/nrendo.2014.22
- Pittenger, C., & Duman, R. S. (2008). Stress, depression, and neuroplasticity: A convergence of mechanisms. Neuropsychopharmacology : Official Publication of the American College of Neuropsychopharmacology, 33(1), 88–109. https://doi.org/10.1038/sj.npp.1301574
- Polman, J. A., de Kloet, E. R., & Datson, N. A. (2013). Two populations of glucocorticoid receptor-binding sites in the male rat hippocampal genome. Endocrinology, 154(5), 1832–1844. https://doi.org/10.1210/en.2012-2187
- Pooley, J. R., Flynn, B. P., Grøntved, L., Baek, S., Guertin, M. J., Kershaw, Y. M., Birnie, M. T., Pellatt, A., Rivers, C. A., Schiltz, R. L., Hager, G. L., Lightman, S. L., & Conway-Campbell, B. L. (2017). Genome-wide identification of basic helix-loop-helix and NF-1 motifs underlying GR binding sites in male rat hippocampus. Endocrinology, 158(5), 1486–1501. https://doi.org/10.1210/en.2016-1929
- Pooley, J. R., Rivers, C. A., Kilcooley, M. T., Paul, S. N., Cavga, A. D., Kershaw, Y. M., Muratcioglu, S., Gursoy, A., Keskin, O., & Lightman, S. L. (2020). Beyond the heterodimer model for mineralocorticoid and glucocorticoid receptor interactions in nuclei and at DNA. PLoS One, 15(1), e0227520. https://doi.org/10.1371/journal.pone.0227520
- Poolman, T. M., Farrow, S. N., Matthews, L., Loudon, A. S., & Ray, D. W. (2013). Pin1 promotes GR transactivation by enhancing recruitment to target genes. Nucleic Acids Research, 41(18), 8515–8525. https://doi.org/10.1093/nar/gkt624
- Prager, E. M., Brielmaier, J., Bergstrom, H. C., McGuire, J., & Johnson, L. R. (2010). Localization of mineralocorticoid receptors at mammalian synapses. PLoS One, 5(12), e14344. https://doi.org/10.1371/journal.pone.0014344
- Provencal, N., Arloth, J., Cattaneo, A., Anacker, C., Cattane, N., Wiechmann, T., Roh, S., Kodel, M., Klengel, T., & Czamara, D. (2019). Glucocorticoid exposure during hippocampal neurogenesis primes future stress response by inducing changes in DNA methylation. Proceedings of the National Academy of Sciences of the United States of America. https://doi.org/10.1073/pnas.1820842116
- Quinn, D. P., Kolar, A., Harris, S. A., Wigerius, M., Fawcett, J. P., & Krueger, S. R. (2019). The stability of glutamatergic synapses is independent of activity level, but predicted by synapse size. Frontiers in Cellular Neuroscience, 13, 291. https://doi.org/10.3389/fncel.2019.00291
- Ramamoorthy, S., & Cidlowski, J. A. (2013). Exploring the molecular mechanisms of glucocorticoid receptor action from sensitivity to resistance. Endocrine Development, 24, 41–56. https://doi.org/10.1159/000342502
- Rao-Ruiz, P., Couey, J. J., Marcelo, I. M., Bouwkamp, C. G., Slump, D. E., Matos, M. R., van der Loo, R. J., Martins, G. J., van den Hout, M., van IJcken, W. F., Costa, R. M., van den Oever, M. C., & Kushner, S. A. (2019). Engram-specific transcriptome profiling of contextual memory consolidation. Nature Communications, 10(1), 2232. https://doi.org/10.1038/s41467-019-09960-x
- Rapicavoli, N. A., Qu, K., Zhang, J., Mikhail, M., Laberge, R. M., & Chang, H. Y. (2013). A mammalian pseudogene lncRNA at the interface of inflammation and anti-inflammatory therapeutics. eLife, 2, e00762. https://doi.org/10.7554/eLife.00762
- Ratajczak, T., Cluning, C., & Ward, B. K. (2015). Steroid receptor-associated immunophilins: A gateway to steroid signalling. The Clinical Biochemist. Reviews, 36(2), 31–52.
- Ratman, D., Vanden Berghe, W., Dejager, L., Libert, C., Tavernier, J., Beck, I. M., & De Bosscher, K. (2013). How glucocorticoid receptors modulate the activity of other transcription factors: A scope beyond tethering. Molecular and Cellular Endocrinology, 380(1-2), 41–54. https://doi.org/10.1016/j.mce.2012.12.014
- Rattiner, L. M., Davis, M., French, C. T., & Ressler, K. J. (2004a). Brain-derived neurotrophic factor and tyrosine kinase receptor B involvement in amygdala-dependent fear conditioning. The Journal of Neuroscience : The Official Journal of the Society for Neuroscience, 24(20), 4796–4806. https://doi.org/10.1523/JNEUROSCI.5654-03.2004
- Rattiner, L. M., Davis, M., & Ressler, K. J. (2004b). Differential regulation of brain-derived neurotrophic factor transcripts during the consolidation of fear learning. Learning & Memory (Cold Spring Harbor, N.Y.), 11(6), 727–731. https://doi.org/10.1101/lm.83304
- Reul, J. M., & de Kloet, E. R. (1985). Two receptor systems for corticosterone in rat brain: Microdistribution and differential occupation. Endocrinology, 117(6), 2505–2511. https://doi.org/10.1210/endo-117-6-2505
- Revest, J. M., Le Roux, A., Roullot-Lacarriere, V., Kaouane, N., Vallee, M., Kasanetz, F., Rouge-Pont, F., Tronche, F., Desmedt, A., & Piazza, P. V. (2014). BDNF-TrkB signaling through Erk1/2 phosphorylation mediates the enhancement of fear memory induced by glucocorticoids. Molecular Psychiatry, 19(9), 1001–1009. https://doi.org/10.1038/mp.2013.134
- Rincon-Cortes, M., Herman, J. P., Lupien, S., Maguire, J., & Shansky, R. M. (2019). Stress: Influence of sex, reproductive status and gender. Neurobiology of Stress, 10, 100155. https://doi.org/10.1016/j.ynstr.2019.100155
- Rivers, C. A., Rogers, M. F., Stubbs, F. E., Conway-Campbell, B. L., Lightman, S. L., & Pooley, J. R. (2019). Glucocorticoid receptor-tethered mineralocorticoid receptors increase glucocorticoid-induced transcriptional responses. Endocrinology, 160(5), 1044–1056. https://doi.org/10.1210/en.2018-00819
- Rollins, D. A., Kharlyngdoh, J. B., Coppo, M., Tharmalingam, B., Mimouna, S., Guo, Z., Sacta, M. A., Pufall, M. A., Fisher, R. P., Hu, X., Chinenov, Y., & Rogatsky, I. (2017). Glucocorticoid-induced phosphorylation by CDK9 modulates the coactivator functions of transcriptional cofactor GRIP1 in macrophages. Nature Communications, 8(1), 1739. https://doi.org/10.1038/s41467-017-01569-2
- Rothman, S. M., & Mattson, M. P. (2013). Activity-dependent, stress-responsive BDNF signaling and the quest for optimal brain health and resilience throughout the lifespan. Neuroscience, 239, 228–240. https://doi.org/10.1016/j.neuroscience.2012.10.014
- Russell, G. M., Kalafatakis, K., & Lightman, S. L. (2015). The importance of biological oscillators for hypothalamic-pituitary-adrenal activity and tissue glucocorticoid response: Coordinating stress and neurobehavioural adaptation. Journal of Neuroendocrinology, 27(6), 378–388. https://doi.org/10.1111/jne.12247
- Russo, M. F., Ah Loy, S. R., Battle, A. R., & Johnson, L. R. (2016). Membrane associated synaptic mineralocorticoid and glucocorticoid receptors are rapid regulators of dendritic spines. Frontiers in Cellular Neuroscience, 10, 161. https://doi.org/10.3389/fncel.2016.00161
- Saaltink, D. J., & Vreugdenhil, E. (2014). Stress, glucocorticoid receptors, and adult neurogenesis: A balance between excitation and inhibition? Cellular and Molecular Life Sciences : CMLS, 71(13), 2499–2515. https://doi.org/10.1007/s00018-014-1568-5
- Sacta, M. A., Chinenov, Y., & Rogatsky, I. (2016). Glucocorticoid signaling: An update from a genomic perspective. Annual Review of Physiology, 78, 155–180. https://doi.org/10.1146/annurev-physiol-021115-105323
- Sarabdjitsingh, R. A., Conway-Campbell, B. L., Leggett, J. D., Waite, E. J., Meijer, O. C., de Kloet, E. R., & Lightman, S. L. (2010a). Stress responsiveness varies over the ultradian glucocorticoid cycle in a brain-region-specific manner. Endocrinology, 151(11), 5369–5379. https://doi.org/10.1210/en.2010-0832
- Sarabdjitsingh, R. A., Isenia, S., Polman, A., Mijalkovic, J., Lachize, S., Datson, N., de Kloet, E. R., & Meijer, O. C. (2010b). Disrupted corticosterone pulsatile patterns attenuate responsiveness to glucocorticoid signaling in rat brain. Endocrinology, 151(3), 1177–1186. https://doi.org/10.1210/en.2009-1119
- Sarabdjitsingh, R. A., Jezequel, J., Pasricha, N., Mikasova, L., Kerkhofs, A., Karst, H., Groc, L., & Joels, M. (2014). Ultradian corticosterone pulses balance glutamatergic transmission and synaptic plasticity. Proceedings of the National Academy of Sciences of the United States of America, 111(39), 14265–14270. https://doi.org/10.1073/pnas.1411216111
- Sarabdjitsingh, R. A., Spiga, F., Oitzl, M. S., Kershaw, Y., Meijer, O. C., Lightman, S. L., & de Kloet, E. R. (2010c). Recovery from disrupted ultradian glucocorticoid rhythmicity reveals a dissociation between hormonal and behavioural stress responsiveness. Journal of Neuroendocrinology, 22(8), 862–871. https://doi.org/10.1111/j.1365-2826.2010.02004.x
- Scheimann, J. R., Mahbod, P., Morano, R., Frantz, L., Packard, B., Campbell, K., & Herman, J. P. (2018). Deletion of glucocorticoid receptors in forebrain GABAergic neurons alters acute stress responding and passive avoidance behavior in female mice. Frontiers in Behavioral Neuroscience, 12, 325. https://doi.org/10.3389/fnbeh.2018.00325
- Scheimann, J. R., Moloney, R. D., Mahbod, P., Morano, R. L., Fitzgerald, M., Hoskins, O., Packard, B. A., Cotella, E. M., Hu, Y. C., & Herman, J. P. (2019). Conditional deletion of glucocorticoid receptors in rat brain results in sex-specific deficits in fear and coping behaviors. eLife, 8, e44672. https://doi.org/10.7554/eLife.44672
- Schiller, B. J., Chodankar, R., Watson, L. C., Stallcup, M. R., & Yamamoto, K. R. (2014). Glucocorticoid receptor binds half sites as a monomer and regulates specific target genes. Genome Biology, 15(7), 418. https://doi.org/10.1186/s13059-014-0418-y
- Schouten, M., Bielefeld, P., Garcia-Corzo, L., Passchier, E. M. J., Gradari, S., Jungenitz, T., Pons-Espinal, M., Gebara, E., Martín-Suárez, S., Lucassen, P. J., De Vries, H. E., Trejo, J. L., Schwarzacher, S. W., De Pietri Tonelli, D., Toni, N., Mira, H., Encinas, J. M., & Fitzsimons, C. P. (2020). Circadian glucocorticoid oscillations preserve a population of adult hippocampal neural stem cells in the aging brain. Molecular Psychiatry, 25(7), 1382–1405. https://doi.org/10.1038/s41380-019-0440-2
- Schwabe, L., Joels, M., Roozendaal, B., Wolf, O. T., & Oitzl, M. S. (2012). Stress effects on memory: An update and integration. Neurosci Biobehav Rev, 36(7), 1740–1749. https://doi.org/10.1016/j.neubiorev.2011.07.002
- Selye, H. (1956). The stress of life. McGrawHill.
- Shalev, I., Lerer, E., Israel, S., Uzefovsky, F., Gritsenko, I., Mankuta, D., Ebstein, R. P., & Kaitz, M. (2009). BDNF Val66Met polymorphism is associated with HPA axis reactivity to psychological stress characterized by genotype and gender interactions. Psychoneuroendocrinology, 34(3), 382–388. https://doi.org/10.1016/j.psyneuen.2008.09.017
- Simard, M., Couldwell, W. T., Zhang, W., Song, H., Liu, S., Cotrina, M. L., Goldman, S., & Nedergaard, M. (1999). Glucocorticoids-potent modulators of astrocytic calcium signaling. Glia, 28(1), 1–12. https://doi.org/10.1002/(SICI)1098-1136(199910)28:1<1::AID-GLIA1>3.0.CO;2-4
- Snyder-Mackler, N., Sanz, J., Kohn, J. N., Voyles, T., Pique-Regi, R., Wilson, M. E., Barreiro, L. B., & Tung, J. (2019). Social status alters chromatin accessibility and the gene regulatory response to glucocorticoid stimulation in rhesus macaques. Proceedings of the National Academy of Sciences of the United States of America, 116(4), 1219–1228. https://doi.org/10.1073/pnas.1811758115
- Soares, J. M., Sampaio, A., Ferreira, L. M., Santos, N. C., Marques, P., Marques, F., Palha, J. A., Cerqueira, J. J., & Sousa, N. (2013). Stress impact on resting state brain networks. PLoS One, 8(6), e66500. https://doi.org/10.1371/journal.pone.0066500
- Soliman, F., Glatt, C. E., Bath, K. G., Levita, L., Jones, R. M., Pattwell, S. S., Jing, D., Tottenham, N., Amso, D., Somerville, L. H., Voss, H. U., Glover, G., Ballon, D. J., Liston, C., Teslovich, T., Van Kempen, T., Lee, F. S., & Casey, B. J. (2010). A genetic variant BDNF polymorphism alters extinction learning in both mouse and human. Science (New York, N.Y.), 327(5967), 863–866. https://doi.org/10.1126/science.1181886
- Solomon, M. B., & Herman, J. P. (2009). Sex differences in psychopathology: Of gonads, adrenals and mental illness. Physiology & Behavior, 97(2), 250–258. https://doi.org/10.1016/j.physbeh.2009.02.033
- Stepanichev, M., Manolova, A., Peregud, D., Onufriev, M., Freiman, S., Aniol, V., Moiseeva, Y., Novikova, M., Lazareva, N., & Gulyaeva, N. (2018). Specific activity features in the forced swim test: Brain neurotrophins and development of stress-induced depressive-like behavior in rats. Neuroscience, 375, 49–61. https://doi.org/10.1016/j.neuroscience.2018.02.007
- Stournaras, C., Gravanis, A., Margioris, A. N., & Lang, F. (2014). The actin cytoskeleton in rapid steroid hormone actions. Cytoskeleton (Hoboken, N.J.), 71(5), 285–293. https://doi.org/10.1002/cm.21172
- Suderman, M., McGowan, P. O., Sasaki, A., Huang, T. C., Hallett, M. T., Meaney, M. J., Turecki, G., & Szyf, M. (2012). Conserved epigenetic sensitivity to early life experience in the rat and human hippocampus. Proceedings of the National Academy of Sciences of the United States of America, 109 Suppl 2, 17266–17272. https://doi.org/10.1073/pnas.1121260109
- Swanson, A. M., Shapiro, L. P., Whyte, A. J., & Gourley, S. L. (2013). Glucocorticoid receptor regulation of action selection and prefrontal cortical dendritic spines. Communicative & Integrative Biology, 6(6), e26068. https://doi.org/10.4161/cib.26068
- Taliaz, D., Loya, A., Gersner, R., Haramati, S., Chen, A., & Zangen, A. (2011). Resilience to chronic stress is mediated by hippocampal brain-derived neurotrophic factor. The Journal of Neuroscience : The Official Journal of the Society for Neuroscience, 31(12), 4475–4483. https://doi.org/10.1523/JNEUROSCI.5725-10.2011
- Tasker, J. G. (2006). Rapid glucocorticoid actions in the hypothalamus as a mechanism of homeostatic integration. Obesity (Silver Spring, Md.), 14 Suppl 5, 259S–265S. https://doi.org/10.1038/oby.2006.320
- Taylor, S. E., Klein, L. C., Lewis, B. P., Gruenewald, T. L., Gurung, R. A., & Updegraff, J. A. (2000). Biobehavioral responses to stress in females: Tend-and-befriend, not fight-or-flight. Psychological Review, 107(3), 411–429. https://doi.org/10.1037/0033-295x.107.3.411
- Tornese, P., Sala, N., Bonini, D., Bonifacino, T., La Via, L., Milanese, M., Treccani, G., Seguini, M., Ieraci, A., Mingardi, J., Nyengaard, J. R., Calza, S., Bonanno, G., Wegener, G., Barbon, A., Popoli, M., & Musazzi, L. (2019). Chronic mild stress induces anhedonic behavior and changes in glutamate release, BDNF trafficking and dendrite morphology only in stress vulnerable rats. The rapid restorative action of ketamine. Neurobiology of Stress, 10, 100160. https://doi.org/10.1016/j.ynstr.2019.100160
- Touma, C., Bunck, M., Glasl, L., Nussbaumer, M., Palme, R., Stein, H., Wolferstatter, M., Zeh, R., Zimbelmann, M., Holsboer, F., & Landgraf, R. (2008). Mice selected for high versus low stress reactivity: A new animal model for affective disorders. Psychoneuroendocrinology, 33(6), 839–862. https://doi.org/10.1016/j.psyneuen.2008.03.013
- Touma, C., Fenzl, T., Ruschel, J., Palme, R., Holsboer, F., Kimura, M., & Landgraf, R. (2009). Rhythmicity in mice selected for extremes in stress reactivity: Behavioural, endocrine and sleep changes resembling endophenotypes of major depression. PLoS One, 4(1), e4325. https://doi.org/10.1371/journal.pone.0004325
- Trapp, T., Rupprecht, R., Castren, M., Reul, J. M., & Holsboer, F. (1994). Heterodimerization between mineralocorticoid and glucocorticoid receptor: A new principle of glucocorticoid action in the CNS. Neuron, 13(6), 1457–1462. https://doi.org/10.1016/0896-6273(94)90431-6
- Tronche, F., Kellendonk, C., Kretz, O., Gass, P., Anlag, K., Orban, P. C., Bock, R., Klein, R., & Schutz, G. (1999). Disruption of the glucocorticoid receptor gene in the nervous system results in reduced anxiety. Nature Genetics, 23(1), 99–103. https://doi.org/10.1038/12703
- Ulrich-Lai, Y. M., & Herman, J. P. (2009). Neural regulation of endocrine and autonomic stress responses. Nature reviews. Neuroscience, 10(6), 397–409. https://doi.org/10.1038/nrn2647
- van der Laan, S., de Kloet, E. R., & Meijer, O. C. (2009). Timing is critical for effective glucocorticoid receptor mediated repression of the cAMP-induced CRH gene. PLoS One, 4(1), e4327. https://doi.org/10.1371/journal.pone.0004327
- van Weert, L., Buurstede, J. C., Mahfouz, A., Braakhuis, P. S. M., Polman, J. A. E., Sips, H. C. M., Roozendaal, B., Balog, J., de Kloet, E. R., Datson, N. A., & Meijer, O. C. (2017). NeuroD factors discriminate mineralocorticoid from glucocorticoid receptor DNA binding in the male rat brain. Endocrinology, 158(5), 1511–1522. https://doi.org/10.1210/en.2016-1422
- van Weert, L. T. C. M., Buurstede, J. C., Sips, H. C. M., Vettorazzi, S., Mol, I. M., Hartmann, J., Prekovic, S., Zwart, W., Schmidt, M. V., Roozendaal, B., Tuckermann, J. P., Sarabdjitsingh, R. A., & Meijer, O. C. (2019). Identification of mineralocorticoid receptor target genes in the mouse hippocampus. Journal of Neuroendocrinology, 31(8), e12735. https://doi.org/10.1111/jne.12735
- Vandevyver, S., Dejager, L., & Libert, C. (2014). Comprehensive overview of the structure and regulation of the glucocorticoid receptor. Endocrine Reviews, 35(4), 671–693. https://doi.org/10.1210/er.2014-1010
- Veenema, A. H., Koolhaas, J. M., & de Kloet, E. R. (2004). Basal and stress-induced differences in HPA axis, 5-HT responsiveness, and hippocampal cell proliferation in two mouse lines. Annals of the New York Academy of Sciences, 1018, 255–265. https://doi.org/10.1196/annals.1296.030
- Viho, E. M. G., Buurstede, J. C., Mahfouz, A., Koorneef, L. L., van Weert, L., Houtman, R., Hunt, H. J., Kroon, J., & Meijer, O. C. (2019). Corticosteroid action in the brain: The potential of selective receptor modulation. Neuroendocrinology, 109(3), 266–276. https://doi.org/10.1159/000499659
- Vinckier, F., Rigoux, L., Oudiette, D., & Pessiglione, M. (2018). Neuro-computational account of how mood fluctuations arise and affect decision making. Nature Communications, 9(1), 1708. https://doi.org/10.1038/s41467-018-03774-z
- Vinkers, C. H., Joels, M., Milaneschi, Y., Gerritsen, L., Kahn, R. S., Penninx, B. W., & Boks, M. P. (2015). Mineralocorticoid receptor haplotypes sex-dependently moderate depression susceptibility following childhood maltreatment. Psychoneuroendocrinology, 54, 90–102. https://doi.org/10.1016/j.psyneuen.2015.01.018
- Vockley, C. M., D'Ippolito, A. M., McDowell, I. C., Majoros, W. H., Safi, A., Song, L., Crawford, G. E., & Reddy, T. E. (2016). Direct GR binding sites potentiate clusters of TF binding across the human genome. Cell, 166(5), 1269–1281.e19. https://doi.org/10.1016/j.cell.2016.07.049
- Walker, S. E., Papilloud, A., Huzard, D., & Sandi, C. (2018). The link between aberrant hypothalamic-pituitary-adrenal axis activity during development and the emergence of aggression-Animal studies. Neuroscience and Biobehavioral Reviews, 91, 138–152. https://doi.org/10.1016/j.neubiorev.2016.10.008
- Walker, S. E., Zanoletti, O., Guillot de Suduiraut, I., & Sandi, C. (2017). Constitutive differences in glucocorticoid responsiveness to stress are related to variation in aggression and anxiety-related behaviors. Psychoneuroendocrinology, 84, 1–10. https://doi.org/10.1016/j.psyneuen.2017.06.011
- Wamsteeker Cusulin, J. I., Fuzesi, T., Inoue, W., & Bains, J. S. (2013). Glucocorticoid feedback uncovers retrograde opioid signaling at hypothalamic synapses. Nature Neuroscience, 16(5), 596–604. https://doi.org/10.1038/nn.3374
- Watson, L. C., Kuchenbecker, K. M., Schiller, B. J., Gross, J. D., Pufall, M. A., & Yamamoto, K. R. (2013). The glucocorticoid receptor dimer interface allosterically transmits sequence-specific DNA signals. Nature Structural & Molecular Biology, 20(7), 876–883. https://doi.org/10.1038/nsmb.2595
- Weikum, E. R., Knuesel, M. T., Ortlund, E. A., & Yamamoto, K. R. (2017). Glucocorticoid receptor control of transcription: Precision and plasticity via allostery. Nature Reviews. Molecular Cell Biology, 18(3), 159–174. https://doi.org/10.1038/nrm.2016.152
- Whirledge, S., & DeFranco, D. B. (2018). Glucocorticoid signaling in health and disease: Insights from tissue-specific GR knockout mice. Endocrinology, 159(1), 46–64. https://doi.org/10.1210/en.2017-00728
- Wild, A. R., Sinnen, B. L., Dittmer, P. J., Kennedy, M. J., Sather, W. A., & Dell'Acqua, M. L. (2019). Synapse-to-nucleus communication through NFAT is mediated by L-type Ca2+ channel Ca2+ spike propagation to the soma. Cell Reports, 26(13), 3537–3550.e4. https://doi.org/10.1016/j.celrep.2019.03.005
- Wochnik, G. M., Ruegg, J., Abel, G. A., Schmidt, U., Holsboer, F., & Rein, T. (2005). FK506-binding proteins 51 and 52 differentially regulate dynein interaction and nuclear translocation of the glucocorticoid receptor in mammalian cells. The Journal of Biological Chemistry, 280(6), 4609–4616. https://doi.org/10.1074/jbc.M407498200
- Wood, S. K., & Bhatnagar, S. (2015). Resilience to the effects of social stress: Evidence from clinical and preclinical studies on the role of coping strategies. Neurobiology of Stress, 1, 164–173. https://doi.org/10.1016/j.ynstr.2014.11.002
- Wook Koo, J., Labonte, B., Engmann, O., Calipari, E. S., Juarez, B., Lorsch, Z., Walsh, J. J., Friedman, A. K., Yorgason, J. T., Han, M. H., & Nestler, E. J. (2016). Essential role of mesolimbic brain-derived neurotrophic factor in chronic social stress-induced depressive behaviors. Biological Psychiatry, 80(6), 469–478. https://doi.org/10.1016/j.biopsych.2015.12.009
- Yamashita, N., Joshi, R., Zhang, S., Zhang, Z. Y., & Kuruvilla, R. (2017). Phospho-regulation of soma-to-axon transcytosis of neurotrophin receptors. Developmental Cell, 42(6), 626–639 e625. https://doi.org/10.1016/j.devcel.2017.08.009
- Yang, Y., Yamada, T., Hill, K. K., Hemberg, M., Reddy, N. C., Cho, H. Y., Guthrie, A. N., Oldenborg, A., Heiney, S. A., Ohmae, S., Medina, J. F., Holy, T. E., & Bonni, A. (2016). Chromatin remodeling inactivates activity genes and regulates neural coding. Science (New York, N.Y.), 353(6296), 300–305. https://doi.org/10.1126/science.aad4225
- Yap, E. L., & Greenberg, M. E. (2018). Activity-regulated transcription: Bridging the gap between neural activity and behavior. Neuron, 100(2), 330–348. https://doi.org/10.1016/j.neuron.2018.10.013
- Yu, H., Guo, Y., Zhao, Y., Zhou, F., Zhao, K., Li, M., Wen, J., He, Z., Zhu, X., & He, X. (2019). Both insufficient and excessive glucocorticoid receptor-mediated signaling impair neuronal migration. Journal of Endocrinology, 242(2), 103–114.
- Yu, H., Wang, D. D., Wang, Y., Liu, T., Lee, F. S., & Chen, Z. Y. (2012). Variant brain-derived neurotrophic factor Val66Met polymorphism alters vulnerability to stress and response to antidepressants. The Journal of Neuroscience : The Official Journal of the Society for Neuroscience, 32(12), 4092–4101. https://doi.org/10.1523/JNEUROSCI.5048-11.2012
- Yu, X., & Zuo, Y. (2011). Spine plasticity in the motor cortex. Current Opinion in Neurobiology, 21(1), 169–174. https://doi.org/10.1016/j.conb.2010.07.010
- Zalachoras, I., Verhoeve, S. L., Toonen, L. J., van Weert, L. T., van Vlodrop, A. M., Mol, I. M., Meelis, W., de Kloet, E. R., & Meijer, O. C. (2016). Isoform switching of steroid receptor co-activator-1 attenuates glucocorticoid-induced anxiogenic amygdala CRH expression. Molecular Psychiatry, 21(12), 1733–1739. https://doi.org/10.1038/mp.2016.16
- Zhang, S. J., Zou, M., Lu, L., Lau, D., Ditzel, D. A., Delucinge-Vivier, C., Aso, Y., Descombes, P., & Bading, H. (2009). Nuclear calcium signaling controls expression of a large gene pool: Identification of a gene program for acquired neuroprotection induced by synaptic activity. PLoS Genetics, 5(8), e1000604. https://doi.org/10.1371/journal.pgen.1000604
- Zhang, X., Clark, A. F., & Yorio, T. (2008). FK506-binding protein 51 regulates nuclear transport of the glucocorticoid receptor beta and glucocorticoid responsiveness. Investigative Ophthalmology & Visual Science, 49(3), 1037–1047. https://doi.org/10.1167/iovs.07-1279