Abstract
Hypothalamic-pituitary-adrenal (HPA) axis dynamics are disrupted by opioids and may be involved in substance abuse; this persists during withdrawal and abstinence and is associated with co-morbid sleep disruption leading to vulnerability to relapse. We hypothesized that chronic sleep restriction (SR) alters the HPA axis diurnal rhythm and the sexually dimorphic response to acute stressor during opioid abstinence. We developed a rat model to evaluate the effect of persistent sleep loss during opioid abstinence on HPA axis dynamics in male and female rats. Plasma ACTH and corticosterone were measured diurnally and in response to acute restraint stress in rats Before (control) compared to During subsequent opioid abstinence without or with SR. Abstinence, regardless of sleep state, led to an increase in plasma ACTH and corticosterone in the morning in males. There was a tendency for higher PM plasma ACTH during abstinence in SR males (p = 0.076). ACTH and corticosterone responses to restraint were reduced in male SR rats whereas there was a failure to achieve the post-restraint nadir in female SR rats. There was no effect of the treatments or interventions on adrenal weight normalized to body weight. SR resulted in a dramatic increase in hypothalamic PVN AVP mRNA and plasma copeptin in male but not female rats. This corresponded to the attenuation of the HPA axis stress response in SR males during opioid abstinence. We have identified a potentially unique, sexually dimorphic role for magnocellular vasopressin in the control of the HPA axis during opioid abstinence and sleep restriction.
Introduction
Opioid misuse/use disorder is a personal, familial, and national crisis of historic proportions (Humphreys et al., Citation2022; Lim et al., Citation2022; Rao et al., Citation2021). Most people who stop misusing opioids relapse early in the abstinence period (Ivers et al., Citation2018); this presents a window of opportunity for mitigating the risk of relapse. Persistent sleep deficiency, a common finding in opioid users during abstinence, is a significant comorbidity that may increase opioid drug seeking (Burke et al., Citation2008; Coffey et al., Citation2016; Dunn et al., Citation2018; Frers et al., Citation2021; Gillin, Citation1998; Greenwald et al., Citation2021; Hartwell et al., Citation2014; Hasler et al., Citation2012; Kaplan et al., Citation2014; Maulik et al., Citation2002; Mehtry et al., Citation2014; Mukherjee et al., Citation2021; Peles et al., Citation2006; Raj et al., Citation2000; Roth & Workshop, Citation2009; Teplin et al., Citation2006). Elucidating the mechanisms of persistent sleep deficiency as a predictor and cause of relapse in opioid use provides an opportunity for interventions aimed at decreasing this vulnerability.
Stressful events are correlated with craving for most drugs of abuse (Piazza & Le Moal, Citation1996), and the hypothalamic-pituitary-adrenal (HPA) axis response to stress is a critical factor influencing individual vulnerability to drug abuse (Aouizerate et al., Citation2006; Kreek, Citation2000; Piazza & Le Moal, Citation1996; Wemm & Sinha, Citation2019; Zhou et al., Citation2013; Zhou & Leri, Citation2016). During prolonged abstinence from opioids, HPA axis dynamics are altered in ways that are specific among drugs of abuse and relapse vulnerability (Aouizerate et al., Citation2006; Bunce et al., Citation2015; Zhou et al., Citation2013). Concurrently, sleep is markedly deficient—sleep latency is increased, sleep is fragmented by wakefulness, and sleep efficiency is poor (Burke et al., Citation2008; Howe et al., Citation1980; Mehtry et al., Citation2014; Tripathi et al., Citation2020). Sleep amounts remain decreased in patients who relapse four months after residential treatment, whereas sleep amounts increase to normal values in patients who abstain from opioid use during the same time period (Mukherjee et al., Citation2021). While opioid maintenance therapy (e.g. with methadone) may normalize basal cortisol and its diurnal rhythmicity during abstinence (Aouizerate et al., Citation2006; Kreek, Citation2000), it fails to normalize disrupted sleep (Beswick et al., Citation2003; Dunn et al., Citation2018; Kay, Citation1975; Mehtry et al., Citation2014) and dysregulated glucocorticoid negative feedback (Aouizerate et al., Citation2006; Kreek, Citation2000).
We hypothesize that chronic sleep restriction (SR) alters the HPA axis diurnal rhythm and the response to acute stressors during opioid abstinence in a sex-dependent manner. Females differ from males in HPA axis activity and stress reactivity, as well as an increased tendency toward relapse in both humans and laboratory animals (Becker & Chartoff, Citation2019; Fattore et al., Citation2008; Goel et al., Citation2014; Heck & Handa, Citation2019; Kosten & Ambrosio, Citation2002; Oyola & Handa, Citation2017).
Using our animal model of chronic sleep deficiency (Everson & Szabo, Citation2009), this study evaluated the effect of persistent sleep loss during opioid abstinence on diurnal HPA axis activity and its response to a standard restraint stress (Gehrand et al., Citation2018; Raff et al., Citation2007). We measured plasma ACTH, corticosterone and copeptin (as a surrogate for plasma vasopressin) and analyzed the expression of mRNAs for HPA axis genes we have previously shown reflect critical aspects of the HPA axis control system as well as the downstream effects of glucocorticoids (Gehrand et al., Citation2016, Citation2018, Citation2022).
Methods
Animals and general experimental procedures
All experimental procedures were approved by and under the compliance of the Institutional Animal Care and Use Committee (IACUC) at MCW. Sprague-Dawley rats (38 males and 36 females, Envigo, Madison, WI, USA) were pair housed. Rats were provided food and water ad libitum during this time and throughout the study. The week prior to surgery, all animals were singly housed and were handled daily for three days. Jugular catheterization surgery was performed at ∼13 weeks of age as described previously (Muelbl et al., Citation2022; Nawarawong et al., Citation2019). The first day post-surgery, the rats were given a subcutaneous 5 mg/kg injection of carpofen (Rimadyl, Zoetis, Inc). The next and all subsequent days, catheters were flushed twice per day for 6 days/week. Recovery from surgery was approximately 5 days before an 8-day pre-oxycodone period. All post-surgery animal testing was performed during the light phase (0600–1800 h). Measurements taken as part of a companion behavioral study that are not reported here included allodynia and/or hyperalgesia responses to mechanical (Deuis et al., Citation2017), cold (Brenner et al., Citation2012), and heat stimuli (Cheah et al., Citation2017), and 10-min exploration in a novel environment (Schmitt & Hiemke, Citation1998). These noninvasive measurements were conducted during the pre-oxycodone and the abstinence phases of the study and were separated from blood drawing or extinction testing by at least 24 h.
Self-administration
All self-administration training occurred with operant conditioning chambers (31.5 × 25.4 × 26.7 cm, Med Associates, Fairfax, VT, USA). These were equipped with infusion pumps that were computerized to give calculated drug infusions based on an animal’s weight. The first self-administration training allowed the rats to learn to press an “active” lever (side was counterbalanced within each group: equal numbers of rats were assigned the left or right lever to be active). A press of the active lever yielded on sucrose pellet and a corresponding cue light that turned on for five seconds. When the inactive lever was pressed, there was neither a pellet nor a cue light. The initial training was completed on a Fixed Ratio (FR)-1 schedule (i.e., one active lever press was required to earn the sucrose pellet). Once the criteria were met, the training was increased to an FR-2 schedule (i.e., two active lever presses were required to earn the sucrose pellet). When criteria were met for the FR-2 schedule, the rats would not be tested again until the last day of the two-week training period. On this day, the rats were given a “reminder” session to prepare for the next phase of testing. Two days after the reminder session, rats started oxycodone self-administration sessions (0.1 mg/kg/infusion, Medisca, Plattsburgh, NY, USA). The oxycodone self-administration testing was conducted for ten days with two days separating Days 1–5 (FR-2) and Days 6–10 (FR-4). Also, for the oxycodone testing, there was a ten-second timeout following a reinforcer, in which case a lever press would be recorded, but was not counted toward the next infusion reward. Approximately 18 h after the last oxycodone self-administration session, rats were given an infusion of methohexital (9 mg/kg, intravenous; JHP Pharmaceuticals, Rochester, NY) to check for catheter patency. Rats with patent catheters were then assigned to either the sleep restriction (SR, N = 16 males and 16 females) or ambulatory control (AC) group (N = 16 males and 15 females). Group assignment was counterbalanced by oxycodone intake during self-administration.
Experimental sequence
The successive experimental periods of pre-oxycodone baseline, oxycodone self-administration, and oxycodone abstinence with and without chronic sleep restriction are provided in . We chose a priori not to have a group of rats that were not exposed to opioids and abstinence because our main hypothesis focused on the effect of sleep restriction as a vulnerability during abstinence from opioids. We therefore focused on comparing “During” opioid-abstinence to “Before” opioid-abstinence in a repeated measures design and comparing sleep restriction to an ambulatory control group (described below).
Figure 1. Experimental protocol timeline. Rats were allotted 7 d for recovery from surgery and 8 d for baseline measurements, which included diurnal and restraint stress ACTH and corticosterone. After 10 d of sucrose SA and 10 d of oxycodone SA during a 26-d timespan, rats entered the abstinence phase and were acclimated to the sleep restriction apparatuses. During the next 35 d of abstinence, sleep restriction or ambulation control conditions were carried out. HPA axis assessments were repeated on Days 20–23 of sleep restriction or control conditions. The final nine experimental days of sleep restriction or control conditions were composed of extinction testing, footshock reinstatement, and tissue harvests.
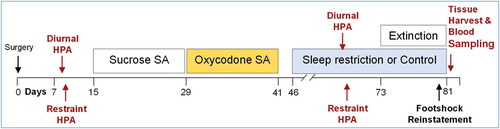
HPA Axis assessment
Diurnal blood samples (1600 h and then 0800 h) were collected during the pre-oxycodone baseline period and during Days 20–23 of the sleep restriction period. The AM sampling time was immediately followed by 30 min of restraint for measurement of stress-induced increases in ACTH and corticosterone at 30, 60, 90, and 150 min after onset of restraint. Blood was sampled by tail clip, which is quick and minimally stressful (Chintamaneni et al., Citation2014; Gehrand et al., Citation2016, Citation2018). Restraint was carried out by placing the rat in a flat-bottomed restrainer (Plas-Labs, Inc., Lansing, MI), modified to accommodate the intrascapular catheter port.
Procedure for producing chronic sleep restriction and ambulation controls
The repeated measures design was constructed so the same rats were studied before and during the abstinence period (after oxycodone self-administration). Rats were each handled for 2 min per day. Rats were allotted 5 days of acclimation in Bergmann–Rechtschaffen apparatuses, which are large open-aired chambers that permit freedom of movement and normal behaviors (Bergmann et al., Citation1989; Everson & Szabo, Citation2009). Rats were assigned to sleep restriction or ambulation control conditions in a counterbalanced manner. Sleep restriction was produced by a validated modification of the Bergmann–Rechtschaffen paradigm in which 6-s rotations of the housing platform produced ambulation requirements at variable intervals incompatible with consolidated sleep; a 35% reduction in sleep amount and high sleep fragmentation (Everson & Szabo, Citation2009). Chronic sleep restriction was produced by repeating a 7-day cycle composed of 5 days of sleep restriction followed by 2 days of sleep ad libitum for 35–37 days. This duration was chosen because it is long enough for subclinical physiological changes to evolve but short enough to preclude morbidity observed later (Everson & Szabo, Citation2009). Ambulation control conditions were the same as for chronic sleep restriction except that the ambulation requirements were consolidated into periods that permitted other periods with more lengthy sleep opportunities. Sleep amounts attained during sleep restriction days vary by a standard deviation of 7% of total time for sleep restricted rats and 4% for ambulation controls (Everson & Szabo, Citation2009). Maintenance of the experimental conditions for sleep restriction and ambulation control was verified repeatedly daily.
Cardiac puncture and tissue harvest
During the final eight days of abstinence, rats were brought back to the self-administration chambers on six of those days for extinction testing (no oxycodone delivered) and to measure foot shock reinstatement of responding after extinction. Within 48 h of testing foot shock reinstatement (randomly between 0800 and 1430), rats were deeply anesthetized (4% isoflurane) and exsanguinated by cardiac puncture and blood was processed for the measurement of plasma copeptin. Necropsy examinations and tissue harvests were performed. Adrenal glands and anterior pituitaries were placed in chilled RNAlater™, cold incubated for 24 h, and then stored at −80 °C until RNA extraction. Whole brains were frozen by immersion in 2-methylbutane on dry ice and stored at −80 °C until RNA extraction.
Measurements
Plasma hormones
Plasma ACTH (MP Biomedicals; Orangeburg, NJ; catalog #07106101, RRID:AB_2783719) and corticosterone (MP Biomedicals; catalog #07120102; RRID:AB_2783720) were measured by direct radioimmunoassays as described and validated previously (Bruder et al., Citation2008). Briefly, the plasma ACTH immunoassay uses a rabbit anti-porcine ACTH antibody and human synthetic ACTH (1–39) as standards and I125- tracer. The assay has 100% crossreactivity with ACTH (1–24) which is shared with the rat ACTH sequence and <1% crossreactivity with other POMC fragments. The intraassay and interassay coefficients of variation (CVs) are 3–6%. The plasma corticosterone immunoassay uses a rabbit anti-corticosterone-BSA antibody and I125-corticosterone tracer. It has <1% crossreactivity with other known endogenous rat corticosteroids. The intra- and interassay CVs range from 4% to 10%. Plasma copeptin was measured by direct competitive ELISA as described and validated previously [(Balapattabi et al., Citation2019); MyBiosource MBS724037, San Diego, CA; RRID:AB_2920579]. This competitive enzyme immunoassay uses a polyclonal rat anti-copeptin antibody and a rat copeptin-HRP conjugate. It does not have significant crossreactivity with copeptin analogues tested. The sensitivity of the assay is 1.0 pg/ml and its intra-and interaassay CVs are <10%. Most importantly, the plasma copeptin assay correlates well with plasma vasopressin levels for which it is a useful clinical surrogate (Balapattabi et al., Citation2019).
mRNA by qPCR
Essential tissue elements of the HPA axis were evaluated by real-time PCR as described previously with minor modifications (Bruder et al., Citation2008; Chintamaneni et al., Citation2013; Gehrand et al., Citation2018, Citation2020). Rat brains were sectioned in the coronal plane in a cryostat at −20 °C until 0.9 mm caudal to bregma was reached. The cryostat blade was warmed with a blow dryer, then a 1100-µm thick section was cut that ended at 2.0 mm caudal to bregma. A punch tool (1.2-mm diameter) was then used to extract the PVN bilaterally. The tissue punch was placed in a tube for homogenizing with 1 mL of QIAzol Lysis Reagent (Qiagen), homogenized for 1 minute and placed in a freezer.
Total RNA from the hypothalamus (PVN), pituitaries, and whole adrenal glands were isolated using the RNeasy Lipid Tissue Mini kit with an on-column DNase digestion (Qiagen, Carlsbad, CA). PCR conditions and methods were performed as described previously (Gehrand et al., Citation2018).
The list of rat genes and corresponding TaqMan hydrolysis probe assays are shown in Supplemental Table 1 the link to which is found in the reference list (Raff, Citation2022a). Rpl19 (Rn00821265_g1) and Actb (Rn00667869_m1) were analyzed as reference genes for each tissue as listed in Supplemental Table 1. Fold-change analysis was calculated by normalizing to ambulatory control with their respective reference gene listed previously using the 2-ΔΔCT method as described elsewhere (Livak & Schmittgen, Citation2001). Quantification cycle values were used to calculate fold-change analysis within each tissue using a standardized algorithm described previously (Gehrand et al., Citation2022).
Table 1. mRNA data not shown in (fold-change from Male AC).
Statistical analysis
A repeated measures ANOVA was fitted to the log-transformed ACTH and square root transformed corticosterone measurements using a mixed effects linear model with a random-effects for animal and fully interacting fixed effects of SR vs AC condition, sex, and abstinence timing (Before vs During). The transformations were selected using Box-Cox analysis. Separate models were fitted for AM and PM measurements. A type III ANOVA table with the Kenward-Roger degrees of freedom calculation was used to evaluate the overall effect of the predictors and their interactions. Targeted post-hoc comparisons of “simple effects,” i.e., levels of one factor within fixed combinations of all other factors were made based on the model, with single-step multivariate t-distribution adjustment for multiple testing within each set of simple contrasts. Because of the large number of contrasts evaluated, only the major comparisons are shown in the figures and described in the text. The contrasts comparing ACTH or corticosterone at all 5 time-points between two groups were combined into a single test of equality during stress responses between the groups using Hotelling’s T-test. The complete ANOVA output for the plasma ACTH and corticosterone data are supplied in Supplemental Table 2 the link to which is found in the reference list (Raff, Citation2022b). These analyses were performed using R 4.0.3. Fold-changes in qPCR mRNA and copeptin data were analyzed by Kruskal-Wallis analysis of variance on ranks and all pairwise multiple comparison procedures (Dunn’s Method) using SigmaPlot 14.5; Systat Software, Inc. P < 0.05 was considered statistically significant and values are reported as mean ± SE.
Results
Adrenal gland and body weights
Body weights after randomization of rats but before treatments were started were not different within sex: Male, AC (411 ± 6 g; N = 16) vs SR (423 ± 8 g; N = 16) (p = 0.25); Female, AC (245 ± 4 g; N = 15); vs. SR (251 ± 3 g; N = 16) (p = 0.24). During opioid abstinence, body weight in sleep restricted males (408 ± 6 g; N = 16) was not different from male ambulatory controls (423 ± 9 g; N = 16; p = 0.066). Body weight in sleep restricted females (257 ± 4 g; N = 16) was not different than female ambulatory controls (250 ± 4 g; N = 15; p = 0.38). Adrenal weight normalized to body weight in sleep restricted males (0.62% ± 0.01%; N = 15) was not different than male ambulatory controls (0.60% ± 0.02%; N = 15; p = 0.16). Adrenal weight normalized to body weight in sleep restricted females (1.11% ± 0.03%; N = 15) was not different than female ambulatory controls (1.11% ± 0.03%; N = 15; p = 0.96).
Diurnal rhythm
We assessed basal AM (∼0800 h) and PM (∼1600 h) plasma levels of ACTH and corticosterone in male and female rats before oxycodone self-administration and then during abstinence under either ambulatory control (normal sleep) or sleep restricted conditions (). Plasma ACTH in the AM and corticosterone in the AM and PM were lower in male compared to female rats subsequently assigned to AC or SR during opioid abstinence. During opioid abstinence, the AM male plasma ACTH and corticosterone had increased regardless of AC vs SR. Although the male plasma ACTH in the AM was no longer different than female plasma ACTH, the plasma corticosterone remained lower. The pattern for plasma ACTH in the PM was similar to the AM. However, although not statistically different, male plasma ACTH was numerically higher in the SR compared to the AC during opioid abstinence (p = 0.076). That said, the plasma ACTH values were quite variable. Even so, when analyzing this specific comparison by t-test, the p value was 0.039. These findings and variability were not reflected in the PM plasma corticosterone findings. As opposed to the AM, opioid abstinence resulted in a decrease in corticosterone in the PM in both sexes regardless of AC vs SR.
Figure 2. Diurnal plasma ACTH (upper panels) and corticosterone (lower panels) in female and male rats Before and then During abstinence from opioids in rats under sleep restricted (SR) conditions vs. ambulatory controls (AC) (Male: N = 15–16 per mean/SE for treatment groups; Female: N = 14–16 per mean/SE for treatment groups). All statistical symbols are p < 0.05. (a) Male different from female within Before or During, (b) During different from Before within sex. For overall ANOVA and all specific comparisons, see the link to Supplementary Table 2 in the reference list (Raff, Citation2022b).
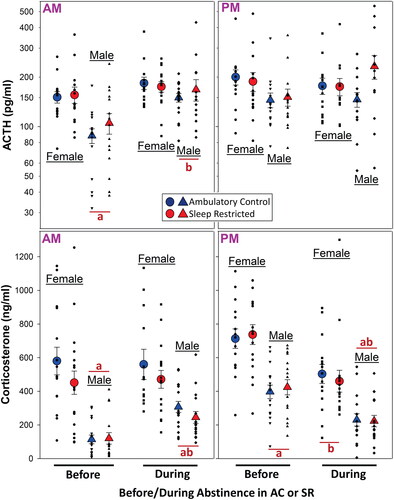
Restraint stress
In order to interrogate HPA axis responsivity, we performed 30 min of standard restraint stress in male and female rats before and then again during abstinence in ambulatory control (AC) and sleep restricted (SR) male and female rats (respectively in ).
Figure 3. Plasma ACTH and corticosterone response to 30 min of restraint in male and female rats Before and then During abstinence from opioids in ambulatory control rats and rats under sleep restricted conditions (N values as in ; mean/SE). All statistical symbols are p < 0.05. Symbol colors are specific to AC (blue) or SR (red) rats. (a) Different from 0 min; (b) above the dashed line indicates an overall difference between AC and SR “During” by Hotelling’s test (p < 0.019); (c) different from “Before” at that time point. c above the dashed line indicates an overall difference from “Before” by Hotelling’s test for AC (p = 0.0027) and SR (p = 0.026) rats. (d) Different from male within same treatment at same time point. For overall ANOVA and all specific comparisons, see link to Supplementary Table 2 in the reference list (Raff, Citation2022b).
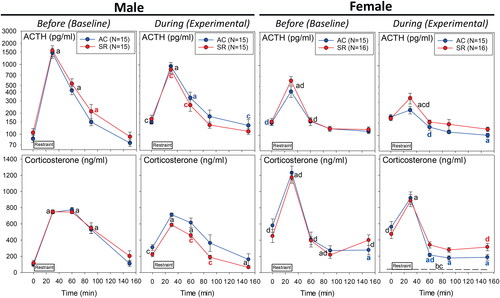
ANOVA interaction effects
The restraint data were analyzed for all interactions. These interactions allowed us to focus in on specific, relevant multiple comparisons annotated in . Because of the large number of interactions, the symbols in focus on main hypotheses proposed and the Results highlighted below. For all of the statistical details about the interactions and multiple comparisons, please see Supplementary Table 2 the link to which is found in the reference list (Raff, Citation2022b).
(left panels) shows basal and the restraint-stimulated plasma ACTH and corticosterone responses in male rats. The rats assigned to the ambulatory control (AC) or sleep restriction (SR) groups showed similar, classic responses to restraint (“Before”—left column). This is expected because both groups were treated identically until oxycodone abstinence. Baseline (0 min) plasma ACTH and corticosterone were increased during abstinence regardless of sleep state. The pattern of the plasma ACTH and corticosterone response to restraint During abstinence was different from Before primarily in the sleep restricted group with higher 0 min levels and smaller responses to restraint. In male rats, there was a tendency for an overall effect of sleep restriction on the plasma corticosterone pattern During abstinence (p = 0.15).
(right panels) shows the basal and restraint-stimulated plasma ACTH and corticosterone responses in female rats. The rats assigned to the ambulatory control (AC) or sleep restriction (SR) groups showed similar, classic responses to restraint (“Before”—left column) without statistically significant group differences. As opposed to males, baseline (0 min) plasma ACTH and corticosterone levels were not altered by opioid abstinence. The plasma ACTH response to restraint at 30 min was attenuated During abstinence compared to Before. Interestingly, the post restraint plasma ACTH (at 150 min) and corticosterone (60–150 min) were below the 0 min in the ambulatory controls (AC), but not sleep restricted (SR) rats. Said another way, sleep restriction prevented the full nadir in plasma ACTH and corticosterone during the recovery from restraint that occurred in abstinent AC female rats. This effect of sleep state on corticosterone in female rats is reflected by the overall ANOVA findings for SR vs AC (p = 0.019).
Male vs female
The details of the multiple comparisons of male vs. female rats are provided in a supplemental file [linked in (Raff, Citation2022b)]. As alluded to above, there were overall differences by ANOVA in the pattern of the plasma ACTH (p < 0.0001) and plasma corticosterone (p < 0.0001) in male vs female rats exposed to restraint stress. There was also a significant interaction between Sex x Abstinence x Sleep Restriction in plasma ACTH (p = 0.034) and plasma corticosterone (p = 0.0003). Female rats before exposure to opioids and sleep disruption had higher baseline plasma ACTH and corticosterone concentrations than males. Furthermore, there was a smaller peak plasma ACTH response but a larger peak corticosterone response to restraint compared to male rats. In fact, the pattern of the HPA response to restraint was different between sexes with the males showing a more prolonged response compared to females.
We also analyzed the restraint stress data by different calculations of the area under the curves for individual rats in responses to restraint. The magnitude of the inter-animal variability in the AUC data precluded any additional statistical findings of significance.
Analysis of mRNAs from the paraventricular nucleus (PVN), pituitary, and adrenal glands
We analyzed the mRNA expression of critical HPA axis-associated genes in the paraventricular nucleus ( and ) and pituitary and adrenal glands obtained at the end of the experiments but while the rats were still under SR (vs. AC) conditions. What was remarkable was the effect of SR that revealed sexual dimorphism of the expression of corticotropin-releasing hormone (CRH) and arginine vasopressin (AVP) mRNAs in the paraventricular nucleus. There was a large increase in both of these critical hypophysiotropic factors in the PVN in male SR rats with the magnitude of the increase in AVP being especially dramatic. The female rats showed no such changes in PVN CRH and AVP. Another noteworthy effect of SR in the PVN was a decrease in Gilz and Per1 mRNAs—markers of glucocorticoid action—which occurred in male rats only (). Although the expression of many other mRNAs in the anterior pituitary and adrenal were lower in females compared to males, there were no effects of SR ().
Figure 4. Paraventricular nucleus mRNAs for corticotropin-releasing factor (CRH) and arginine vasopressin (AVP) from brains obtained at the end of the abstinence/sleep restriction vs. abstinence/ambulatory control periods. Data shown as fold changes (calculations described in Methods section). N = 6 per mean/standard error. All statistical symbols are p < 0.05. (a) Different from AC within sex. (b) different from male within treatment group.
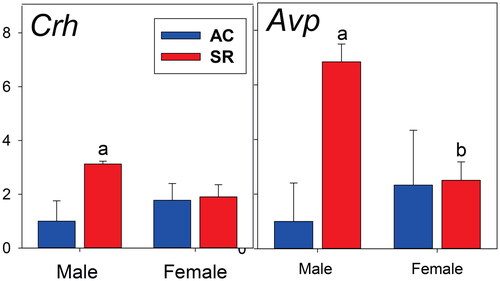
Plasma copeptin
The PVN contains both parvo- and magnocellular vasopressinergic cell bodies and both are hypophysiotropic (Raff, Citation1987, Citation1993; Rivier et al., Citation1984; Rivier & Vale, Citation1983, Citation1983); furthermore, CRH stimulates magnocellular AVP release (Raff et al., Citation1996). Therefore, we measured plasma copeptin which is a marker of magnocellular vasopressin synthesis and release from the posterior pituitary (Balapattabi et al., Citation2020; Bolignano et al., Citation2014; Katan et al., Citation2008). As shown in , the copeptin concentrations mirrored those of AVP mRNA expression in the PVN shown in with an increase in male but not female rats under SR conditions.
Figure 5. Plasma copeptin concentration at the end of the abstinence/sleep restriction vs. abstinence/ambulatory control periods (N = 9 per mean/SE). (a) Different from AC within sex (p < 0.001). (b) Different from male within treatment group (p < 0.001).
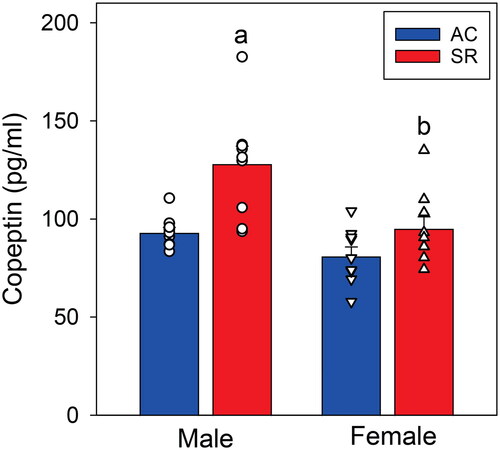
Discussion
The purpose of this study was to evaluate the effects of sleep restriction compared to an ambulatory control condition in rats during abstinence from prior opioid use on the hypothalamic-pituitary-adrenal (HPA) axis. Major findings were (a) compared to before opioid exposure, opioid abstinence resulted in an increase AM plasma ACTH and corticosterone in male but not female rats regardless of sleep state, (b) compared to before opioid exposure, PM plasma corticosterone was lower during opioid abstinence per se in male and female rats, (c) there was a tendency for sleep restriction to increase plasma ACTH in the PM but not corticosterone in male rats during abstinence from opioids, (d) opioid abstinence per se attenuated the plasma ACTH response to restraint in male and female rats compared to before opioid exposure, (e) the corticosterone response to restraint was attenuated in sleep restricted male rats during abstinence compared to before abstinence, (f) sleep restricted female rats did not achieve the full nadir in corticosterone during recovery from restraint, and (g) hypothalamic PVN Crh and Avp mRNA expression was increased and Gilz and Per1 mRNA expression was decreased in male but not female sleep restricted rats during abstinence; this correlated with plasma copeptin concentration.
Diurnal rhythm—non-stressed (basal) findings
A striking finding was the increased plasma ACTH and corticosterone in male but not female rats during abstinence in the AM regardless of sleep state. Furthermore, there was a trend toward an effect of sleep restriction during abstinence to increase unstressed PM plasma ACTH in males but not females. There is a well-known sex difference in basal HPA axis circadian rhythms that may be accentuated by chronic stressors akin to sleep restriction (Goel et al., Citation2014; Seale et al., Citation2004; Spiga et al., Citation2014). At first, we thought the lack of the same pattern in plasma corticosterone in males could be due to a ceiling effect of ACTH stimulation of corticosterone secretion. However, the concentrations of plasma corticosterone in the male rats are not close to maximal as shown by the corticosterone response to acute stress in . That said, there is a diurnal rhythm in adrenal sensitivity in rats that could be involved in this dissociation and thought to be controlled by the underappreciated post-ganglionic neural input to the zona fasciculata of the rat adrenal via the splanchnic nerve (Dallman et al., Citation1978; Engeland, Citation1998; Jasper & Engeland, Citation1994, Citation1997; Ulrich-Lai et al., Citation2006). There are also non-ACTH humoral inputs to the adrenal gland that could be involved, although probably not vasopressin viz the copeptin results (Brooks et al., Citation1988; Raff, Citation1993). Ultimately, independent adrenocortical control may be a way to protect the body against the detrimental effects of glucocorticoids. As an aside, there was a trend for body weight to be lower in sleep restricted males compared to male ambulatory controls. Although not p < 0.05, this could theoretically reflect increases in stress levels in some rats and account for higher variability in plasma ACTH.
This raises two mechanistic issues. The first are the potential factors that could be driving the basal sex difference during opioid abstinence on plasma ACTH in males but not female rats. One possibility is an increase in hypothalamic CRH release as supported by the increase in CRH mRNA in SR males in the PVN () (Seale et al., Citation2004, Citation2004). Furthermore, there was an even more dramatic increase in PVN AVP mRNA in male, but not female, SR rats. Vasopressin is well known as an important hypophysiotropic factor and interacts with CRH in the control of ACTH release (Rivier et al., Citation1984; Rivier & Vale, Citation1983; Schwartz et al., Citation1994). We obviously cannot distinguish between parvocellular and magnocellular AVP mRNA using PVN punches for the analysis (Raff et al., Citation2007). However, we suspect that magnocellular AVP is very likely to be a major input because the plasma copeptin levels obtained from blood when the brains were removed very much paralleled the PVN mRNA signal. Copeptin is a very accurate and validated index of hypothalamic PVN magnocellular vasopressin synthesis and secretion from the posterior pituitary (Balapattabi et al., Citation2020, Citation2021; Katan et al., Citation2008). It is also well known that magnocellular AVP is involved in the control of ACTH secretion via branches of the AVP-secreting axons that terminate on long portal veins in the median eminence as well as via short portal veins from the posterior pituitary to anterior pituitary gland (Raff, Citation1987, Citation1993). Future experiments to resolve this issue could include RNAscope for AVP mRNA in the hypothalamus or spatial molecular imaging of the brain, as well as evaluating the effects of systemically applied AVP receptor antagonists (Schwartz & Reid, Citation1986). It would also be interesting to evaluate chronic water balance in the SR rats as an important endpoint of magnocellular, circulating vasopressin. We plan to carefully evaluate water balance and electrolytes/osmolality in future studies.
Another interesting sexually dimorphic finding (i.e., in males only) was the consistent, large sleep-restriction-induced decrease in the expression of Gilz and Per1 mRNAs in the male hypothalamic PVN during abstinence without a change in Nr3C1 or Nr3c2 (glucocorticoid or mineralocorticoid receptor) mRNAs. This did not occur in the females. The expression of Gilz and Per1 are well known downstream biomarkers of glucocorticoid activity (Ayyar et al., Citation2015; Gehrand et al., Citation2022; George et al., Citation2017). Of relevance to this study of sleep restriction in rats during opioid abstinence is the decrease in PVN Per1 (Period Circadian Regulator 1) mRNA expression. Per1 expression is altered with both sleep restriction (Li et al., Citation2022) and opioid abstinence (Li et al., Citation2009) although we not aware of a study evaluating their interaction. Furthermore, the importance of the sex differences in molecular clock gene expression in the hypothalamus with respect to both diurnal rhythmicity and stress responses has been established (Chun et al., Citation2015, Citation2018). Therefore, it is possible that expression of these glucocorticoid sensitive Gilz and Per1 genes may be involved in the transduction or a reflection of the interactive effects of opioid abstinence and sleep restriction.
Acute restraint stress
In addition to evaluating basal diurnal plasma ACTH and corticosterone, we found that there was a sex difference in the effect of sleep restriction during opioid abstinence on the plasma ACTH and corticosterone response to restraint stress. Sex differences in the HPA axis response to stress ascribed to gonadal steroids are well known (Goel et al., Citation2014; Goel & Bale, Citation2008, Citation2010; Seale et al., Citation2004; Spiga et al., Citation2014). The first intriguing finding was the lack of a statistically significant plasma ACTH response to restraint in ambulatory control (AC) female rats during abstinence despite an increase in plasma corticosterone. As described above for diurnal rhythmicity, this is more evidence that adrenal sensitivity to ACTH could be altered during abstinence (Dallman et al., Citation1978; Engeland, Citation1998; Jasper & Engeland, Citation1994, Citation1997; Ulrich-Lai et al., Citation2006). It is possible that plasma ACTH was increased before 30 min (e.g. at 15 min) in this group. That said, sleep restriction (SR) in females clearly augmented the plasma ACTH response to restraint at 30 min during abstinence compared to ambulatory control. The negligible plasma ACTH response to restraint in female AC rats during abstinence was reflected in the lower nadir plasma corticosterone at 60 minutes and afterwards. In other words, the larger plasma ACTH response in SR rats during abstinence is reflected in a higher nadir in corticosterone at later time points. This is consistent with the ability of the zona fasciculata to integrate a prior ACTH signal (Keller-Wood et al., Citation1983; Spiga et al., Citation2014). That SR female rats did not achieve the full nadir in corticosterone during opioid abstinence is a unique finding worthy of further investigation.
In males, SR during abstinence attenuated the plasma ACTH and corticosterone response to restraint compared to before abstinence and SR. However, there was no difference in the ACTH response to restraint in AC vs SR during abstinence. However, the significant within-group comparison is likely a more sensitive indication of change than a between-group comparison because the same subjects composed each timepoint. It is also possible that abstinence per se affected the HPA axis sufficiently to obscure any subtle effects of SR in male rats in a between-groups comparison.
In comparing males to females, it seems possible that the higher corticosterone response during the recovery period from restraint in abstinent females during sleep restriction is related to the sex difference in susceptibility to relapse (Bakhti-Suroosh et al., Citation2021; Malone et al., Citation2021; Smethells et al., Citation2020) and may cause other negative systemic effects, such as insulin resistance and hypertension. These data are consistent with our previous studies showing a nadir in corticosterone in control female rats during recovery from restraint that is below the 0 min time point (before the onset of restraint) (Gehrand et al., Citation2018). As described above, this sexual dimorphism of the HPA axis response to restraint during SR could be ascribed to changes in gene expression in the hypothalamus, in particular CRH and AVP mRNA.
ACTH vs. corticosterone
There was an apparent quantitative dissociation of plasma ACTH and corticosterone in several findings including the baseline diurnal results () and during restraint (see ). It is well known that the stimulus-induced secretion of endogenous corticosteroids from the adrenal cortex is a better “bioassay” for small changes in circulating plasma ACTH (and therefore hypothalamic control) than plasma ACTH concentrations measured by immunoassay at a limited number of time points (Raff et al., Citation1983; Wood et al., Citation1982, Citation1982). This is partially due to the pulsatile, episodic nature of ACTH secretion (Spiga et al., Citation2014) and that the adrenal cortex amplifies and integrates this plasma ACTH signal (Keller-Wood et al., Citation1983; Spiga et al., Citation2014). There are also several non-ACTH stimulatory inputs to the adrenal zona fasciculata including the splanchnic nerves and humoral signals (Brooks et al., Citation1988; Ehrhart-Bornstein et al., Citation1995; Engeland, Citation1998). These possibilities suggest that there may be an effect of sleep restriction that can bypass the classic descending ACTH input to differentially control the secretion of endogenous corticosteroids like cortisol and corticosterone.
Limitations
The purpose of this study was to investigate the effects of sleep restriction on the HPA axis during opioid abstinence as the first step in evaluating the relevance of sleep restriction as a vulnerability factor in altered HPA axis responses. A limitation of the findings is the unknown extent to which the sleep restriction effects on the HPA axis are unique to opioid abstinence vs. no opioid exposure or to nonopioid substances of misuse. The current findings provide a rationale for future studies of a group not exposed to opioids. Male rats not exposed to opioids and sleep restricted for eight days by different experimental means showed an attenuation of the ACTH response to restraint stress without a difference in the corticosterone response compared to controls (Meerlo et al., Citation2002). Comparing this outcome to the present findings in opioid abstinence in male rats suggests the interaction with opioid abstinence could be unique and highlights the critical points to be emphasized and remembered—sleep restriction interferes with mechanisms of the HPA axis, and it does so in ways that would not be elucidated by studying corticosterone concentrations per se.
Another limitation is an undetermined extent to which sleep restriction by instrumental means interferes with the same mechanisms as endogenously driven sleep disruption in abstinent humans. This limitation is weighed against the benefit of selective sleep restriction in our experimental model that allows for the examination of main effects and mechanistic differences between sexes to better position the field for comparative research and identification of biomarkers and mediators. It should be noted that all rats received isoflurane anesthesia during jugular catheterization and received an infusion of methohexital to confirm catheter patency after oxycodone self-administration. HPA axis measurements were taken at least 8 days following isoflurane exposure, and methohexital infusion was done at least 25 days prior to subsequent outcome measures. Although we cannot exclude the likelihood that one or both procedures impacted the reported outcomes, all subjects were exposed by the same means.
Finally, when we designed these experiments, we chose to do assessments as part of a companion behavioral study that are not reported here including allodynia and/or hyperalgesia responses to mechanical (Deuis et al., Citation2017), cold (Brenner et al., Citation2012), and heat stimuli (Cheah et al., Citation2017), and 10-min exploration in a novel environment (Schmitt & Hiemke, Citation1998). These noninvasive measurements without physical restraint were conducted during the pre-oxycodone and the abstinence phases of the study and were separated from blood drawing or extinction testing by at least 24 h. We realize that these procedures represent potential additional stressors that could account for the higher plasma ACTH and corticosterone in male rats during abstinence (see and ). That the Ambulatory Control plasma ACTH and corticosterone concentrations were not different Before and During abstinence in females suggests that this may not be a major effect although it could have affected the response to restraint. A final phase of the behavioral assessments involved footshock reinstatement of oxycodone seeking, a procedure where rats were placed into the drug self-administration chambers and exposed to footshock (0.5 mA, 0.5 s duration for 15 min under a 40-s variable interval (VI-40) schedule). This occurred ∼48 hours prior to tissue collection, which could have influenced the brain mRNA expression. However, all comparisons are between groups receiving the same treatments and the same environmental conditions.
We plan to report the results of these extensive behavioral assessments in a subsequent publication as they generated a very large data set.
Perspectives: An abnormal response to stress, and the effects of drugs on the HPA axis that mediate its reactivity to stress, have been proposed as a biological basis for substance misuse (Piazza & Le Moal, Citation1996; Shi et al., Citation2009; Sinha et al., Citation2003; Zhou & Leri, Citation2016). The female HPA axis responds differently than the male HPA axis to acute and chronic stressors and to drugs of abuse, including opioids (Becker et al., Citation2017; Becker & Koob, Citation2016; Carroll & Lynch, Citation2016; Figueira & Ouakinin, Citation2010; Laviola et al., Citation2002; Ordaz & Luna, Citation2012). Compared with men, women are believed to possess more highly reactive sleep systems in which they experience drastic deterioration of sleep when stressed (Kalmbach et al., 2018, Citation2018), which the present results indicate could further modify the stress response. In the present studies, mechanistic differences between sexes during opioid abstinence were exposed by sleep restriction. The results of the interaction of stress and sleep restriction modeled in the current study is broadly consistent with sexually dimorphic phenomenon observed in humans. The next step will be to determine if modifications of HPA axis function by sleep restriction are associated with drug seeking, which could lead to feasible low risk pharmacological interventions in humans. Finally, the dramatic effects on hypothalamic vasopressin mRNA expression and plasma copeptin may redirect our focus to the peripheral interactions of vasopressin, ACTH, and corticosterone in our model of opioid abstinence and sleep restriction.
Supplemental Material
Download Zip (206 KB)Acknowledgements
This report is in honor of the memory of Mary Dallman with whom one of us (HR) trained. We thank the contributors to this study: Rachel Jackson, Madison Kruk, and Nicholas Pucek (animal experimentation and preliminary data analyses), Jonathan Phillips (hormone assays and brain processing), Ashley Gehrand (PCR), and William Cullinan (brain processing).
Disclosure statement
Hershel Raff is a Consultant for Corcept Therapeutics and Cerium Pharmaceuticals. The other authors have nothing to declare.
Data availability statement
All data generated or analyzed during this study are included in this published article or in the supplementary files in the data repository cited in the references.
Additional information
Funding
References
- Aouizerate, B., Ho, A., Schluger, J. H., Perret, G., Borg, L., Le Moal, M., Piazza, P. V., & Kreek, M. J. (2006). Glucocorticoid negative feedback in methadone-maintained former heroin addicts with ongoing cocaine dependence: Dose-response to dexamethasone suppression. Addiction Biology, 11(1), 84–96. https://doi.org/10.1111/j.1369-1600.2006.00006.x
- Ayyar, V. S., Almon, R. R., Jusko, W. J., & DuBois, D. C. (2015). Quantitative tissue-specific dynamics of in vivo GILZ mRNA expression and regulation by endogenous and exogenous glucocorticoids. Physiological Reports, 3(6), e12382. https://doi.org/10.14814/phy2.12382
- Bakhti-Suroosh, A., Towers, E. B., & Lynch, W. J. (2021). A buprenorphine-validated rat model of opioid use disorder optimized to study sex differences in vulnerability to relapse. Psychopharmacology, 238(4), 1029–1046. https://doi.org/10.1007/s00213-020-05750-2
- Balapattabi, K., Farmer, G. E., Knapp, B. A., Little, J. T., Bachelor, M., Yuan, J. P., & Cunningham, J. T. (2019). Effects of salt-loading on supraoptic vasopressin neurones assessed by ClopHensorN chloride imaging. Journal of Neuroendocrinology, 31(8), e12752. https://doi.org/10.1111/jne.12752
- Balapattabi, K., Little, J. T., Bachelor, M. E., Cunningham, R. L., & Cunningham, J. T. (2021). Sex differences in the regulation of vasopressin and oxytocin secretion in bile duct-ligated rats. Neuroendocrinology, 111(3), 237–248. https://doi.org/10.1159/000508104
- Balapattabi, K., Little, J. T., Bachelor, M., & Cunningham, J. T. (2020). Brain-derived neurotrophic factor and supraoptic vasopressin neurons in hyponatremia. Neuroendocrinology, 110(7–8), 630–641. https://doi.org/10.1159/000503723
- Becker, J. B., & Chartoff, E. (2019). Sex differences in neural mechanisms mediating reward and addiction. Neuropsychopharmacology, 44(1), 166–183. https://doi.org/10.1038/s41386-018-0125-6
- Becker, J. B., & Koob, G. F. (2016). Sex differences in animal models: Focus on addiction. Pharmacological Reviews, 68(2), 242–263. https://doi.org/10.1124/pr.115.011163
- Becker, J. B., McClellan, M. L., & Reed, B. G. (2017). Sex differences, gender and addiction. Journal of Neuroscience Research, 95(1–2), 136–147. https://doi.org/10.1002/jnr.23963
- Bergmann, B. M., Kushida, C. A., Everson, C. A., Gilliland, M. A., Obermeyer, W., & Rechtschaffen, A. (1989). Sleep deprivation in the rat: II. Methodology. Sleep, 12(1), 5–12. https://doi.org/10.1093/sleep/12.1.5
- Beswick, T., Best, D., Rees, S., Bearn, J., Gossop, M., & Strang, J. (2003). Major disruptions of sleep during treatment of the opiate withdrawal syndrome: Differences between methadone and lofexidine detoxification treatments. Addiction Biology, 8(1), 49–57. https://doi.org/10.1080/1355621031000069882
- Bolignano, D., Cabassi, A., Fiaccadori, E., Ghigo, E., Pasquali, R., Peracino, A., Peri, A., Plebani, M., Santoro, A., Settanni, F., & Zoccali, C. (2014). Copeptin (CTproAVP), a new tool for understanding the role of vasopressin in pathophysiology. Clinical Chemistry and Laboratory Medicine, 52(10), 1447–1456.
- Brenner, D. S., Golden, J. P., & Gereau, R. (2012). A novel behavioral assay for measuring cold sensation in mice. PLoS One, 7(6), e39765. https://doi.org/10.1371/journal.pone.0039765
- Brooks, V. L., Blakemore, L. J., & Keil, L. C. (1988). Intravenous vasopressin infusion decreases plasma ACTH concentration in conscious dogs. The American Journal of Physiology, 255(4 Pt 2), R665–71. https://doi.org/10.1152/ajpregu.1988.255.4.R665
- Bruder, E. D., Taylor, J. K., Kamer, K. J., & Raff, H. (2008). Development of the ACTH and corticosterone response to acute hypoxia in the neonatal rat. American Journal of Physiology. Regulatory, Integrative and Comparative Physiology, 295(4), R1195–R1203. https://doi.org/10.1152/ajpregu.90400.2008
- Bunce, S. C., Harris, J. D., Bixler, E. O., Taylor, M., Muelly, E., Deneke, E., Thompson, K. W., & Meyer, R. E. (2015). Possible evidence for re-regulation of HPA axis and brain reward systems over time in treatment in prescription opioid-dependent patients. Journal of Addiction Medicine, 9(1), 53–60. https://doi.org/10.1097/ADM.0000000000000087
- Burke, C. K., Peirce, J. M., Kidorf, M. S., Neubauer, D., Punjabi, N. M., Stoller, K. B., Hursh, S., & Brooner, R. K. (2008). Sleep problems reported by patients entering opioid agonist treatment. Journal of Substance Abuse Treatment, 35(3), 328–333. https://doi.org/10.1016/j.jsat.2007.10.003
- Carroll, M. E., & Lynch, W. J. (2016). How to study sex differences in addiction using animal models. Addiction Biology, 21(5), 1007–1029. https://doi.org/10.1111/adb.12400
- Cheah, M., Fawcett, J. W., & Andrews, M. R. (2017). Assessment of thermal pain sensation in rats and mice using the Hargreaves Test. Bio Protocol, 7(16), e2506.
- Chintamaneni, K., Bruder, E. D., & Raff, H. (2013). Effects of age on ACTH, corticosterone, glucose, insulin, and mRNA levels during intermittent hypoxia in the neonatal rat. American Journal of Physiology. Regulatory, Integrative and Comparative Physiology, 304(9), R782–R789. https://doi.org/10.1152/ajpregu.00073.2013
- Chintamaneni, K., Bruder, E. D., & Raff, H. (2014). Programming of the hypothalamic-pituitary-adrenal axis by neonatal intermittent hypoxia: Effects on adult male ACTH and corticosterone responses are stress specific. Endocrinology, 155(5), 1763–1770. https://doi.org/10.1210/en.2013-1736
- Chun, L. E., Christensen, J., Woodruff, E. R., Morton, S. J., Hinds, L. R., & Spencer, R. L. (2018). Adrenal-dependent and -independent stress-induced Per1 mRNA in hypothalamic paraventricular nucleus and prefrontal cortex of male and female rats. Stress, 21(1), 69–83. https://doi.org/10.1080/10253890.2017.1404571
- Chun, L. E., Woodruff, E. R., Morton, S., Hinds, L. R., & Spencer, R. L. (2015). Variations in phase and amplitude of rhythmic clock gene expression across prefrontal cortex, hippocampus, amygdala, and hypothalamic paraventricular and suprachiasmatic nuclei of male and female rats. Journal of Biological Rhythms, 30(5), 417–436. https://doi.org/10.1177/0748730415598608
- Coffey, A. A., Guan, Z., Grigson, P. S., & Fang, J. (2016). Reversal of the sleep-wake cycle by heroin self-administration in rats. Brain Research Bulletin, 123, 33–46. https://doi.org/10.1016/j.brainresbull.2015.09.008
- Dallman, M. F., Engeland, W. C., Rose, J. C., Wilkinson, C. W., Shinsako, J., & Siedenburg, F. (1978). Nycthemeral rhythm in adrenal responsiveness to ACTH. The American Journal of Physiology, 235(5), R210–8. https://doi.org/10.1152/ajpregu.1978.235.5.R210
- Deuis, J. R., Dvorakova, L. S., & Vetter, I. (2017). Methods used to evaluate pain behaviors in rodents. Frontiers in Molecular Neuroscience. 10, 284.
- Dunn, K. E., Finan, P. H., Andrew Tompkins, D., & Strain, E. C. (2018). Frequency and correlates of sleep disturbance in methadone and buprenorphine-maintained patients. Addictive Behaviors, 76, 8–14. https://doi.org/10.1016/j.addbeh.2017.07.016
- Ehrhart-Bornstein, M., Bornstein, S. R., Gonzalez-Hernandez, J., Holst, J. J., Waterman, M. R., & Scherbaum, W. A. (1995). Sympathoadrenal regulation of adrenocortical steroidogenesis. Endocrine Research, 21(1–2), 13–24. https://doi.org/10.3109/07435809509030417
- Engeland, W. C. (1998). Functional innervation of the adrenal cortex by the splanchnic nerve. Hormone and Metabolic Research = Hormon- Und Stoffwechselforschung = Hormones et Metabolisme, 30(6–7), 311–314. https://doi.org/10.1055/s-2007-978890
- Everson, C. A., & Szabo, A. (2009). Recurrent restriction of sleep and inadequate recuperation induce both adaptive changes and pathological outcomes. American Journal of Physiology. Regulatory, Integrative and Comparative Physiology, 297(5), R1430–40. https://doi.org/10.1152/ajpregu.00230.2009
- Fattore, L., Altea, S., & Fratta, W. (2008). Sex differences in drug addiction: A review of animal and human studies. Women’s Health, 4, 51–65. https://doi.org/10.2217/17455057.4.1.51
- Figueira, M. L., & Ouakinin, S. (2010). Gender-related endocrinological dysfunction and mental disorders. Current Opinion in Psychiatry, 23(4), 369–372. https://doi.org/10.1097/YCO.0b013e3283399b86
- Frers, A., Shaffer, J., Edinger, J., & Wachholtz, A. (2021). The relationship between sleep and opioids in chronic pain patients. Journal of Behavioral Medicine, 44(3), 412–420. https://doi.org/10.1007/s10865-021-00205-1
- Gehrand, A. L., Hoeynck, B., Jablonski, M., Leonovicz, C., Cullinan, W. E., & Raff, H. (2018). Programming of the adult HPA axis after neonatal separation and environmental stress in male and female rats. Endocrinology, 159(7), 2777–2789. https://doi.org/10.1210/en.2018-00370
- Gehrand, A. L., Hoeynck, B., Jablonski, M., Leonovicz, C., Ye, R., Scherer, P. E., & Raff, H. (2016). Sex differences in adult rat insulin and glucose responses to arginine: Programming effects of neonatal separation, hypoxia, and hypothermia. Physiological Reports, 4(18), e12972. https://doi.org/10.14814/phy2.12972
- Gehrand, A. L., Phillips, J., Malott, K., & Raff, H. (2020). Corticosterone, adrenal, and the pituitary-gonadal axis in neonatal rats: Effect of maternal separation and hypoxia. Endocrinology, 161(7), 1–14. https://doi.org/10.1210/endocr/bqaa085
- Gehrand, A. L., Phillips, J., Welhouse, K. D., Siddiqui, H., Schulgit, M., Hoffman, J., Hunt, H., & Raff, H. (2022). Glucocorticoid receptor antagonist alters corticosterone and receptor-sensitive mRNAs in the hypoxic neonatal rat. Endocrinology, 163(1), bqab232. https://doi.org/10.1210/endocr/bqab232
- George, C. L., Birnie, M. T., Flynn, B. P., Kershaw, Y. M., Lightman, S. L., & Conway-Campbell, B. L. (2017). Ultradian glucocorticoid exposure directs gene-dependent and tissue-specific mRNA expression patterns in vivo. Molecular and Cellular Endocrinology, 439, 46–53. https://doi.org/10.1016/j.mce.2016.10.019
- Gillin, J. C. (1998). Are sleep disturbances risk factors for anxiety, depressive and addictive disorders? Acta Psychiatrica Scandinavica. Supplementum, 393, 39–43. https://doi.org/10.1111/j.1600-0447.1998.tb05965.x
- Goel, N., & Bale, T. L. (2008). Organizational and activational effects of testosterone on masculinization of female physiological and behavioral stress responses. Endocrinology, 149(12), 6399–6405. https://doi.org/10.1210/en.2008-0433
- Goel, N., & Bale, T. L. (2010). Sex differences in the serotonergic influence on the hypothalamic-pituitary-adrenal stress axis. Endocrinology, 151(4), 1784–1794. https://doi.org/10.1210/en.2009-1180
- Goel, N., Innala, L., & Viau, V. (2014). Sex differences in serotonin (5-HT) 1A receptor regulation of HPA axis and dorsal raphe responses to acute restraint. Psychoneuroendocrinology, 40, 232–241. https://doi.org/10.1016/j.psyneuen.2013.11.020
- Goel, N., Workman, J. L., Lee, T. T., Innala, L., & Viau, V. (2014). Sex differences in the HPA axis. Comprehensive Physiology, 4(3), 1121–1155.
- Greenwald, M. K., Moses, T. E. H., & Roehrs, T. A. (2021). At the intersection of sleep deficiency and opioid use: Mechanisms and therapeutic opportunities. Translational Research, 234, 58–73. https://doi.org/10.1016/j.trsl.2021.03.006
- Hartwell, E. E., Pfeifer, J. G., McCauley, J. L., Moran-Santa Maria, M., & Back, S. E. (2014). Sleep disturbances and pain among individuals with prescription opioid dependence. Addictive Behaviors, 39(10), 1537–1542. https://doi.org/10.1016/j.addbeh.2014.05.025
- Hasler, B. P., Smith, L. J., Cousins, J. C., & Bootzin, R. R. (2012). Circadian rhythms, sleep, and substance abuse. Sleep Medicine Reviews, 16(1), 67–81. https://doi.org/10.1016/j.smrv.2011.03.004
- Hatzinger, M., Kocher, R., Hemmeter, U., Ladewig, D., & Holsboer-Trachsler, E. (1995). Sleep and addiction. Praxis, 84(15), 450–453. [
- Heck, A. L., & Handa, R. J. (2019). Sex differences in the hypothalamic-pituitary-adrenal axis’ response to stress: An important role for gonadal hormones. Neuropsychopharmacology, 44(1), 45–58. https://doi.org/10.1038/s41386-018-0167-9
- Howe, R. C., Hegge, F. W., & Phillips, J. L. (1980). Acute heroin abstinence in man: I. Changes in behavior and sleep. Drug and Alcohol Dependence, 5(5), 341–356. https://doi.org/10.1016/0376-8716(80)90160-x
- Howe, R. C., Hegge, F. W., & Phillips, J. L. (1980). Acute heroin abstinence in man: II. Alterations in rapid eye movement (REM) sleep. Drug and Alcohol Dependence, 6(3), 149–161. https://doi.org/10.1016/0376-8716(80)90454-8
- Howe, R. C., Phillips, J. L., & Hegge, F. W. (1980). Acute heroin abstinence in man. III. Effect upon waking and slow wave sleep. Drug and Alcohol Dependence, 6(4), 247–262. https://doi.org/10.1016/0376-8716(80)90329-4
- Humphreys, K., Shover, C. L., Andrews, C. M., Bohnert, A. S. B., Brandeau, M. L., Caulkins, J. P., Chen, J. H., Cuéllar, M.-F., Hurd, Y. L., Juurlink, D. N., Koh, H. K., Krebs, E. E., Lembke, A., Mackey, S. C., Larrimore Ouellette, L., Suffoletto, B., & Timko, C. (2022). Responding to the opioid crisis in North America and beyond: Recommendations of the Stanford-Lancet Commission. Lancet, 399(10324), 555–604. https://doi.org/10.1016/S0140-6736(21)02252-2
- Ivers, J.-H., Zgaga, L., Sweeney, B., Keenan, E., Darker, C., Smyth, B. P., & Barry, J. (2018). A naturalistic longitudinal analysis of post-detoxification outcomes in opioid-dependent patients. Drug and Alcohol Review, 37(Suppl 1), S339–S347. https://doi.org/10.1111/dar.12597
- Jasper, M. S., & Engeland, W. C. (1994). Splanchnic neural activity modulates ultradian and circadian rhythms in adrenocortical secretion in awake rats. Neuroendocrinology, 59(2), 97–109. https://doi.org/10.1159/000126645
- Jasper, M. S., & Engeland, W. C. (1997). Splanchnicotomy increases adrenal sensitivity to ACTH in nonstressed rats. The American Journal of Physiology, 273(2 Pt 1), E363–E368. https://doi.org/10.1152/ajpendo.1997.273.2.E363
- Kalmbach, D. A., Anderson, J. R., & Drake, C. L. (2018). The impact of stress on sleep: Pathogenic sleep reactivity as a vulnerability to insomnia and circadian disorders. Journal of Sleep Research, 27(6), e12710. https://doi.org/10.1111/jsr.12710
- Kalmbach, D. A., Cuamatzi-Castelan, A. S., Tonnu, C. V., Tran, K. M., Anderson, J. R., Roth, T., & Drake, C. L. (2018). Hyperarousal and sleep reactivity in insomnia: Current insights. Nature and Science of Sleep, 10, 193–201. https://doi.org/10.2147/NSS.S138823
- Kaplan, K. A., McQuaid, J., Primich, C., & Rosenlicht, N. (2014). An evidence-based review of insomnia treatment in early recovery. Journal of Addiction Medicine, 8(6), 389–394. https://doi.org/10.1097/ADM.0000000000000052
- Katan, M., Morgenthaler, N., Widmer, I., Puder, J. J., Konig, C., Muller, B., & Christ-Crain, M. (2008). Copeptin, a stable peptide derived from the vasopressin precursor, correlates with the individual stress level. Neuro Endocrinology Letters, 29(3), 341–346.
- Kay, D. C. (1975). Human sleep and EEG through a cycle of methadone dependence. Electroencephalography and Clinical Neurophysiology, 38(1), 35–43. https://doi.org/10.1016/0013-4694(75)90208-4
- Keller-Wood, M. E., Shinsako, J., & Dallman, M. F. (1983). Integral as well as proportional adrenal responses to ACTH. The American Journal of Physiology, 245(1), R53–9. https://doi.org/10.1152/ajpregu.1983.245.1.R53
- Kosten, T. A., & Ambrosio, E. (2002). HPA axis function and drug addictive behaviors: Insights from studies with Lewis and Fischer 344 inbred rats. Psychoneuroendocrinology, 27(1–2), 35–69. https://doi.org/10.1016/s0306-4530(01)00035-x
- Kreek, M. J. (2000). Methadone-related opioid agonist pharmacotherapy for heroin addiction. History, recent molecular and neurochemical research and future in mainstream medicine. Annals of the New York Academy of Sciences, 909, 186–216. https://doi.org/10.1111/j.1749-6632.2000.tb06683.x
- Kreek, M. J., & Koob, G. F. (1998). Drug dependence: Stress and dysregulation of brain reward pathways. Drug and Alcohol Dependence, 51(1-2), 23–47. https://doi.org/10.1016/s0376-8716(98)00064-7
- Laviola, G., Adriani, W., Morley-Fletcher, S., & Terranova, M. L. (2002). Peculiar response of adolescent mice to acute and chronic stress and to amphetamine: Evidence of sex differences. Behavioural Brain Research, 130(1–2), 117–125. https://doi.org/10.1016/s0166-4328(01)00420-x
- Li, S-x., Shi, J., Epstein, D. H., Wang, X., Zhang, X-l., Bao, Y-p., Zhang, D., Zhang, X-y., Kosten, T. R., & Lu, L. (2009). Circadian alteration in neurobiology during 30 days of abstinence in heroin users. Biological Psychiatry, 65(10), 905–912. https://doi.org/10.1016/j.biopsych.2008.11.025
- Li, W., Wang, Z., Cao, J., Dong, Y., & Chen, Y. (2022). Role of sleep restriction in daily rhythms of expression of hypothalamic core clock genes in mice. Current Issues in Molecular Biology, 44(2), 609–625. https://doi.org/10.3390/cimb44020042
- Lim, T. Y., Stringfellow, E. J., Stafford, C. A., DiGennaro, C., Homer, J. B., Wakeland, W., Eggers, S. L., Kazemi, R., Glos, L., Ewing, E. G., Bannister, C. B., Humphreys, K., Throckmorton, D. C., & Jalali, M. S. (2022). Modeling the evolution of the US opioid crisis for national policy development. Proceedings of the National Academy of Sciences of the United States of America, 119(23), e2115714119.
- Livak, K. J., & Schmittgen, T. D. (2001). Analysis of relative gene expression data using real-time quantitative PCR and the 2(-Delta Delta C(T)) method. Methods, 25(4), 402–408. https://doi.org/10.1006/meth.2001.1262
- Malone, S. G., Keller, P. S., Hammerslag, L. R., & Bardo, M. T. (2021). Escalation and reinstatement of fentanyl self-administration in male and female rats. Psychopharmacology, 238(8), 2261–2273. https://doi.org/10.1007/s00213-021-05850-7
- Maulik, P. K., Tripathi, B. M., & Pal, H. R. (2002). Coping behaviors and relapse precipitants in opioid dependence: A study from North India. Journal of Substance Abuse Treatment, 22(3), 135–140. https://doi.org/10.1016/s0740-5472(02)00225-8
- Meerlo, P., Koehl, M., van der Borght, K., & Turek, F. W. (2002). Sleep restriction alters the hypothalamic-pituitary-adrenal response to stress. Journal of Neuroendocrinology, 14(5), 397–402. https://doi.org/10.1046/j.0007-1331.2002.00790.x
- Mehtry, V., Nizamie, S. H., Parvez, N., & Pradhan, N. (2014). Sleep profile in opioid dependence: A polysomnographic case-control study. Journal of Clinical Neurophysiology, 31(6), 517–522. https://doi.org/10.1097/WNP.0000000000000117
- Muelbl, M. J., Glaeser, B. L., Shah, A. S., Chiariello, R. A., Nawarawong, N. N., Stemper, B. D., Budde, M. D., & Olsen, C. M. (2022). Repeated blast mild traumatic brain injury and oxycodone self-administration produce interactive effects on neuroimaging outcomes. Addiction Biology. 27(2), e13134.
- Mukherjee, D., Stankoski, D. M., Tilden, S. E., Huhn, A. S., Bixler, E. O., Kong, L., Meyer, R. E., Deneke, E., Freet, C. S., & Bunce, S. C. (2021). Reregulation of cortisol levels and sleep in patients with prescription opioid use disorder during long-term residential treatment. Drug and Alcohol Dependence, 227, 108931. https://doi.org/10.1016/j.drugalcdep.2021.108931
- Nawarawong, N. N., Slaker, M., Muelbl, M., Shah, A. S., Chiariello, R., Nelson, L. D., Budde, M. D., Stemper, B. D., & Olsen, C. M. (2019). Repeated blast model of mild traumatic brain injury alters oxycodone self-administration and drug seeking. The European Journal of Neuroscience, 50(3), 2101–2112. https://doi.org/10.1111/ejn.14281
- Ordaz, S., & Luna, B. (2012). Sex differences in physiological reactivity to acute psychosocial stress in adolescence. Psychoneuroendocrinology, 37(8), 1135–1157. https://doi.org/10.1016/j.psyneuen.2012.01.002
- Oyola, M. G., & Handa, R. J. (2017). Hypothalamic-pituitary-adrenal and hypothalamic-pituitary-gonadal axes: Sex differences in regulation of stress responsivity. Stress (Amsterdam, Netherlands), 20(5), 476–494. https://doi.org/10.1080/10253890.2017.1369523
- Parvaresh, N., Sabahi, A. R., Mazhari, S., & Gilani, H. (2015). A study of the sexual function, sleep, and weight status of patients after 6 months of methadone maintenance treatment. Addict Health, 7(1–2), 24–29.
- Peles, E., Schreiber, S., & Adelson, M. (2006). Variables associated with perceived sleep disorders in methadone maintenance treatment (MMT) patients. Drug and Alcohol Dependence, 82(2), 103–110. https://doi.org/10.1016/j.drugalcdep.2005.08.011
- Piazza, P. V., & Le Moal, M. L. (1996). Pathophysiological basis of vulnerability to drug abuse: Role of an interaction between stress, glucocorticoids, and dopaminergic neurons. Annual Review of Pharmacology and Toxicology, 36, 359–378. https://doi.org/10.1146/annurev.pa.36.040196.002043
- Raff, H. (1987). Glucocorticoid inhibition of neurohypophysial vasopressin secretion. The American Journal of Physiology, 252(4 Pt 2), R635–44. https://doi.org/10.1152/ajpregu.1987.252.4.R635
- Raff, H. (1993). Interactions between neurohypophysial hormones and the ACTH-adrenocortical axis. Annals of the New York Academy of Sciences, 689, 411–425. https://doi.org/10.1111/j.1749-6632.1993.tb55564.x
- Raff, H. (2022a). Supplemental Table 1. List of rat genes and corresponding TaqMan hydrolysis probe assays. Deposited 6-24-2022 Figshare. https://figshare.com/articles/figure/Table_Rat_Genes_and_TaqMan_IDs_PDF/20137538
- Raff, H. (2022b). Supplementary_Table 2_Statistics_PDF.pdf. Deposited 11-25-2022 FigShare2022. https://figshare.com/articles/dataset/Raff_Opioid_Restraint_ANOVA_Supplementary_Table_2/21623355
- Raff, H., Jacobson, L., & Cullinan, W. E. (2007). Augmented hypothalamic corticotrophin-releasing hormone mRNA and corticosterone responses to stress in adult rats exposed to perinatal hypoxia. Journal of Neuroendocrinology, 19(11), 907–912. https://doi.org/10.1111/j.1365-2826.2007.01595.x
- Raff, H., Papanek, P. E., & Cowles, V. E. (1996). Vasopressin responses to corticotropin-releasing factor and hypertonicity after truncal vagotomy in dogs. The American Journal of Physiology, 270(1 Pt 2), R94–R98. https://doi.org/10.1152/ajpregu.1996.270.1.R94
- Raff, H., Shinsako, J., & Dallman, M. F. (1983). Surgery potentiates adrenocortical responses to hypoxia in dogs. Proceedings of the Society for Experimental Biology and Medicine, 172(4), 400–406. https://doi.org/10.3181/00379727-172-41578
- Raj, H., Ray, R., & Prakash, B. (2000). Relapse precipitants in opiate addiction: Assessment in community treatment setting. Indian Journal of Psychiatry, 42(3), 253–257.
- Rao, I. J., Humphreys, K., & Brandeau, M. L. (2021). Effectiveness of policies for addressing the US opioid epidemic: A model-based analysis from the Stanford-Lancet Commission on the North American Opioid Crisis. Lancet Regional Health Americas, 3, 100031.
- Rivier, C., & Vale, W. (1983). Interaction of corticotropin-releasing factor and arginine vasopressin on adrenocorticotropin secretion in vivo. Endocrinology, 113(3), 939–942. https://doi.org/10.1210/endo-113-3-939
- Rivier, C., & Vale, W. (1983). Modulation of stress-induced ACTH release by corticotropin-releasing factor, catecholamines and vasopressin. Nature, 305(5932), 325–327. https://doi.org/10.1038/305325a0
- Rivier, C., Rivier, J., Mormede, P., & Vale, W. (1984). Studies of the nature of the interaction between vasopressin and corticotropin-releasing factor on adrenocorticotropin release in the rat. Endocrinology, 115(3), 882–886. https://doi.org/10.1210/endo-115-3-882
- Roth, T., & Workshop, P. (2009). Does effective management of sleep disorders reduce substance dependence? Drugs, 69(Suppl 2), 65–75.
- Sarnyai, Z. (1998). Neurobiology of stress and cocaine addiction. Studies on corticotropin-releasing factor in rats, monkeys, and humans. Annals of the New York Academy of Sciences, 851, 371–387. https://doi.org/10.1111/j.1749-6632.1998.tb09011.x
- Sarnyai, Z., Shaham, Y., & Heinrichs, S. C. (2001). The role of corticotropin-releasing factor in drug addiction. Pharmacological Reviews, 53(2), 209–243.
- Schmitt, U., & Hiemke, C. (1998). Strain differences in open-field and elevated plus-maze behavior of rats without and with pretest handling. Pharmacology, Biochemistry, and Behavior, 59(4), 807–811. https://doi.org/10.1016/s0091-3057(97)00502-9
- Schwartz, J., & Reid, I. A. (1986). Characteristics of the receptors which mediate the stimulation of ACTH secretion by vasopressin in conscious dogs. Neuroendocrinology, 42(2), 93–96. https://doi.org/10.1159/000124256
- Schwartz, J., Ash, P., Ford, V., Raff, H., Crosby, S., & White, A. (1994). Secretion of adrenocorticotrophin (ACTH) and ACTH precursors in ovine anterior pituitary cells: Actions of corticotrophin-releasing hormone, arginine vasopressin and glucocorticoids. The Journal of Endocrinology, 140(2), 189–195. https://doi.org/10.1677/joe.0.1400189
- Seale, J. V., Wood, S. A., Atkinson, H. C., Bate, E., Lightman, S. L., Ingram, C. D., Jessop, D. S., & Harbuz, M. S. (2004). Gonadectomy reverses the sexually diergic patterns of circadian and stress-induced hypothalamic-pituitary-adrenal axis activity in male and female rats. Journal of Neuroendocrinology, 16(6), 516–524. https://doi.org/10.1111/j.1365-2826.2004.01195.x
- Seale, J. V., Wood, S. A., Atkinson, H. C., Harbuz, M. S., & Lightman, S. L. (2004). Gonadal steroid replacement reverses gonadectomy-induced changes in the corticosterone pulse profile and stress-induced hypothalamic-pituitary-adrenal axis activity of male and female rats. Journal of Neuroendocrinology, 16(12), 989–998. https://doi.org/10.1111/j.1365-2826.2004.01258.x
- Shi, J., Li, S-x., Zhang, X-l., Wang, X., Le Foll, B., Zhang, X.-Y., Kosten, T. R., & Lu, L. (2009). Time-dependent neuroendocrine alterations and drug craving during the first month of abstinence in heroin addicts. The American Journal of Drug and Alcohol Abuse, 35(5), 267–272. https://doi.org/10.1080/00952990902933878
- Sinha, R., Talih, M., Malison, R., Cooney, N., Anderson, G. M., & Kreek, M. J. (2003). Hypothalamic-pituitary-adrenal axis and sympatho-adreno-medullary responses during stress-induced and drug cue-induced cocaine craving states. Psychopharmacology, 170(1), 62–72. https://doi.org/10.1007/s00213-003-1525-8
- Smethells, J. R., Greer, A., Dougen, B., & Carroll, M. E. (2020). Effects of voluntary exercise and sex on multiply-triggered heroin reinstatement in male and female rats. Psychopharmacology, 237(2), 453–463. https://doi.org/10.1007/s00213-019-05381-2
- Spiga, F., Walker, J. J., Terry, J. R., & Lightman, S. L. (2014). HPA axis-rhythms. Comprehensive Physiology, 4(3), 1273–1298.
- Teplin, D., Raz, B., Daiter, J., Varenbut, M., & Tyrrell, M. (2006). Screening for substance use patterns among patients referred for a variety of sleep complaints. The American Journal of Drug and Alcohol Abuse, 32(1), 111–120. https://doi.org/10.1080/00952990500328695
- Tripathi, R., Rao, R., Dhawan, A., Jain, R., & Sinha, S. (2020). Opioids and sleep – A review of literature. Sleep Medicine, 67, 269–275. https://doi.org/10.1016/j.sleep.2019.06.012
- Ulrich-Lai, Y. M., Arnhold, M. M., & Engeland, W. C. (2006). Adrenal splanchnic innervation contributes to the diurnal rhythm of plasma corticosterone in rats by modulating adrenal sensitivity to ACTH. American Journal of Physiology. Regulatory, Integrative and Comparative Physiology, 290(4), R1128–35. https://doi.org/10.1152/ajpregu.00042.2003
- Wemm, S. E., & Sinha, R. (2019). Drug-induced stress responses and addiction risk and relapse. Neurobiology of Stress, 10, 100148. https://doi.org/10.1016/j.ynstr.2019.100148
- Wood, C. E., Shinsako, J., Keil, L. C., & Dallman, M. F. (1982). Adrenal sensitivity to adrenocorticotropin in normovolemic and hypovolemic conscious dogs. Endocrinology, 110(4), 1422–1429. https://doi.org/10.1210/endo-110-4-1422
- Wood, C. E., Shinsako, J., Keil, L. C., Ramsay, D. J., & Dallman, M. F. (1982). Apparent dissociation of adrenocorticotropin and corticosteroid responses to 15 ml/kg hemorrhage in conscious dogs. Endocrinology, 110(4), 1416–1421. https://doi.org/10.1210/endo-110-4-1416
- Yurtsever, T., Streit, F., Foo, J. C., Trifonova, S., Kumsta, R., Muller, C. P., Turner, J. D., Meyer, J., & Schote, A. B. (2019). Temporal dynamics of cortisol-associated changes in mRNA expression of glucocorticoid responsive genes FKBP5, GILZ, SDPR, PER1, PER2 and PER3 in healthy humans. Psychoneuroendocrinology, 102, 63–67. https://doi.org/10.1016/j.psyneuen.2018.11.033
- Zhou, Y., & Leri, F. (2016). Neuroscience of opiates for addiction medicine: From stress-responsive systems to behavior. Progress in Brain Research, 223, 237–251.
- Zhou, Y., Leri, F., Ho, A., & Kreek, M. J. (2013). Suppression of hypothalamic-pituitary-adrenal axis by acute heroin challenge in rats during acute and chronic withdrawal from chronic heroin administration. Neurochemical Research, 38(9), 1850–1860. https://doi.org/10.1007/s11064-013-1091-3