Abstract
Stress is a potent environmental factor that can confer potent and enduring effects on brain structure and function. Exposure to stress during early life (ELS) has been linked to a wide range of consequences later in life. In particular, ELS exerts lasting effects on neurogenesis in the adult hippocampus, suggesting that ELS is a significant regulator of adult neural stem cell numbers and function. Here, we investigated the effect of ELS on cell proliferation and the numbers of neural stem/precursor cells in another neurogenic region: the hypothalamus of adult mice. We show that ELS has long-term suppressive effects on cell proliferation in the hypothalamic parenchyma and reduces the numbers of putative hypothalamic neural stem/precursor cells at 4 months of age. Specifically, ELS reduced the number of PCNA + cells present in hypothalamic areas surrounding the 3rd ventricle with a specific reduction in the proliferation of Sox2+/Nestin-GFP + putative stem cells present in the median eminence at the base of the 3rd ventricle. Furthermore, ELS reduced the total numbers of β-tanycytes lining the ventral 3rd ventricle, without affecting α-tanycyte numbers in more dorsal areas. These results are the first to indicate that ELS significantly reduces proliferation and β-tanycyte numbers in the adult hypothalamus, and may have (patho)physiological consequences for metabolic regulation or other hypothalamic functions in which β-tanycytes are involved.
LAY SUMMARY
We show for the first time, long-lasting effects of exposure to early life stress on cellular plasticity in the hypothalamus of adult mice.
Stress in the first week of life resulted in reduced numbers of (proliferating) stem cells in specific subregions of the hypothalamus at an adult age.
This loss of stem cells and decreased proliferation highlights how early life stress can affect hypothalamic functions in later life.
Introduction
The hypothalamus is a central regulator of the neuroendocrine system and controls key physiological processes such as temperature homeostasis, food intake, reproduction and circadian rhythms. Although many hypothalamic functions are already established during embryonic development (Maggi et al., Citation2014; Romanov et al., Citation2020), the identification of putative neural stem/precursor cells (NSPCs) in the proximity of the adult 3rd ventricle (Pellegrino et al., Citation2018; Prevot et al., Citation2018; Xu et al., Citation2005) suggests that considerable cellular plasticity remains in this region that could also be modified by environmental stimuli.
In addition to the hippocampus, several ‘non-canonical’ neurogenic niches have been recently described in the adult mammalian brain, including the rodent hypothalamus (Yoo and Blackshaw Citation2018; Feliciano et al., Citation2015; Kokoeva et al., Citation2007; Xu et al., Citation2005). This novel hypothalamic neurogenic niche has been observed in mouse, sheep and human brains (Batailler et al., Citation2014; Pellegrino et al., Citation2018). The identity of putative NSPC populations generating new neurons in the hypothalamus is still being investigated, but most studies point toward populations of tanycytes that express NSPC markers such as Nestin, Sox2, or vimentin (Haan et al., Citation2013; Lee et al., Citation2012; Robins et al., Citation2013a). In mice, hypothalamic tanycytes are divided in four types based on their cell type marker expression and localization: α1, α2, β1 and β2. While all α- and β-tanycytes co-express putative NSPC markers such as Sox2 and Nestin, they differ in their localization relative to the 3rd ventricle wall. α-Tanycytes are located more dorsally, while β-tanycytes occupy more ventral parts of the 3rd ventricle ependyma (Goodman and Hajihosseini, Citation2015). In addition, while the processes of α-tanycytes project horizontally to terminate in close proximity to the dorsomedial and ventromedial hypothalamic nucleus (α1) as well as the dorsomedial part of the arcuate nucleus (α2), the processes from β-tanycytes curve to contact the hypothalamic parenchymal capillaries in the arcuate nucleus (β1) or the portal blood vessels of the median eminence (ME) (β2) (Prevot et al., Citation2018; Rizzoti and Lovell-Badge, Citation2017; Rodriguez et al., Citation2005).
Interestingly, adult hypothalamic NSPCs have been associated with the regulation of well-characterized hypothalamic functions, such as metabolic regulation and the control of energy metabolism (Bolborea and Dale, Citation2013; Goodman and Hajihosseini, Citation2015; Lee et al., Citation2012). Early life stress (ELS) is a potent environmental factor that can confer enduring effects on brain structure and function. In particular, ELS exerts lasting effects on neurogenesis in the adult hippocampus, suggesting that ELS is a significant regulator of general NSPC function (Korosi et al., Citation2012; Lucassen et al., Citation2013). Interestingly, ELS has been increasingly linked to alterations in hypothalamic functions later in life, which raises the question whether hypothalamic NSPCs may also be involved and responsive to ELS (Balland et al., Citation2014; Maniam et al., Citation2015; Yam et al., Citation2017).
Therefore, we here investigated the effect of ELS on the numbers and proliferation of putative NSPCs in the hypothalamus of adult 4-month-old Nestin-GFP mice, which allow to readily identify endogenous NSPCs. We used a well-established ELS paradigm known to alter adult hippocampal neurogenesis (Naninck et al., Citation2015; Walker et al., Citation2017). Our results indicate that ELS exerts long term suppressive effects on cell proliferation in the ME. Furthermore, ELS specifically reduced the number of β-tanycytes around the ventral 3rd ventricle, without affecting the numbers of α-tanycytes in more dorsal areas.
Materials and methods
Mice
All experiments were approved by the Animal Experiment Committee of the University of Amsterdam, and were performed in accordance to the European Union (EU) directive 2010/63/EU. Parent mice, bred in-house, were housed in same sex cages of 2–3 animals per cage in standard conditions (temperature 20–22 °C, 40–60% humidity, standard chow and water ad libitum and a 12/12 h light/dark schedule with lights turned on at 8am). During mating, transgenic Nestin-GFP ± male mice (Mignone et al., Citation2004) were housed with wildtype C57/Bl6J female mice in a 2:1 female:male ratio. After 1 week, pregnant females were single-housed in a standard filter-top covered cage and checked every 24 h for pups. If pups were born prior to 9 AM, the preceding day was designated as the day of birth. At postnatal (P) day 2, dam and pup litters were randomly assigned to ES or control (CTL) groups. Throughout the experiment, all handling was kept to a minimum.
Chronic early life stress
The limited nesting/bedding material model of ELS was used from P2 to P9 as described by Rice et al. (Rice et al., Citation2008). At P2, all litters were reduced to 6 pups and cage changes were implemented. Prior to the ELS paradigm, litters were weighed to obtain average bodyweights per pup. Early life stress (ELS) groups were housed in cages containing minimal sawdust and a fine-gauge stainless steel mesh that was placed 1 cm above the floor. A small, 2.5x5cm, section of cotton was available to build the nest (Technilab-BMI, Someren, The Netherlands). Control (CTL) cages had standard amounts of sawdust covering the entire floor of the cage and more nesting fabric using a 5x5cm section of cotton nesting material (Technilab-BMI, Someren, The Netherlands). At P9, pups were weighed and moved to standard cages. At P28, pups were weaned and housed in same sex groups of 2–3 mice per cage under standard conditions until further processing.
Tissue preparation
4-month-old male mice were anesthetized by an intra-peritoneal injection of pentobarbital (Euthasol, 120 mg/kg) before transcardial perfusion with 0.9% saline followed by 4% paraformaldehyde (PFA) in 0.1 M phosphate buffer (PB) at pH 7.4. After dissection, brains were post-fixed overnight in the same 4% PFA solution at 4 °C and stored in 0.1 M PB with 0.01% sodium azide at 4 °C. To facilitate slicing, brains were cryoprotected in 15% sucrose in 0.1 M PB for four hours, which was increased to 30% in 0.1 M PB overnight. A 40 μm-thick 6-slice parallel rostro-coronal series was produced of each frozen brain using a Leica Jung HN 40 microtome. Sections were then stored at −20 °C in antifreeze solution (30% ethylene glycol, 20% glycerol, 50% 0.05 M PBS).
Immunohistochemistry
Putative NSPCs in the adult hypothalamus were identified as cells with tanycyte morphology, located at the wall of the 3rd ventricle and detected by double-labeling with GFP (expressed from the promoter of the intermediate filament protein nestin, present in the Nestin-GFP mice) and the transcription factor Sox2 (Zhang et al., Citation2017). Proliferating cells were identified after immunolabelling for the proliferating cell nuclear antigen (PCNA).
PFA-fixed slices with an interval of ∼240 μm were mounted on glass slides (Superfrost Plus slides, Thermo Fisher, Bremen, Germany) in 0.01 M PB and dried overnight. The slices underwent an antibody retrieval procedure, consisting of heating in 0.01 M citrate buffer (pH 6.0) in a standard microwave oven (Samsung M6235). Samples were heated on full power (800 W) until boiling. Power was then reduced (260 W) to stay just below the boiling point for a total 20 min. After cooling down to room temperature, slides were washed 3 × 5 min in 0.05 M pH 7.6 tris-buffered saline (TBS). Sections were then blocked with mouse FAB fragments to prevent nonspecific binding of secondary mouse antibodies, followed by blocking steps with 1% normal goat and donkey serum.
Primary antibodies included polyclonal rabbit Anti-Sox2 (Millipore, 1:500), polyclonal chicken Anti-GFP (Abcam, 1:500), rabbit anti NeuN (Millipore, 1:1000) and monoclonal mouse anti-PCNA (Dako, 1:400). Antibodies were delivered in 300 μl per slide in blocking mix of 1% normal donkey serum, 1% normal goat serum and 0.3% Triton X-100 in 0.05 M TBS. Slides were incubated in primary antibodies for one hour at room temperature (RT) followed by overnight incubation at 4 °C. After 5 × 5 min 0.05 M TBS washes, the following secondary antibodies were incubated for two hours at RT in the same blocking medium: polyclonal donkey anti-rabbit Alexa647 (Invitrogen, 1:500), polyclonal goat anti-mouse Alexa594 (Invitrogen, 1:500) and polyclonal goat anti-chicken Alexa488 (Invitrogen, 1:500). Negative controls were included in which the primary antibodies were omitted from the first incubation. Dentate gyrus-containing sections were used as positive control for double and triple stains with Sox2, PCNA and GFP antibodies. After TBS and TB washes, slides were simultaneously counterstained and coverslipped in DAPI-containing VectaShield mounting medium (Vector Laboratories).
Confocal microscopy
A Zeiss LSM 510 confocal laser-scanning microscope (10x air, 40x water objectives) was used to obtain Z-stack images of the ventricular wall and surrounding brain areas. A 10x overview was taken and tanycyte subpopulations were identified using the unique orientation of the GFP + processes (Robins et al., Citation2013a). 40x Z-stack images with 1 µm intervals were then produced of the hypothalamic ME and the ventricle walls adjacent to the arcuate nucleus, thus containing β- and α-tanycytes respectively. Cell numbers were assessed using the cell counter plug-in for ImageJ (Schneider et al., Citation2012). The observer was unaware of the identity of the sections at the time of counting. Cells counts were normalized to the ventricle wall, ME, or hypothalamic parenchymal volumes to produce values of cells per mm3.
Statistical analyses
Graphpad Prism 6.0 (Graphpad software, San Diego, CA, USA) was used to carry out two-tailed unpaired t-tests for significant differences between ELS and CTL groups taking into account normal distribution of data and equal variances. Data is expressed as mean ± standard error of the mean (SEM) of cell counts. In all cases, differences were considered statistically significant when P < 0.05.
Results
First, we validated a previously described chronic ELS paradigm based on restricted availability of nesting/bedding material from postnatal day (P) 2 to 9, here in Nestin-GFP transgenic mice, that are commonly used as a NSPC reporter line in other brain areas (Mignone et al., Citation2004; Naninck et al., Citation2015). As expected, pups exposed to ELS showed a significant decrease in body weight at the end of a 7 day-long exposure to ELS at P9 (t-test, N = 9, CTL 5.021 ± 0.1282 gr vs ELS 4.108 ± 0.1702 gr, p = 0.0006), which correlated with a significant decrease in body weight gain during the ELS paradigm from P2-P9, as compared to the control group (t-test, N = 9, CTL 3.508 ± 0.1131 gr vs ELS 2.730 ± 0.1705 gr, p = 0.0016) (), both well-characterized physiological signs indicative of the induction of chronic ELS in mouse pups (Naninck et al., Citation2015).
Figure 1. ELS reduces the number of β-tanycytes in the hypothalamus of adult male mice. The ELS paradigm consisting of limited nesting/bedding-material results in significant reductions in body weight (A) and body weight gain between P2 and P9 (B). In the hypothalamus, tanycytes were co-labeled with GFP and SOX2 (C). Nuclear DNA was labeled with DAPI. α-tanycytes and β-tanycytes were quantified in the hypothalamic areas outlined dashed lines. Adult mice exposed to ELS between P2 and P9 showed a significant decrease in the number of β-tanycytes (E), while no differences in the numbers of α-tanycytes were observed (D). BW: body weight; *p < 0.05.
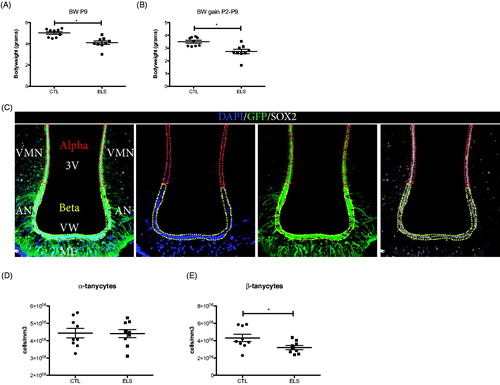
In Nestin-GFP mice, tanycytes can be distinguished guided by GFP fluorescence from ependymal cells in the ventricular wall by their characteristic morphology. α-tanycytes can be observed projecting horizontal processes terminating in close proximity to the dorsomedial and ventromedial hypothalamic nucleus (α1) or the dorsomedial part of the arcuate nucleus (α2), while the processes from β-tanycytes curve to contact the hypothalamic parenchyma in the arcuate nucleus (β1) or ME (β2) ().
In 4-month-old male Nestin-GFP mice, we observed abundant GFP+/Sox2+ cell numbers in the wall of the 3rd ventricle and in the parenchyma of the ME (). ELS resulted in a significant decrease in the numbers of GFP+/Sox2+ cells with β-tanycyte morphology located in more ventral parts of the 3rd ventricle ependyma (T-test, N = 9, CTL 4.312E6 ± 416600 cells/mm3 vs ELS 3.211E6 ± 232294 cells/mm3, p = 0.0347) (). In contrast, the numbers of GFP+/Sox2+ cells with α-tanycyte morphology located in more dorsal areas were unaffected by ELS (t-test, N = 9, CTL 4.437E6 ± 277677 cells/mm3 vs ELS 4.409E6 ± 235789 cells/mm3, p = 0.9396 (), indicating a specific effect on the β-tanycyte population.
To assess the putative neurogenic potential of hypothalamic NSPCs we quantified cell proliferation. Due to the low proliferation capacity of adult tanycytes, some studies have used intracerebroventricular BrdU labeling, repeated BrdU injections for long periods of time, or lineage tracing of the GLutamate ASpartate Transporter (GLAST) (Kokoeva et al., Citation2007; Robins et al., Citation2013a, Citation2013b), while others used the expression of endogenous proliferation markers such as PCNA (Ma et al., Citation2015; Pierce and Xu, Citation2010). As invasive procedures, such as (repeated) injections, induce stress to mice (Drude et al., Citation2011), we decided to use PCNA as proliferation indicator ( and Supplementary Table 1). We found PCNA+ cells in hypothalamic areas surrounding the 3rd ventricle (Ventromedial Nucleus of the hypothalamus, Arcuate Nucleus and the Median Eminence) (). Specifically, numerous PCNA+/Sox2+/Nestin-GFP + cells were located in the parenchyma of the median eminence (). Interestingly, ELS had a significant effect on the total numbers of PCNA + cells present in these hypothalamic areas (t-test, N = 8 for CTL, N = 5 for ELS, CTL 591.2 ± 136.5 cells/mm3 vs ELS 228.7 ± 41.36 cells/mm3, p = 0.0346, Welch’s correction for unequal variance) () and specifically on the numbers of PCNA+/Sox2+/Nestin-GFP + cells present in the median eminence, at the base of the 3rd ventricle (t-test, N = 7 for CTL, N = 9 for ELS, CTL 393.3 ± 98.19 cells/mm3 vs ELS 141.2 ± 50.91 cells/mm3, p = 0.029 (). No PCNA labeling was observed in postmitotic NeuN + neurons (Figure S1).
Figure 2. ELS reduces cell proliferation in the hypothalamus of adult male mice. Proliferating (PCNA+) cells, were found in parenchymal areas surrounding the 3rd ventricle but not on the ventricular wall of the hypothalamus in Nestin-GFP mice (A). Representative image from a male control mouse. PCNA + cells were found in the parenchyma (B) and there, total numbers of proliferating PCNA + cells and putative proliferating NSCs positive for Nestin-GFP, SOX2 and PCNA in the ME were quantified. ELS resulted in a significant reduction of the total numbers of PCNA + cells (C) and specifically in a significant reduction of PCNA+/SOX2+/Nestin + proliferative cells (D) in the ME. *p < 0.05.
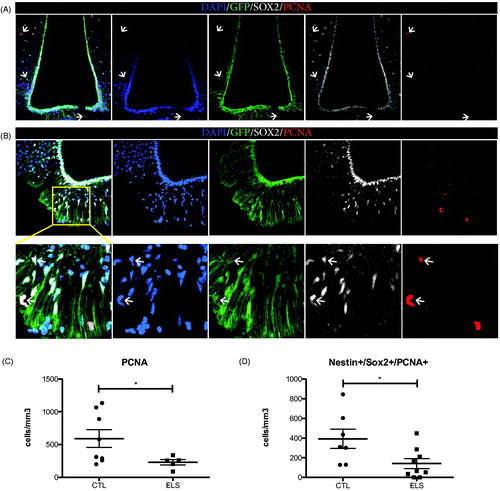
Discussion
Here, we show for the first time that chronic ELS, a well-characterized inhibitor of neurogenesis in other neurogenic areas of the adult brain (Korosi et al., Citation2012; Mirescu et al., Citation2004; Naninck et al., Citation2015; Oomen et al., Citation2010), also reduces the number of β-tanycytes in the hypothalamus of adult, 4-month-old male mice, accompanied by an overall reduction in proliferating cells in the parenchymal areas surrounding the 3rd ventricle. Furthermore, we found a strong reduction in proliferation of Nestin-GFP+/Sox2+ cells in the ME, which may be considered putative NSPCs. Our results indicate that ELS has long term effects on cell proliferation in the ventral parenchymal areas of the 3rd ventricle and the ME, together with a lasting decrease in the number of β-tanycytes present in the 3rd ventricular wall.
The hypothalamus is a conglomerate of cellular nuclei surrounding the 3rd ventricle. One of its main functions is the maintenance of homeostasis through the (feedback) regulation of diverse physiological functions, such as feeding, circadian rhythms, body temperature and energy metabolism (Myers and Olson, Citation2012). Recent studies have identified a diet-responsive proliferative zone in the ME of the ventral adult hypothalamus, in which Nestin+/Sox2+ β-tanycytes generate new neurons (Lee et al., Citation2012). Although the evidence for ongoing plasticity and neurogenesis in the adult hypothalamus in mice is clear, most of it stems from work done on young-adults (Yoo and Blackshaw, Citation2018). Whether tanycytes also show neurogenic activity at later ages remains unknown.
Earlier work has confirmed the presence of tanycytes in the deeper layers of the ME (Miranda-Angulo et al., Citation2014; Wittmann et al., Citation2017). Although Sox2+ NSPCs in the hypothalamus are widely considered to be tanycytes, caution should be taken in interpreting the result we report here, as a previous report has indicated that a subset of Sox2+ cells in the ME are neurogenic NG2+ glia cells (Robins et al., Citation2013b).
Here, we show decreased numbers of proliferative cells in the ME, quantified as PCNA+/Sox2+/Nestin-GFP + cells. Previous observations have shown that some PCNA antibodies can also label postmitotic cells (Ino and Chiba, Citation2000). However, in our hands, PCNA was not detected in postmitotic NeuN + neurons (Gusel’nikova and Korzhevskiy, Citation2015) in the ME, indicating preferential labeling of proliferative cells in our experimental conditions.
In other brain areas such as the hippocampus or the olfactory bulb, both a stress-induced decrease in proliferation, or antidepressant-induced excessive proliferation at young age that is followed by a subsequent depletion of the NSPC pool, result in an overall loss of adult neurogenesis at later ages (Sierra et al., Citation2015; Zhao et al., Citation2008). These changes have been linked to impairments in some of the functions in which these areas are involved (Lledo and Valley, Citation2016; Toda et al., Citation2019). Although not assessed in this study, it is plausible to assume from work on other brain areas and the neurogenic dynamics therein, that a reduction in proliferation of putative NSPCs is likely to result in a decrease in neurogenesis and that this change may have functional effects on hypothalamic functioning.
Direct inhibition of adult ME neurogenesis results in impaired hypothalamic functions, like weight regulation, energy metabolism and body adiposity, and alterations in the response to a high-fat diet (Lee et al., Citation2012; McNay et al., Citation2012; Yoo et al., Citation2020). Importantly, ELS itself induces changes in body adiposity, leptin signaling and diet responsivity (Maniam et al., Citation2015; Yam et al., Citation2017), while tanycytes in the ME regulate the transport of peripherally released leptin into the mediobasal hypothalamus and thereby also affect energy metabolism (Balland et al., Citation2014). Therefore, the ELS-induced reduction in the number of β-tanycytes and decrease in proliferation in hypothalamic areas surrounding the 3rd ventricle that we describe here may contribute to the effects of ELS on hypothalamic (patho)physiology.
Supplemental Material
Download MS Excel (12.4 KB)Acknowledgments
We acknowledge the assistance of Ronald Breedijk and Mark Hink at the Leeuwenhoek Centre for Advanced Microscopy, University of Amsterdam for providing technical assistance with the confocal microscope.
Disclosure statement
The authors declare that they have no conflict of interest.
Additional information
Funding
Notes on contributors
Pascal Bielefeld
Pascal Bielefeld is a neuroscience PostDoc at the university of Amsterdam, currently at Babraham Institute, Cambridge, United Kingdom.
Maralinde R. Abbink
Maralinde R. Abbink is a neuroscience PhD candidate at the university of Amsterdam, currently at Novartis Netherlands.
Anna R. Davidson
Anna R. Davidson is a neuroscience undergrad student at the university of Amsterdam, currently at Solentim, United Kingdom.
Niels Reijner
Niels Reijner is a neuroscience undergrad student at the university of Amsterdam.
Oihane Abiega
Oihane Abiega is a neuroscience PostDoc at the university of Amsterdam.
Paul J. Lucassen
Paul J. Lucassen is a professor of brain plasticity at the university of Amsterdam.
Aniko Korosi
Aniko Korosi is an associate professor in neuroscience at the university of Amsterdam.
Carlos P. Fitzsimons
Carlos P. Fitzsimons is an assistant professor in neuroscience at the university of Amsterdam.
References
- Balland, E., Dam, J., Langlet, F., Caron, E., Steculorum, S., Messina, A., Rasika, S., Falluel-Morel, A., Anouar, Y., Dehouck, B., Trinquet, E., Jockers, R., Bouret, S. G., & Prévot, V. (2014). Hypothalamic tanycytes are an ERK-gated conduit for leptin into the brain. Cell Metabolism, 19(2), 293–301. https://doi.org/10.1016/j.cmet.2013.12.015
- Batailler, M., Droguerre, M., Baroncini, M., Fontaine, C., Prevot, V., & Migaud, M. (2014). DCX-expressing cells in the vicinity of the hypothalamic neurogenic niche: A comparative study between mouse, sheep, and human tissues. The Journal of Comparative Neurology, 522(8), 1966–1985. https://doi.org/10.1002/cne.23514
- Bolborea, M., & Dale, N. (2013). Hypothalamic tanycytes: Potential roles in the control of feeding and energy balance. Trends in Neurosciences, 36(2), 91–100. https://doi.org/10.1016/j.tins.2012.12.008
- Drude, S., Geissler, A., Olfe, J., Starke, A., Domanska, G., Schuett, C., & Kiank-Nussbaum, C. (2011). Side effects of control treatment can conceal experimental data when studying stress responses to injection and psychological stress in mice. Lab Animal, 40(4), 119–128. https://doi.org/10.1038/laban0411-119
- Feliciano, D. M., Bordey, A., & Bonfanti, L. (2015). Noncanonical sites of adult neurogenesis in the mammalian brain. Cold Spring Harbor Perspectives in Biology, 7(10), a018846. https://doi.org/10.1101/cshperspect.a018846
- Goodman, T., & Hajihosseini, M. K. (2015). Hypothalamic tanycytes-masters and servants of metabolic, neuroendocrine, and neurogenic functions. Frontiers in Neuroscience, 9, 387. https://doi.org/10.3389/fnins.2015.00387
- Gusel’nikova, V. V., & Korzhevskiy, D. E. (2015). NeuN as a neuronal nuclear antigen and neuron differentiation marker. Acta Naturae, 7(2), 42–47. https://doi.org/10.32607/20758251-2015-7-2-42-47
- Haan, N., Goodman, T., Najdi-Samiei, A., Stratford, C. M., Rice, R., El Agha, E., Bellusci, S., & Hajihosseini, M. K. (2013). Fgf10-expressing tanycytes add new neurons to the appetite/energy-balance regulating centers of the postnatal and adult hypothalamus. The Journal of Neuroscience: The Official Journal of the Society for Neuroscience, 33(14), 6170–6180. https://doi.org/10.1523/JNEUROSCI.2437-12.2013
- Ino, H., & Chiba, T. (2000). Expression of proliferating cell nuclear antigen (PCNA) in the adult and developing mouse nervous system. Brain Research. Molecular Brain Research, 78(1–2), 163–174. https://doi.org/10.1016/S0169-328X(00)00092-9
- Kokoeva, M. V., Yin, H., & Flier, J. S. (2007). Evidence for constitutive neural cell proliferation in the adult murine hypothalamus. The Journal of Comparative Neurology, 505(2), 209–220. https://doi.org/10.1002/cne.21492
- Korosi, A., Naninck, E. F. G., Oomen, C. A., Schouten, M., Krugers, H., Fitzsimons, C., & Lucassen, P. J. (2012). Early-life stress mediated modulation of adult neurogenesis and behavior. Behavioural Brain Research, 227(2), 400–409. https://doi.org/10.1016/j.bbr.2011.07.037
- Lee, D. A., Bedont, J. L., Pak, T., Wang, H., Song, J., Miranda-Angulo, A., Takiar, V., Charubhumi, V., Balordi, F., Takebayashi, H., Aja, S., Ford, E., Fishell, G., & Blackshaw, S. (2012). Tanycytes of the hypothalamic median eminence form a diet-responsive neurogenic niche. Nature Neuroscience, 15(5), 700–702. https://doi.org/10.1038/nn.3079
- Lledo, P. M., & Valley, M. (2016). Adult olfactory bulb neurogenesis. Cold Spring Harbor Perspectives in Biology, 8(8), a018945. https://doi.org/10.1101/cshperspect.a018945
- Lucassen, P. J., Naninck, E. F. G., van Goudoever, J. B., Fitzsimons, C., Joels, M., & Korosi, A. (2013). Perinatal programming of adult hippocampal structure and function; emerging roles of stress, nutrition and epigenetics. Trends in Neurosciences, 36(11), 621–631. https://doi.org/10.1016/j.tins.2013.08.002
- Ma, M.-S., Brouwer, N., Wesseling, E., Raj, D., van der Want, J., Boddeke, E., Balasubramaniyan, V., & Copray, S. (2015). Multipotent stem cell factor UGS148 is a marker for tanycytes in the adult hypothalamus. Molecular and Cellular Neurosciences, 65, 21–30. https://doi.org/10.1016/j.mcn.2015.02.002
- Maggi, R., Zasso, J., & Conti, L. (2014). Neurodevelopmental origin and adult neurogenesis of the neuroendocrine hypothalamus. Frontiers in Cellular Neuroscience, 8, 440 https://doi.org/10.3389/fncel.2014.00440
- Maniam, J., Antoniadis, C. P., Wang, K. W., & Morris, M. J. (2015). Early life stress induced by limited nesting material produces metabolic resilience in response to a high-fat and high-sugar diet in male rats. Frontiers in Endocrinology, 6, 138 https://doi.org/10.3389/fendo.2015.00138
- McNay, D. E. G., Briançon, N., Kokoeva, M. V., Maratos-Flier, E., & Flier, J. S. (2012). Remodeling of the arcuate nucleus energy-balance circuit is inhibited in obese mice. The Journal of Clinical Investigation, 122(1), 142–152. https://doi.org/10.1172/JCI43134
- Mignone, J. L., Kukekov, V., Chiang, A.-S., Steindler, D., & Enikolopov, G. (2004). Neural stem and progenitor cells in nestin-GFP transgenic mice.The Journal of Comparative Neurology, 469(3), 311–324. https://doi.org/10.1002/cne.10964
- Miranda-Angulo, A. L., Byerly, M. S., Mesa, J., Wang, H., & Blackshaw, S. (2014). Rax regulates hypothalamic tanycyte differentiation and barrier function in mice. The Journal of Comparative Neurology, 522(4), 876–899. https://doi.org/10.1002/cne.23451
- Mirescu, C., Peters, J. D., & Gould, E. (2004). Early life experience alters response of adult neurogenesis to stress. Nature Neuroscience, 7(8), 841–846. https://doi.org/10.1038/nn1290
- Myers, M. G., Jr., & Olson, D. P. (2012). Central nervous system control of metabolism. Nature, 491(7424), 357–363. https://doi.org/10.1038/nature11705
- Naninck, E. F. G., Hoeijmakers, L., Kakava-Georgiadou, N., Meesters, A., Lazic, S. E., Lucassen, P. J., & Korosi, A. (2015). Chronic early life stress alters developmental and adult neurogenesis and impairs cognitive function in mice. Hippocampus, 25(3), 309–328. https://doi.org/10.1002/hipo.22374
- Oomen, C. A., Soeters, H., Audureau, N., Vermunt, L., van Hasselt, F. N., Manders, E. M. M., Joëls, M., Lucassen, P. J., & Krugers, H. (2010). Severe early life stress hampers spatial learning and neurogenesis, but improves hippocampal synaptic plasticity and emotional learning under high-stress conditions in adulthood. The Journal of Neuroscience: The Official Journal of the Society for Neuroscience, 30(19), 6635–6645. https://doi.org/10.1523/JNEUROSCI.0247-10.2010
- Pellegrino, G., Trubert, C., Terrien, J., Pifferi, F., Leroy, D., Loyens, A., Migaud, M., Baroncini, M., Maurage, C.-A., Fontaine, C., Prévot, V., & Sharif, A. (2018). A comparative study of the neural stem cell niche in the adult hypothalamus of human, mouse, rat and gray mouse lemur (Microcebus murinus).The Journal of Comparative Neurology, 526(9), 1419–1443. https://doi.org/10.1002/cne.24376
- Pierce, A. A., & Xu, A. W. (2010). De novo neurogenesis in adult hypothalamus as a compensatory mechanism to regulate energy balance. The Journal of Neuroscience, 30(2), 723–730. https://doi.org/10.1523/JNEUROSCI.2479-09.2010
- Prevot, V., Dehouck, B., Sharif, A., Ciofi, P., Giacobini, P., & Clasadonte, J. (2018). The versatile tanycyte: A hypothalamic integrator of reproduction and energy metabolism. Endocrine Reviews, 39(3), 333–368. https://doi.org/10.1210/er.2017-00235
- Rice, C. J., Sandman, C. A., Lenjavi, M. R., & Baram, T. Z. (2008). A novel mouse model for acute and long-lasting consequences of early life stress. Endocrinology, 149(10), 4892–4900. https://doi.org/10.1210/en.2008-0633
- Rizzoti, K., & Lovell-Badge, R. (2017). Pivotal role of median eminence tanycytes for hypothalamic function and neurogenesis. Molecular and Cellular Endocrinology, 445, 7–13. https://doi.org/10.1016/j.mce.2016.08.020
- Robins, S. C., Stewart, I., McNay, D. E., Taylor, V., Giachino, C., Goetz, M., Ninkovic, J., Briancon, N., Maratos-Flier, E., Flier, J. S., Kokoeva, M. V., & Placzek, M. (2013a). alpha-Tanycytes of the adult hypothalamic third ventricle include distinct populations of FGF-responsive neural progenitors. Nature Communications, 4, 2049. https://doi.org/10.1038/ncomms3049
- Robins, S. C., Trudel, E., Rotondi, O., Liu, X., Djogo, T., Kryzskaya, D., Bourque, C. W., & Kokoeva, M. V. (2013b). Evidence for NG2-glia derived, adult-born functional neurons in the hypothalamus. PLoS One, 8(10), e78236 https://doi.org/10.1371/journal.pone.0078236
- Rodriguez, E., Blazquez, J., Pastor, F., Pelaez, B., Pena, P., Peruzzo, B., & Amat, P. (2005). Hypothalamic tanycytes: A key component of brain-endocrine interaction. International Review of Cytology, 247, 89–164. https://doi.org/10.1016/S0074-7696(05)47003-5
- Romanov, R. A., Tretiakov, E. O., Kastriti, M. E., Zupancic, M., Häring, M., Korchynska, S., Popadin, K., Benevento, M., Rebernik, P., Lallemend, F., Nishimori, K., Clotman, F., Andrews, W. D., Parnavelas, J. G., Farlik, M., Bock, C., Adameyko, I., Hökfelt, T., Keimpema, E., & Harkany, T. (2020). Molecular design of hypothalamus development. Nature, 582(7811), 246–252. https://doi.org/10.1038/s41586-020-2266-0
- Schneider, C. A., Rasband, W. S., & Eliceiri, K. W. (2012). NIH Image to ImageJ: 25 years of image analysis. Nature Methods, 9(7), 671–675. https://doi.org/10.1038/nmeth.2089
- Sierra, A., Martín-Suárez, S., Valcárcel-Martín, R., Pascual-Brazo, J., Aelvoet, S.-A., Abiega, O., Deudero, J. J., Brewster, A. L., Bernales, I., Anderson, A. E., Baekelandt, V., Maletić-Savatić, M., & Encinas, J. M. (2015). Neuronal hyperactivity accelerates depletion of neural stem cells and impairs hippocampal neurogenesis. Cell Stem Cell, 16(5), 488–503. https://doi.org/10.1016/j.stem.2015.04.003
- Toda, T., Parylak, S. L., Linker, S. B., & Gage, F. H. (2019). The role of adult hippocampal neurogenesis in brain health and disease. Molecular Psychiatry, 24(1), 67–87. https://doi.org/10.1038/s41380-018-0036-2
- Walker, C.-D., Bath, K. G., Joels, M., Korosi, A., Larauche, M., Lucassen, P. J., Morris, M. J., Raineki, C., Roth, T. L., Sullivan, R. M., Taché, Y., & Baram, T. Z. (2017). Chronic early life stress induced by limited bedding and nesting (LBN) material in rodents: Critical considerations of methodology, outcomes and translational potential. Stress (Amsterdam, Netherlands), 20(5), 421–448. https://doi.org/10.1080/10253890.2017.1343296
- Wittmann, G., Farkas, E., Szilvásy-Szabó, A., Gereben, B., Fekete, C., & Lechan, R. M. (2017). Variable proopiomelanocortin expression in tanycytes of the adult rat hypothalamus and pituitary stalk. The Journal of Comparative Neurology, 525(3), 411–441. https://doi.org/10.1002/cne.24090
- Xu, Y., Tamamaki, N., Noda, T., Kimura, K., Itokazu, Y., Matsumoto, N., Dezawa, M., & Ide, C. (2005). Neurogenesis in the ependymal layer of the adult rat 3rd ventricle. Experimental Neurology, 192(2), 251–264. https://doi.org/10.1016/j.expneurol.2004.12.021
- Yam, K. Y., Naninck, E. F. G., Abbink, M. R., la Fleur, S. E., Schipper, L., van den Beukel, J. C., Grefhorst, A., Oosting, A., van der Beek, E. M., Lucassen, P. J., & Korosi, A. (2017). Exposure to chronic early-life stress lastingly alters the adipose tissue, the leptin system and changes the vulnerability to western-style diet later in life in mice. Psychoneuroendocrinology, 77, 186–195. https://doi.org/10.1016/j.psyneuen.2016.12.012
- Yoo, S., & Blackshaw, S. (2018). Regulation and function of neurogenesis in the adult mammalian hypothalamus. Progress in Neurobiology, 170, 53–66. https://doi.org/10.1016/j.pneurobio.2018.04.001
- Yoo, S., Cha, D., Kim, S., Jiang, L., Cooke, P., Adebesin, M., Wolfe, A., Riddle, R., Aja, S., & Blackshaw, S. (2020). Tanycyte ablation in the arcuate nucleus and median eminence increases obesity susceptibility by increasing body fat content in male mice. Glia, 68(10), 1987–2000. https://doi.org/10.1002/glia.23817
- Zhang, Y., Kim, M. S., Jia, B., Yan, J., Zuniga-Hertz, J. P., Han, C., & Cai, D. (2017). Hypothalamic stem cells control ageing speed partly through exosomal miRNAs. Nature, 548(7665), 52–57. https://doi.org/10.1038/nature23282
- Zhao, C., Deng, W., & Gage, F. H. (2008). Mechanisms and functional implications of adult neurogenesis. Cell, 132(4), 645–660. https://doi.org/10.1016/j.cell.2008.01.033