Abstract
Stress during development affects maternal behavior and offspring phenotypes. Stress in adolescence is particularly consequential on brain development and maturation, and is implicated in several psychiatric disorders. We previously showed that pre-reproductive stress (PRS) in female adolescent rats affects behavior and corticotropin releasing hormone receptor 1 (CRHR1) expression in first- (F1) and second- (F2) generation offspring. We further showed that offspring phenotypes are partially reversed by post-stress treatment with fluoxetine (FLX) or the CRHR1 antagonist NBI27914 (NBI). Epigenetic processes, such as DNA methylation, are implicated in the stress response and interact with maternal care quality across generations. Here, we asked whether PRS and FLX or NBI exposure would affect maternal care and global DNA methylation in the brains of exposed dams and their adult F1 and paternally-derived F2 offspring. We found that PRS decreased self-care while increasing pup-care behaviors. PRS also increased DNA methylation in the amygdala of dams and their F1 male offspring, but decreased it in F2 females. Drug treatment had no effect on maternal care, but affected DNA methylation patterns in F0 and F1 generations. Furthermore, PRS altered the expression of DNA methylating enzymes in brain, blood and oocytes. Finally, maternal care variables differentially predicted methylation levels in PRS and control offspring. Thus, the effects of adolescent stress are long-lasting and impact methylation levels across three generations. Combined with our findings of epigenetic changes in PRS-exposed oocytes, the present data imply that biological changes and social mechanisms act in concert to influence adult offspring phenotypes.
Introduction
The post-partum period is a critical timepoint for both mother and child. The mother’s care-giving style during this time is greatly affected by her past, particularly by aversive experience (Choi et al., Citation2022). In turn, early-life parent–child interactions have a tremendous impact on offspring survival rates, growth, physiology and adult behavior (Cameron et al., Citation2005; Curley & Champagne, Citation2016; Lauby et al., Citation2021).
Rodent studies have aspired to uncover mechanisms underlying the association between female stress exposure and early life development. In rats and mice, dams are the exclusive providers of pup care, and display natural variation in licking and grooming (LG), nursing style and pup handling (Champagne et al., Citation2003; Francis et al., Citation1999; Liu et al., Citation2000). While there is ample evidence for the impact of gestational stress on maternal care (e.g. (John, Citation2019)), few studies have focused on pre-gestational stress during adolescence despite its well-documented impact on brain development (Romeo, Citation2017). The results of these studies are inconclusive (Gemmel et al., Citation2018; Kott et al., Citation2018; Pisu et al., Citation2017), owing perhaps to variability in stress parameters and maternal care measurement.
Stress affects DNA methylation, a molecular process that alters gene expression and is catalyzed by a family of DNA methyltransferases (DNMTs; (Moore et al., Citation2013)). Both acute and chronic stress were shown to affect global methylation patterns (Blaze et al., Citation2018, Citation2013; Doherty et al., Citation2016; Mychasiuk et al., Citation2016; Rodrigues et al., Citation2015), as well as methylation of stress-associated gene promoters in brain, blood and germline cells (Barrès et al., Citation2012; Day & Sweatt, Citation2010; Miller & Sweatt, Citation2007). Methylation abnormalities were reported in several stress-related psychopathologies including major depressive disorder, anxiety disorders and schizophrenia (Brown et al., Citation2019; Fries et al., Citation2016; Palma-Gudiel et al., Citation2015; Pries et al., Citation2017).
Stress and trauma affect not only the exposed individuals but also their offspring (Franklin et al., Citation2011, Citation2010; Mychasiuk et al., Citation2013; Plank et al., Citation2021; van Steenwyk et al., Citation2018; Yehuda et al., Citation2014; Zaidan et al., Citation2021, Citation2013). Animal studies seeking a biological mechanism for this phenomenon have focused mostly on male rodents and on the impact of early life stress (Franklin et al., Citation2011, Citation2010; Mychasiuk et al., Citation2013; van Steenwyk et al., Citation2018). Studies in our lab have shown that mild, chronic pre-reproductive stress (PRS) to adolescent female rats alters anxiogenic behavior in F1 as well as maternally- and paternally-derived F2 offspring (Zaidan et al., Citation2021, Citation2013; Zaidan & Gaisler-Salomon, Citation2015). Furthermore, PRS impacts offspring’s HPA axis function and corticotropin-releasing hormone receptor 1 (CRHR1) mRNA gene expression in brain, blood, and oocytes (Bock et al., Citation2016; Shachar-Dadon et al., Citation2009; Zaidan et al., Citation2021, Citation2013; Zaidan & Gaisler-Salomon, Citation2015). We have shown that some PRS-induced changes in offspring are reversed by post-stress pre-reproductive maternal treatment with the CRHR1 inhibitor NBI 27914 or the serotonin selective reuptake inhibitor (SSRI) fluoxetine (Zaidan et al., Citation2021).
Methylation patterns in offspring are also affected by parental stress, specifically during gestation or in early life (Bierer et al., Citation2020; Franklin et al., Citation2010). The impact of adolescent stress on methylation patterns in exposed females and their offspring has not been studied. Furthermore, while some female PRS effects on offspring are reversed by pharmacological treatment of dams, the reversal of stress effects on methylation patterns has not been tested.
In this study, we asked whether adolescent PRS in female rats would affect maternal behavior and global DNA methylation in the medial prefrontal cortex (mPFC) and amygdala (AMY) which are implicated in the stress response and are significantly altered during adolescence (Akirav & Maroun, Citation2007; Cunningham et al., Citation2002; Tottenham & Galván, Citation2016). We further asked whether subsequent treatment with the antidepressant drug fluoxetine (FLX) or the CRHR1 antagonist NBI 27914 (NBI) would reverse these abnormalities. Next, we asked how PRS would impact global DNA methylation levels across generations. Finally, we asked whether dam methylation and maternal care profiles can predict methylation changes in their F1 and F2 offspring.
Methods and materials
Animals
Virgin adolescent female and adult male Sprague-Dawley rats were purchased from Envigo (Jerusalem). Housing conditions (except during the stress procedure) included wood-flake bedding, ad lib food and water, 12h artificial lighting during the day (07-19h), and temperature maintained at 22 ± 2 °C. Rats were minimally handled except for weekly weighing, and soiled bedding was partially replaced periodically, without removing the rats, so that home-cage conditions were minimally disrupted.
Animals were randomly distributed across groups (see Experimental procedure below) and handled in accordance with the NIH guidelines for the Care and Use of Laboratory Animals, 8th edition (Council, Citation2010). The study was approved by the University of Haifa Committee on animal experimentation (294/13, 351/14).
Experimental procedure
The experimental timeline is outlined in . Adolescent female rats (P40, equivalent to mid-adolescence (Brenhouse & Andersen, Citation2011; García-Cabezas & Zikopoulos, Citation2019; Sengupta, Citation2013), were group housed (4-5 rats per cage) in 56x35x19cm cages. Cages were randomly divided into control (C) and PRS conditions. PRS rats underwent a 7-day chronic unpredictable stress procedure as previously described (Shachar-Dadon et al., Citation2009; Zaidan et al., Citation2021, Citation2013; Zaidan & Gaisler-Salomon, Citation2015). Briefly, the stress procedure was conducted during 7 consecutive days and included (1) 15 min warm swim (22 °C), (2) 10 min cold swim (15 °C) followed by warming (3) 24h food and water deprivation, (4) 24h constant light, (5) 3 times 30 min on a raised platform at 30 min intervals, (6) electric shock (10 × 0.5 mA 1s at 30s intervals), (7) 24h crowding (8 females in a cage 56x35x19 cm high) with constant light. Following the stress procedure, the rats were returned to their original cages. Twenty-four hours later (P48), females from C and PRS groups were divided into 2 cohorts. Rats in cohort 1 underwent a 2-day ovulation induction procedure (performed as previously (Shkolnik et al., Citation2011; Zaidan et al., Citation2021, Citation2013)) starting on P57. On P61 (14 days after the end of the stress procedure) mature oocytes were counted and collected, trunk blood was collected, and brain was extracted. Oocyte, blood and mPFC and AMY samples were used for DNMT enzyme mRNA expression levels. In cohort 2, twenty-four hours after the end of the stress procedure, females were randomly injected intraperitoneally (IP) with either vehicle (VEH), the selective CRHR1 antagonist NBI (5 mg/ml; 5 days) or the selective serotonin reuptake inhibitor FLX (5 mg/kg, injection volume 0.5 ml) for 7 consecutive days (Zaidan et al., Citation2021). One week later, the females from each group were mated with behaviorally naïve adult male rats, who were removed from the mating cage 7 days later. Female rats were returned to their home cage; pregnancy was verified by weekly weighing. Each pregnant rat was moved to a 37x30x19cm cage 7 days prior to parturition.
Figure 1. Experimental procedure. Timeline of the experimental procedure in the parent (F0) and offspring (F1 and F2) generations.
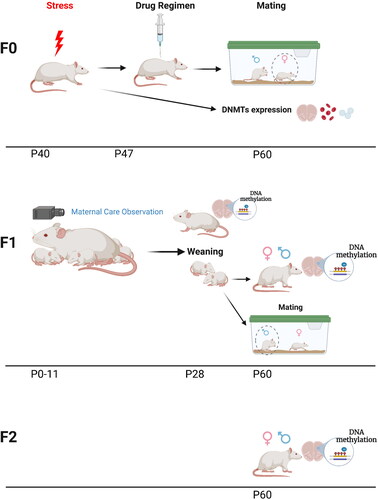
On P0 (up to 24h after birth), the litters were culled to 10 pups, with approximately equal numbers of males and females. Dams and pups were videotaped in their home cages for 20 min each day for 12 days starting on P0 (see details below). Cages were placed on a table in the animal room and returned back after the recording session; no disturbance or contact with animals was made.
Pups remained in their home cages undisturbed until P30, then weaned and raised in same-sex, same-condition groups of 4–5. Dams from each group were sacrificed after weaning; mPFC and AMY were extracted for the measurement of global DNA methylation. On P60, a subset of randomly selected behaviorally naïve F1 males and females from all groups and litters were sacrificed. mPFC and AMY from a subset of animals in all groups were extracted for measurement of global DNA methylation.
Behaviorally-naïve adult male F1-C and F1-PRS offspring were mated with naïve adult female rats. Mating, pregnancy, weighing, and weaning of F2 pups were conducted as described above for F1. F2 pups were raised undisturbed until P30, then weaned and raised in same-sex, same-condition groups of 4–5. F2-C and F2-PRS males and females were sacrificed at P60, and their mPFC and AMY were extracted for the measurement of global DNA methylation.
Drugs
Doses and administration regimes of NBI and FLX were determined according to previous publications, including our own (Hummel et al., Citation2010; Lee et al., Citation2001; Núñez et al., Citation2010; Zaidan et al., Citation2021). NBI (Sigma- Aldrich, St. Louis, MO) and FLX (Sigma- Aldrich) were dissolved in dimethyl sulfoxide (DMSO) and diluted with 0.9% saline to a concentration of 5 mg/ml with a final DMSO concentration < 0.1%. Drugs were administered i.p. at a volume of 2 ml/kg for 5 days (NBI) or 7 days (FLX). NBI-injected rats received 2 additional days of VEH injections so the total injection period for all groups was 7 days. The VEH group was injected with < 0.1% DMSO in saline.
Maternal behavior
The maternal behavior assessment protocol was performed as previously described, with slight modifications (Champagne et al., Citation2003; Cutuli et al., Citation2015; Myers et al., Citation1989; van Hasselt et al., Citation2012). Detailed observations of maternal and pup behaviors were recorded on the day of birth (P0; up to 12h after birth) and continued until Day 12. Maternal behavior was recorded during 20 min sessions daily, up to 3 times/day, at random times (07:00–11:00, 11:00–15:00, and 15:00–19:00h), for 12 days. All video recordings were made while the dam and litter were in their house cage.
The video recordings were randomly assigned to 3 trained observers blind to the experimental conditions. Every video was rated by at least two observers. Observers manually identified specific behaviors once every 60 sec for 1 sec each time, so that up to 21 observations were recorded for each video. Observer reliability was evaluated by having all 3 observers rate several overlapping videos, chosen randomly. The percent of agreement among all 3 observers across all behaviors was 81%. Frequency of the following 15 behaviors in 4 different categories was collected, in line with previous literature (Champagne et al., Citation2003; Cutuli et al., Citation2015; Fleming & Rosenblatt, Citation1974; Liu et al., Citation2000; Myers et al., Citation1989; Roth & Sullivan, Citation2005):
Dam Self-Care behaviors
Exploration: the dam is moving around the cage and sniffing the bedding, rearing and leaning on the wall but not carrying pups or nesting material; Self-Grooming: the dam is wiping, licking, combing, or scratching any part of its own body; Resting: the dam is lying down alone, out of the nest with no contact with pups. Eating; Drinking.
Pup-Care behaviors
Contact: the dam is in contact with pups but not nursing; Retrieving: the dam is picking up pup in her mouth and carrying it to or from the nest; Licking and Grooming (LG): the dam is licking or grooming any part of the pup’s body; Nest Building: the dam is pushing, pulling and manipulating the bedding to form a nest or tidy it. Arched-Back Nursing: the dam is domed over all pups with the body arched, hind-limbs splayed and no apparent movement; Blanket Nursing: the dam lies over the litter but with no arch in its back and without obvious hind-limb extension; Passive Nursing: the dam is lying either on its back or side while pups nurse.
Aggressive behavior
Rough handling and aggression toward pup: the dam is aggressively grooming a pup or transporting a pup by a limb, stepping or jumping on the pup; throwing the pup some distance, dragging a pup across the cage, or dropping a pup during retrieval or transport; Pushing away: the dam is running from approaching pup or pushing a pup away from her.
Atypical behavior
All distinct behaviors that cannot be classified in the previous categories, e.g. sudden fast rotation (without holding a pup in mouth) to one direction for several turns, burying one or two pups with bedding completely, and in some cases continuously adding more when pups reappear.
Behavioral categories are not mutually exclusive. For example, LG often occurred while the mother was nursing the pups. Arched-Back Nursing, Blanket Nursing and Passive Nursing were pooled together under the Nursing variable. An average between the observers to each video was calculated. Observations of each animal on each day were averaged.
Tissue collection
Adult rats were sacrificed by decapitation and bilateral samples from mPFC (consisting of prelimbic and infralimbic regions) and AMY (central and basolateral nuclei) were removed in the cryostat using 0.5 or 1.0 mm punches (Paxinos & Watson, Citation2006). Trunk blood was collected and stored in Vacuette K2EDTA tubes (Greiner Bio-one, Austria) at −80 °C. RNA was extracted using Monarch Total RNA Miniprep Kit (New England Biolabs, USA) according to the manufacturer’s instructions (Zaidan et al., Citation2021). Oocytes were extracted following ovulation induction as previously described (Goren & Dekel, Citation1994; Shkolnik et al., Citation2011; Zaidan et al., Citation2021, Citation2013). All samples were immediately placed on dry ice and kept at −80 °C until further processing.
Measurement of global DNA methylation
The DNA from the tissue samples was extracted with the use of Quick-gDNA™ MicroPrep Kit (Zymo Research, CA, USA). Next, the isolated DNA has been quantified spectrophotometrically with the use of the NanoDrop ND-1000 UV-Vis Spectrophotometer (Thermo Fisher Scientific, MA, USA). An Imprint Methylated DNA Quantification Kit (Sigma-Aldrich, MO, USA) was used to determine the DNA methylation status. According to the manufacturer’s instructions, 100 ng of purified DNA was added to each well where the methylated DNA was detected by capture and detection antibodies. The absorbance of the colorimetric reaction product was measured at 450 nm using an Infinite M200PRO microplate reader (Tecan, Switzerland) as previously described (Kajta et al., Citation2019; Wnuk et al., Citation2021). Next, according to the manufacturer’s protocol, the standard curve has been created by plotting A450av Methylated Control DNA - A450av Blank versus ng of the Methylated Control DNA using linear regression. Then, based on the linear regression, the amount of methylated DNA in samples was calculated based on the formula: ng methylated DNA in sample = [(A450 av Sample - A450 av Blank) – intercept]/slope.
Quantitative real-time PCR (qRT-PCR) for assessment of RNA expression
RNA extraction, cDNA preparation, and quantitative real-time PCR (qRT-PCR) were performed as previously described (Zaidan et al., Citation2021). cDNA was amplified in a 10 µL reaction (0.6 µL primers (10 µM), 5 µL PerfeCTA SYBR green (Quanta Biosciences, Gaithersburg, USA), 2 µL cDNA (diluted 1:5 with H2O) and 2.4 µL ddH2O), by a Step One RT-PCR machine (Applied Biosystems, Carlsbad, CA). Primers for DNMT1, DNMT2, DNMT3a, DNMT3b, and DNMT3l were designed and synthesized by Agentek (Tel Aviv, Israel). Primer suitability was determined using standard curve analysis, melting curve analysis, and linearity at the threshold (Pfaffl et al., Citation2004). Fold-change values were calculated using the ddCt method (Livak & Schmittgen, Citation2001) relative to the housekeeping gene hypoxanthine phosphoribosyl transferase (HPRT) or 18s (oocytes). dCt values for HKGs were similar between groups (<.5 Ct, t-tests: all p’s > 0.1).
Statistical analyses
The impact of stress and drug treatment on F0 methylation levels was investigated using 2-way analysis of variance (ANOVA), separately for mPFC and AMY data. The effect of stress and drugs on maternal care was investigated using multivariate analysis of variance (MANOVA). The impact of PRS and drug treatment on F1 methylation were examined using a 2-Way ANOVA, separately for mPFC and AMY in males and female offspring. The Least Significant Difference (LSD) test was used for posthoc comparisons when the interaction was significant. Data assessing the impact of PRS on F2 methylation were analyzed with a 1-Way ANOVA, separately for mPFC and AMY in male and female offspring. The impact of PRS on DNMTs was analyzed with a 1-Way ANOVA, separately for mPFC, AMY, blood, and oocyte.
Correlations between DNMTs, mPFC, and AMY methylation levels in F0, F1, and F2, and between F0 methylation and maternal behavior were determined using non-parametric Spearman correlation coefficients since the global methylation variable was not normally distributed (Shapiro–Wilk test).
We constructed separate mixed models for each brain region (mPFC or AMY to examine the association of F0 variables (methylation, dam-pup interactions) with F1 and F2 methylation. Our data formed a 3-level multilevel structure in which F2 data were nested within F1 units and F1 data were nested within F0 units. For instance, F1 methylation data from different offspring of the same dam were considered nested within that dam’s data and thus constituted partially dependent data, requiring mixed models (also referred to as multilevel or hierarchical linear models) analysis (Raudenbush & Bryk, Citation2002). For all mixed model analyses, we used an unstructured covariance structure, as well as restricted maximum likelihood estimation. All dichotomous variables were effects-coded (i.e. −1 and +1) and all continuous variables were centered to facilitate interpretation.
For models with a significant interaction of Sex or Group with F0 DNA methylation or maternal care, separate linear mixed models were constructed for each variable level for the purpose of graphical representation. Significance level was set at p<.05. Results that approach significance were defined as .05≤p≤.075.
All statistical analyses were performed using IBM SPSS Statistics, Version 27 (IBM, Chicago, Illinois) and GraphPad Prism 7.0 (GraphPad Software, San Diego, CA). Graphs were drawn using GraphPad Prism 7.0 and BioRender (https://biorender.com/).
Results
Dams and neonates: Maternal behavior
We assessed the maternal behavior of control and PRS-exposed dams for 12 days, starting on P0. Behaviors were divided into four categories: Pup Care, Self Care, Aggressive and Atypical behavior (see Methods). Overall, dams exposed to PRS showed decreased Self Care (2 × 3 ANOVA; Group F(1,14)=6.7, p < 0.05; left and right) and increased Pup Care (Group F(1,14)=9.3, p < 0.01; 2b left and right). No differences were found in aggressive or atypical behavior categories (all p’s > 0.1). PRS-induced differences were generally observed across drug conditions (see details below).
Figure 2. Maternal behavior in control and PRS-exposed dams across assessment days (D1-12). Stress-exposed dams showed less Self Care across drug conditions (a, left and right; n = 10/group), specifically less eating behavior (c). Stress-exposed dams also showed increased Pup Care, regardless of drug treatment (b, left and right; n = 10/group), i.e. more LG (d) and a trend toward more frequent nursing (e). Data presented as means and standard errors, and/or individual values. #p < 0.075. *p < 0.05, relative to Control.
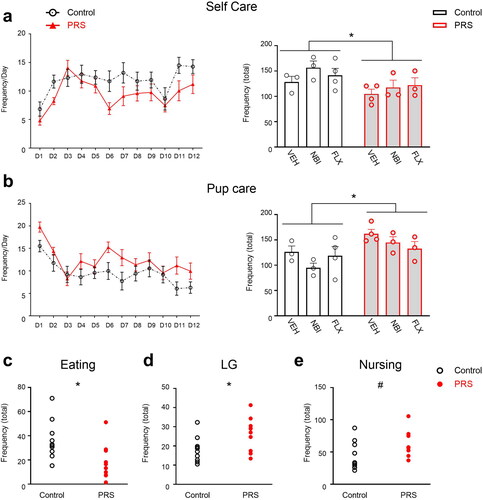
In the Self Care category, PRS-exposed dams showed a trend toward decreased exploration (Group, F(1,14)=3.7, p = 0.075) and less Eating behavior (Group, F(1,14)=8.9, p < 0.01; 2c). NBI-treated dams showed increased Eating behavior compared with VEH- and FLX-treated dams (Drug F(2,14)=4.8, p < 0.05; not shown). No differences in dam weight were observed immediately following parturition or 30 days later (p’s > 0.1). No stress- or drug-induced differences were observed in Drinking, Self-Grooming or Resting away from the pups (p’s > 0.1).
In the Pup Care category, PRS-exposed dams showed more LG behavior across drug conditions (2 × 3 ANOVA, main effect of Group, F(1,14)=6.1, p < 0.05; 2d). PRS-exposed dams also showed a trend toward increased Nursing behavior (F(1,14)=4.3, p = 0.056, 2e), particularly Blanket Nursing (F(1,14)=4.1, p = 0.06324). NBI-treated dams showed a trend toward more Blanket Nursing than FLX, but not VEH-treated animals regardless of stress exposure (Drug, F(2,14)=3.7, p = 0.051). No differences in Arched-Back or Passive Nursing were observed, nor were there any differences in Nest Building or Contact (all p’s > 0.1). C-VEH dams showed more Pup-Retrieving behavior relative to PRS-VEH and C-FLX and C-NBI groups (2 × 3 ANOVA, Group x Drug F(2,14)=5, p < 0.05).
DNA methylation across generations
In the F0 generation (), PRS exposure and/or drug treatment affected global DNA methylation in mPFC and AMY. In mPFC (3a), methylation levels were lowest in the VEH-treated group compared to both NBI and FLX, regardless of prior PRS exposure (2 × 3 ANOVA, Drug F(2,33)=37.235, p < 0.0001). In the AMY (3b), both PRS and drug treatment increased DNA methylation (2 × 3 ANOVA, Group F(1,36)=8.015, p < 0.01; Drug F(2,36)=84.627, p < 0.0001; PRS effects to the right of blue dashed line). There was a positive correlation between mPFC and AMY methylation in both Control (n = 20, r=.540, p < 0.05) and PRS (n = 19, r=.591, p < 0.01) conditions (3c). Assessing the correlation between maternal behavior and F0 methylation levels, we found that Pup Care and LG were negatively correlated with dam AMY methylation levels in control, but not PRS, rats (n = 9, r=–.667, p < 0.05; n = 9, r=–.717, p < 0.05, respectively; p’s < 0.05. See full correlations in Table S1 in SI).
Figure 3. PRS- and drug- induced changes in global DNA methylation in F0, F1 and F2. In F0 (a-c), NBI and FLX treatment increased methylation levels in mPFC (a). In the AMY (b), NBI and FLX increased methylation, as did PRS; PRS effects across drug conditions are depicted to the right of blue dashed line. (c) Methylation levels in mPFC and AMY were positively correlated in both Control and PRS rats. In F1 offspring (d-i), NBI or FLX treatment increased methylation levels in male (d) and female (e) mPFC. In males (d), PRS followed by NBI treatment led to higher methylation than PRS or NBI exposure alone. PRS followed by FLX resulted in control-like methylation levels. In F1 female mPFC (e), PRS marginally increased methylation levels in F1-VEH offspring, and subsequent NBI or FLX treatment amplified this effect. In the AMY of F1 males (f), maternal NBI treatment decreased methylation regardless of PRS, while PRS increased methylation across drug conditions (depicted to the right of blue dashed line). In F1 female AMY (g), PRS had no effect. Maternal NBI treatment decreased methylation regardless of PRS, and maternal FLX increased methylation levels in F1-C but not F1-PRS rats. We found no correlation between mPFC and AMY methylation in F1-C (h), and a negative correlation in F1-PRS (i). In F2 offspring (j-l), we found in the mPFC a trend toward decreased methylation in males, and no effect in females (j). In AMY, we found no effect in males, while F2-PRS females showed decreased methylation levels relative to F2-C (k). We found no correlation between mPFC and AMY methylation in F2-C, and a negative correlation in F2-PRS (l). Data presented as means and standard errors, and/or individual values. #p < 0.075. *p < 0.05. **p < 0.001.
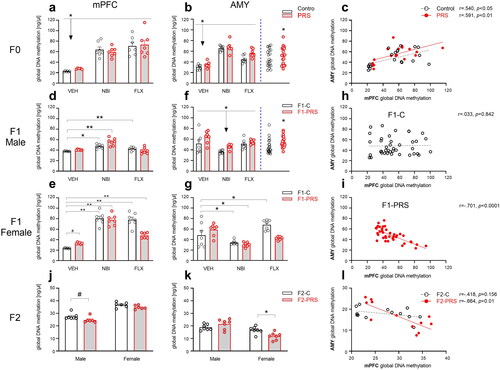
PRS and drug treatment in F0 also affected global methylation in F1 (). In mPFC (3d-e), methylation levels in females were generally higher than in males (F(1,67)=49.746, p < 0.0001), although separate analysis in drug groups revealed that this pattern was prominent in the FLX and NBI groups (1-Way ANOVAs; F(1,25)=12.042, p < 0.01; F(1,26)=62.973, p < 0.0001; respectively) but not in the VEH group where higher methylation rates were found in males (F(1,22)=50.454, p < 0.0001).
Separate analysis in males and females revealed that while PRS did not affect methylation, pre-reproductive NBI or FLX treatment increased methylation in F1-C male offspring (3d). Higher-than-control DNA methylation levels were also observed in the F1-PRS/NBI, but not F1-PRS/FLX, group (2 × 3 ANOVA, Drug F(2,34)=20.844, p < 0.0001, Drug x Group F(2,34)=18.434, p < 0.0001). In females (3e), PRS marginally increased methylation in the VEH group. Pre-reproductive treatment with either NBI or FLX increased methylation in F1-C offspring. As in males, PRS mitigated the effects of FLX on methylation in offspring, and methylation was lowest in offspring of dams not exposed to either PRS or drug treatment, compared to all other groups (2 × 3 ANOVA, Group F(1,33)=7.265, p < 0.05, Drug F(2,33)=106.239, p < 0.0001, Drug x Group F(2,33)=16.038, p < 0.0001).
In the AMY (), no sex-related differences in DNA methylation levels were found (F(1,71)=2.701, p = 0.155). In male offspring (3f), maternal PRS increased methylation across drug conditions (Group, F(1,36)=7.64, p < 0.01; PRS effects depicted to the right of blue dashed line). Methylation levels were lowest in the NBI group regardless of PRS exposure (Drug, F(2,36)=9.606, p < 0.001). In females (3g), FLX treatment increased methylation levels in F1-C but not F1-PRS offspring. NBI treatment decreased methylation regardless of PRS exposure (Drug F(2,35)=16.033, p < 0.0001, Drug x Group F(2,35)=7.875, p < 0.01). Combining male and female data, we found a negative correlation between F1 methylation levels in mPFC and AMY in F1-PRS (n = 38, r=–.701, p < 0.0001), but not F1-C (n = 40, r=.033, p = 0.842), rats, regardless of drug administration (3h-i).
We next examined the effects of PRS in F0 on global DNA methylation in F2 (). In mPFC (), methylation levels were higher in females than males (Sex, F(1,21)= 125.253, p < 0.0001). F2-PRS males trended toward decreased methylation levels relative to F2-C males (F(1,11)=4.127, p = 0.067); no effect was found in females (p > 0.075).
In the AMY (), DNA methylation levels were higher in males than females (Sex, F(1,24)= 24.764, p < 0.0001). F2-PRS females showed decreased methylation levels relative to F2-C (F(1,12)= 9.82, p < 0.01); no effect was found in males (p > 0.1). As in F1, in F2-PRS offspring we found a negative correlation between methylation in mPFC and AMY (n = 12, r=–.664, p < 0.01); no correlation was found in F2-C offspring (n = 13, r=–.418, p = 0.156, 3l). As can be seen in , methylation changes were present in vehicle-treated stress-exposed dams and their F1 and F2 offspring and were manifested differently in males and females.
Figure 4. Flow chart illustrating PRS effects on DNA methylation in VEH-treated female rats (F0) and their first- and second-generation offspring (F1 and F2, respectively).
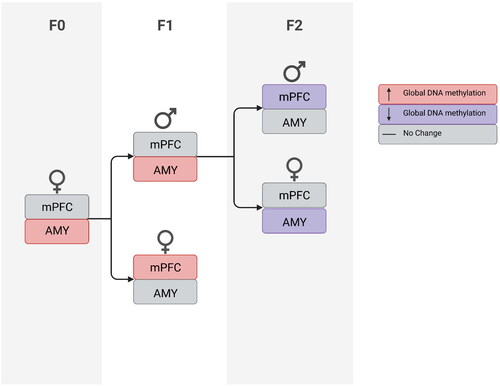
An assessment of demethylating enzyme expression in F0 2 weeks following stress () revealed elevated expression of DNMT1 (1-way ANOVA, F(1,13)=5.58, p < 0.05), DNMT3a (F(1,12)=6.24, p < 0.05), DNMT3b (F(1,12)=5.63, p < 0.05) and DNMT3l (F(1,11)=8.37, p < 0.05) but not DNMT2, in mPFC (5a). In the AMY, stress increased DNMT1 expression (F(1,10)=9.06, p < 0.05) and there was a trend toward an increase in DNMT3l (F(1,12)=4.19, p = 0.063). DNMT3b expression was decreased in stress-exposed dams (F(1,15)=6.17, p < 0.05); no differences were found in DNMT3a expression, and DNMT2 expression was not detected (5b).
Figure 5. Chronic unpredictable stress in adolescence affects methylation enzyme mRNA expression in adulthood. In mPFC (a), stress increased DNMT1, DNMT3a, 3b and 3 l expression. In AMY (b), stress increased DNMT1 and decreased DNMT3b; a trend toward increased DNMT3l expression was also found. In blood (c), stress increased DNMT1 and decreased DNMT3a, 3b and 3 l expression. In oocytes (d), stress decreased DNMT3b expression. Data presented as means and standard errors. (e) Correlations between DNMT enzyme mRNA levels in mPFC, AMY and Blood of F0 dams. #p < 0.075. *p < 0.05. **p < 0.001.
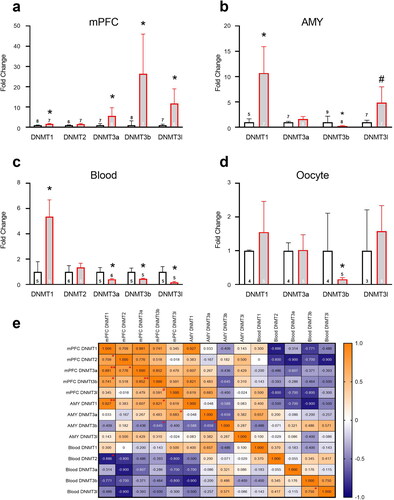
In blood, stress increased DNMT1 mRNA expression (F(1,8)=5.7, p < 0.05) and decreased DNMT3a (F(1,9)=6.64, p < 0.05), DNMT3b (F(1,8)=6.94, p < 0.05) and DNMT3l F(1,8)=7.86, p < 0.05) expression; no differences were found in DNMT2 (5c). In oocytes, stress decreased DNMT3b (F(1,7)=8.60, p < 0.05), and no difference were found in DNMT1, DNMT3a and DNMT3l expression; DNMT2 expression was not detected (5d).
Assessing correlations between DNMT expression levels in the mPFC, AMY and blood, we found a positive correlation between DNMT1 in AMY and mPFC (n = 10, r=.927, p < 0.001), and a negative correlation between DNMT3b in AMY and mPFC (n = 20, r=–.645, p < 0.05; see additional correlations in 5e). Interestingly, while mPFC and AMY methylation levels were generally positively correlated, there was a negative correlation between blood and mPFC methylation levels.
F0 variables as predictors of methylation levels in F1 and F2
To analyze the impact of F0 methylation on F1 methylation, we first constructed a linear mixed-effect model with 3 independent variables and all their possible interactions (F0 PRS, F0 methylation, and F1 sex) as predictors of F1 methylation levels. Separate models were constructed for each brain region (mPFC, AMY) and for each drug treatment regimen (VEH, FLX, NBI). Regression analysis findings for VEH data are summarized in Table S2 in SI. We began by analyzing VEH data in each brain region. In mPFC, we found no significant main effects or interactions between Group, F0 methylation or Sex. In the AMY (), we found a significant F0 Methylation x Group interaction; increased methylation in dams predicted lower methylation in their F1-C, but not F1-PRS offspring.
Figure 6. F0 methylation as predictor of methylation levels in F1 and F2 AMY. In the AMY of F1 offspring (a), increased methylation in F0 predicted lower methylation in F1-C, but not F1-PRS rats. (b) increased methylation levels in F0 AMY predicted lower methylation levels in F2 AMY regardless of Group or offspring Sex. Numbers on the X axis reflect the range of values in our dataset.
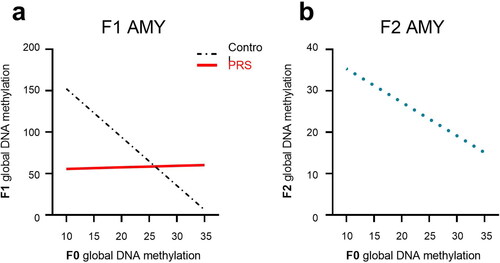
To analyze the relationship between F0 and F2 methylation, we constructed a mixed-effect model with 3 independent variables and all their possible interactions (F0 stress exposure, F0 methylation, and F2 sex) as predictors of F2 adult offspring methylation. Separate models were constructed for each brain region (mPFC, AMY). In the mPFC, there were no main effects or interactions. In the AMY, the constructed model examining F0 Group, F0 methylation, and F2 Sex as fixed effects yielded no main effects or interactions. However, a linear mixed model with F0 methylation as the only predictor revealed a negative association between F0 and F2 methylation levels, indicating that regardless of Group or offspring Sex, increased methylation levels in F0 predicted lower AMY methylation levels in F2 (6b).
We next asked whether maternal behaviors and F1 offspring sex predict F1 methylation levels in mPFC and AMY of C and PRS groups (). A separate model was constructed for each maternal behavior variable. The only variable associated with DNA methylation in adult F1 mPFC was Pup Care. Our analysis showed that increased Pup Care predicted lower methylation levels in male, but higher methylation levels in female, rats (Pup Care x Sex interaction). In males and females combined, we found that increased Pup Care predicted lower methylation in control, but not PRS, rats (Pup Care x Group interaction, 7a). All other models examining the ability of specific maternal behavior variables, e.g. LG, Nursing, Self Care, Self-Grooming, Independent Movement, to predict F1 methylation in mPFC did not yield significant findings (see Table S2).
Figure 7. Maternal care as predictor of methylation levels in F1 offspring. In mPFC (a) increased Pup Care predicted lower methylation in F1-C, but not F1-PRS, offspring. In the AMY(b), increased LG predicted lower methylation in F1-C, but not F1-PRS, rats; while increased Self-Grooming (c) predicted higher methylation levels in F1-C, but not F1-PRS, offspring. Numbers on the X axis reflect the range of values found in our dataset.
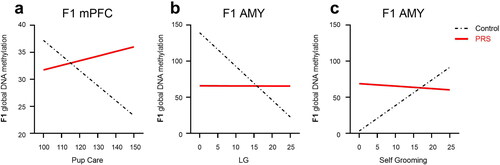
In the AMY, we found that as in mPFC, increased Pup Care predicted lower methylation levels in adult F1 AMY, regardless of F0 Group or F1 Sex (main effect of Pup Care). Examining specific behaviors in this category, we found that increased LG predicted lower methylation in F1-C, but not F1-PRS, rats (LG x Group interaction, 7b). In contrast, increased Nursing predicted higher methylation in F1-C, but not F1-PRS, rats (a Nursing x Group interaction, not shown).
In the Self Care category, higher Self Care predicted higher methylation in F1-C, but not F1-PRS, rats (Self Care x Group interaction). In particular, indicating that increased Self-Grooming (7c) and Independent Movement (not shown) predicted higher methylation levels in control, but not PRS, F1 offspring (Self-Grooming x Group and Independent Movement x Group interactions, respectively).
Separate examination of FLX and NBI data, using the same variables and models used to predict F1 methylation levels in the VEH group, yielded no significant findings (Tables S3,S4).
Discussion
Our findings indicate that stress to female rats during adolescence, a critical developmental time window, impacts maternal behavior and methylation patterns across generations. Furthermore, while post-exposure drug treatment has no effect on maternal behavior, it does alter methylation patterns in exposed rats and their adult offspring. Finally, maternal care quality can predict methylation levels in the AMY of control, but not PRS, offspring.
Our study shows that PRS in adolescent female rats led to increased LG and nursing behavior but reduced pup retrieval. Overall, pup care behaviors were more frequent in stress-exposed dams while non-maternal parameters, i.e. self-care behaviors, were less frequent. These findings are in agreement with previous studies showing increased pup care following early life (Coley et al., Citation2019; Kosten & Kehoe, Citation2010; Stamatakis et al., Citation2015) or post-partum stress (McLeod et al., Citation2007). Furthermore, Kosten and Kehoe (Citation2010) found increased LG but reduced pup retrieval behavior, along with reduced non-maternal behaviors following early-life social isolation, similar to our findings following adolescent PRS. Possibly, stress exposure during critical brain development periods boosts maternal behaviors associated with the emotional state of the animal, e.g. LG (Champagne & Meaney, Citation2006), but leads to an overall decrease in motivation and hedonic behavior, e.g. pup retrieval and self-care behaviors. Notably, the interpretation of maternal care results may not be straight forward: a quantitative increase in LG may not reflect enhanced maternal care quality (Couto-Pereira et al., Citation2016). However, reduced self-care after parturition may be indicative of post-partum depression- or apathy-like behavior (Becker et al., Citation2021; Millstein & Holmes, Citation2007). Previous studies indeed showed that stress-induced changes in maternal care correlate with depression-like phenotypes in female dams (Boccia et al., Citation2007).
To the best of our knowledge, this is the first study to show that stress in adolescent female rodents increases DNA methylation in the adult AMY. Stress exposure during gestation or in early life was previously shown to affect methylation levels in the AMY, particularly in promoters of genes associated with HPA axis function (Boersma et al., Citation2014; van der Doelen et al., Citation2015). Studies in male rodents indicate that stress impacts methylation in the AMY as well as in sperm (Dias & Ressler, Citation2014; Doherty et al., Citation2016; Franklin et al., Citation2010; Roth et al., Citation2014; Sterrenburg et al., Citation2011; Zheng et al., Citation2021). Here, we found that the stress-induced DNA methylation increases in the AMY was accompanied by decreased expression of DNMT3b, involved in de novo methylation (Feng & Fan, Citation2009), in the AMY as well as in oocytes and blood of PRS-exposed females. These findings could indicate that stress-induced methylation-related changes are passed to germline cells via circulation, as suggested by recent human and animal studies (Hua et al., Citation2022; Steenwyk et al., Citation2020; Zaidan et al., Citation2021). The presence of of DNMT3a and DNMT3b mRNA in oocytes, and the absence of DNMT2 expression, is in line with previous findings (Huntriss et al., Citation2004; Petrussa et al., Citation2014).
DNA methylation levels were also elevated in the AMY of F1 adult male, but not female, PRS offspring. Previous rodent studies similarly demonstrated inter-generational transmission of methylation phenotypes (Blaze et al., Citation2017; Coley et al., Citation2019; Dias & Ressler, Citation2014; Franklin et al., Citation2010; Gapp et al., Citation2016; Mueller & Bale, Citation2008; Roth et al., Citation2009; St-Cyr et al., Citation2017), and some of these effects were sex-dependent (Blaze et al., Citation2017; Coley et al., Citation2019; Lei et al., Citation2020). Alterations in maternal care phenotypes may account for changes in offspring methylation patterns. Previous studies show that LG variability correlates with changes in methylation patterns (McGowan et al., Citation2011; Pan et al., Citation2014; Weaver et al., Citation2004; Zhang et al., Citation2010). In the present study, increased LG predicted lower AMY global methylation levels in the offspring of control, but not PRS-exposed female rats, and the opposite pattern was found for self-grooming. These findings support the centrality of pup-care variables, and the inherent differences between pup-care and self-care behaviors, in predicting methylation patterns in adult offspring. Moreover, they indicate that in the presence of maternal stress, the relationship between maternal care and adult phenotypes may be compromised. Future studies should investigate this possibility, focusing on particular maternal behaviors and HPA axis-related genes.
Our findings also indicate that stress in F0 impacts AMY methylation levels in F2 offspring, derived from F1 PRS or control males. The mechanism for this effect remains to be determined. Our previous studies (Zaidan et al., Citation2021, Citation2018, Citation2013; Zaidan & Gaisler-Salomon, Citation2015), join other studies in showing that the transgenerational impact of stress on behavioral, neuroendocrine and molecular phenotypes may be mediated by epigenetic changes in germ cells (Franklin et al., Citation2011, Citation2010; Gapp et al., Citation2014; van Steenwyk et al., Citation2018). The present study also supports this possibility since changes to methylation enzymes, e.g. DNMT3b, was found in oocytes () in line with previous studies showing DNMT expression changes in germline cells following environmental manipulations (Deshpande et al., Citation2020). Notably, some epigenetic marks escape erasure during developmental reprogramming, allowing their transfer from parents to offspring (Kobayashi et al., Citation2012; Radford et al., Citation2014; Seisenberger et al., Citation2012; Tang et al., Citation2015). Future studies should directly examine methylation changes in oocytes, at different time points following fertilization. Presumably, stress-induced epigenetic changes in oocytes would alter gene expression patterns in all offspring cell types, including germline cells, providing a mechanism for transmission to F2. Changes in sperm and oocytes in F1 should be examined in future studies. Additionally, other methylation regulatory processes (e.g. ten-eleven translocation (TET) proteins), possibly accounting for methylation changes in different tissue types (Gu et al., Citation2011) should be examined.
Altered pup care in F1 could also account for methylation changes observed in F2. F1 progeny of stress-exposed F0 females were subject to more LG than progeny of stress-naïve females, and this may have affected their developmental trajectory thus impacting F2 phenotypes. It is interesting to note in this regard that we observed divergent AMY methylation phenotypes in F1 and F2: increased methylation was found in male F1 offspring of stress-exposed females, and decreased methylation was found in female F2 offspring derived from F1 males subjected to more LG behavior (). This could indicate that stress and LG have opposite effects on methylation in subsequent generations. Our findings are in line with previous studies that also found generation-specific effects of environmental exposure (e.g. Constantinof et al., Citation2019). Furthermore, findings from our lab and others indicate that epigenetic changes play a role in transmission of phenotypes to F2 as well (Dias & Ressler, Citation2014; Franklin et al., Citation2011, Citation2010; van Steenwyk et al., Citation2018; Zaidan et al., Citation2021, Citation2018; Zaidan & Gaisler-Salomon, Citation2015).
Most methylation changes we observed across the 3 generations were found in the AMY, with fewer PRS-induced effects observed in mPFC. Previous studies similarly found region-specific effects of stress on methylation levels (Boersma et al., Citation2014). Interestingly, AMY and mPFC methylation levels were positively correlated in F0 in both control and PRS rats, but negatively correlated in F1 and F2 PRS, but not control, offspring.
Post-stress adolescent FLX or NBI treatment had virtually no effect on dam self-care or pup-care behavior. This is the first study to examine the impact of CRHR1 antagonists or pre-gestational FLX administration on maternal care variables. Gestational or perinatal FLX administration was reported by some groups to increase maternal LG and arched-back nursing (Gemmel et al., Citation2018; Pawluski et al., Citation2012) whereas others found no effect on maternal care (Kiryanova et al., Citation2016; Pawluski et al., Citation2012; Rayen et al., Citation2011).
NBI and FLX treatment did increase global methylation levels in mPFC and AMY regardless of prior stress exposure. These drug effects are notable since they are similar to the effects of stress, which also increased methylation levels in the AMY, and were detected several months after stress. Notably, this study measured global methylation; examining methylation at specific gene loci could reveal different methylation patterns for stress and drugs acting via, e.g. CRHR1 or serotonin. Interestingly, glucocorticoids and their receptors act as transcriptional inducers of DNA demethylation (Thomassin et al., Citation2001). We previously showed that NBI reduces blood corticosterone levels (Zaidan et al., Citation2021); this could provide a putative mechanism for its ability to increase DNA methylation rates. We found that FLX treatment enhanced global DNA methylation in mPFC but decreased it in AMY particularly in PRS-exposed animals. The mechanism for FLX effects on methylation are also unknown and could be related to the indirect effects of serotonin on the HPA axis (Budziszewska et al., Citation2004). The effects of drugs on global methylation levels could add to our understanding of the mechanism of action and individual variability in antidepressant drug response. FLX administered to dams post-partum was previously shown to decrease methylation in the hippocampus around the time of pup weaning (Gemmel et al., Citation2016). In our study, F1 drug effects interacted with stress effects and were region-dependent. Future studies should focus on the methylation of gene loci associated with the stress response, e.g. the Crhr1 promoter, or with the serotonergic transmission.
In sum, our findings indicate that stress in adolescence, prior to pregnancy, alters maternal care behavior and impacts global DNA methylation rates in the brain across 3 generations in a sex and region-dependent manner. Combined with our previous findings, these data raise the possibility that germline epigenetic changes and social mechanisms act in concert to impact adult offspring phenotypes. Future studies should seek a mechanism by which maternal care affects adult phenotypes and mitigates the impact of stress across generations; extend the investigation of stress-affected methylation profiles to additional relevant regions and tissue types; and examine early development as well as different stages of adolescence in offspring. Finally, the effects of stress on the HPA axis are complex and likely to be mediated by multiple genes and epigenetic mechanisms; future studies should study these complex relationships in association with stress and the early life environment.
Supplemental Material
Download MS Excel (25.2 KB)Disclosure statement
No potential conflict of interest was reported by the authors.
Additional information
Funding
References
- Akirav, I., & Maroun, M. (2007). The role of the medial prefrontal cortex-amygdala circuit in stress effects on the Extinction of Fear. Neural Plasticity, 2007, 1. https://doi.org/10.1155/2007/30873
- Barrès, R., Yan, J., Egan, B., Treebak, J. T., Rasmussen, M., Fritz, T., Caidahl, K., Krook, A., O’Gorman, D. J., & Zierath, J. R. (2012). Acute exercise remodels promoter methylation in human skeletal muscle. Cell Metabolism, 15(3), 405–15., https://doi.org/10.1016/J.CMET.2012.01.001
- Becker, M., Pinhasov, A., & Ornoy, A. (2021). Animal models of depression: What can they teach us about the human disease? Diagnostics, 11(1)2021, 123. https://doi.org/10.3390/diagnostics11010123
- Bierer, L. M., Bader, H. N., Daskalakis, N. P., Lehrner, A., Provençal, N., Wiechmann, T., Klengel, T., Makotkine, I., Binder, E. B., & Yehuda, R. (2020). Intergenerational effects of maternal holocaust exposure on FKBP5 methylation. The American Journal of Psychiatry, 177(8), 744–753. https://doi.org/10.1176/APPI.AJP.2019.19060618
- Blaze, J., Asok, A., Borrelli, K., Tulbert, C., Bollinger, J., Ronca, A. E., & Roth, T. L. (2017). Intrauterine exposure to maternal stress alters Bdnf IV DNA methylation and telomere length in the brain of adult rat offspring. International Journal of Developmental Neuroscience : The Official Journal of the International Society for Developmental Neuroscience, 62, 56–62. https://doi.org/10.1016/J.IJDEVNEU.2017.03.007
- Blaze, J., Scheuing, L., & Roth, T. L. (2013). Differential methylation of genes in the medial prefrontal cortex of developing and adult rats following exposure to maltreatment or nurturing care during infancy. Developmental Neuroscience, 35(4), 306–316. https://doi.org/10.1159/000350716
- Blaze, J., Wang, J., Ho, L., Mendelev, N., Haghighi, F., & Pasinetti, G. M. (2018). Polyphenolic compounds alter stress-induced patterns of global DNA methylation in brain and blood. Molecular Nutrition & Food Research, 62(8), 1700722. https://doi.org/10.1002/mnfr.201700722
- Boccia, M. L., Razzoli, M., Prasad Vadlamudi, S., Trumbull, W., Caleffie, C., & Pedersen, C. A. (2007). Repeated long separations from pups produce depression-like behavior in rat mothers. Psychoneuroendocrinology, 32(1), 65–71. https://doi.org/10.1016/J.PSYNEUEN.2006.10.004
- Bock, J., Poeschel, J., Schindler, J., Börner, F., Shachar-Dadon, A., Ferdman, N., Gaisler-Salomon, I., Leshem, M., Braun, K., & Poeggel, G. (2016). Transgenerational sex-specific impact of preconception stress on the development of dendritic spines and dendritic length in the medial prefrontal cortex. Brain Structure & Function, 221(2), 855–863. https://doi.org/10.1007/s00429-014-0940-4
- Boersma, G. J., Lee, R. S., Cordner, Z. A., Ewald, E. R., Purcell, R. H., Moghadam, A. A., & Tamashiro, K. L. (2014). Prenatal stress decreases Bdnf expression and increases methylation of Bdnf exon IV in rats. Epigenetics, 9(3), 437–447. 9, 437–447. https://doi.org/10.4161/epi.27558
- Brenhouse, H. C., & Andersen, S. L. (2011). Developmental trajectories during adolescence in males and females: A cross-species understanding of underlying brain changes. Neuroscience and Biobehavioral Reviews, 35(8), 1687–1703. https://doi.org/10.1016/J.NEUBIOREV.2011.04.013
- Brown, A., Fiori, L. M., & Turecki, G. (2019). Bridging basic and clinical research in early life adversity, DNA methylation, and major depressive disorder. Frontiers in Genetics, 10, 229. https://doi.org/10.3389/FGENE.2019.00229/XML/NLM
- Budziszewska, B., Jaworska-Feil, L., Tetich, M., Basta-Kaim, A., Kubera, M., Leśkiewicz, M., & Lasoń, W. (2004). Regulation of the human corticotropin-releasing-hormone gene promoter activity by antidepressant drugs in Neuro-2A and AtT-20 cells. Neuropsychopharmacology : Official Publication of the American College of Neuropsychopharmacology, 29(4), 785–794. https://doi.org/10.1038/SJ.NPP.1300379
- Cameron, N. M., Champagne, F. A., Parent, C., Fish, E. W., Ozaki-Kuroda, K., & Meaney, M. J. (2005). The programming of individual differences in defensive responses and reproductive strategies in the rat through variations in maternal care. Neuroscience and Biobehavioral Reviews, 29(4-5), 843–865. https://doi.org/10.1016/J.NEUBIOREV.2005.03.022
- Champagne, F. A., Francis, D. D., Mar, A., & Meaney, M. J. (2003). Variations in maternal care in the rat as a mediating influence for the effects of environment on development. Physiology & Behavior, 79(3), 359–371. https://doi.org/10.1016/S0031-9384(03)00149-5
- Champagne, F. A., & Meaney, M. J. (2006). Stress during gestation alters postpartum maternal care and the development of the offspring in a rodent model. Biological Psychiatry, 59(12), 1227–1235. https://doi.org/10.1016/J.BIOPSYCH.2005.10.016
- Choi, K. W., Denckla, C. A., Hoffman, N., Budree, S., Goddard, L., Zar, H. J., Stern, M., & Stein, D. J. (2022). Influence of Maternal Childhood Trauma on Perinatal Depression, Observed Mother-Infant Interactions, and Child Growth. Maternal and Child Health Journal, 26(8), 1649–1656. https://doi.org/10.1007/S10995-022-03417-2
- Coley, E. J. L., Demaestri, C., Ganguly, P., Honeycutt, J. A., Peterzell, S., Rose, N., Ahmed, N., Holschbach, M., Trivedi, M., & Brenhouse, H. C. (2019). Cross-generational transmission of early life stress effects on HPA regulators and bdnf are mediated by sex, lineage, and upbringing. Frontiers in Behavioral Neuroscience, 13, 101. https://doi.org/10.3389/FNBEH.2019.00101/FULL
- Constantinof, A., Boureau, L., Moisiadis, V. G., Kostaki, A., Szyf, M., & Matthews, S. G. (2019). Prenatal glucocorticoid exposure results in changes in gene transcription and DNA methylation in the female juvenile guinea pig hippocampus across three generations. Scientific Reports, 9(1), 1–12. https://doi.org/10.1038/s41598-019-54456-9
- Council, N. R. (2010). Guide for the Care and Use of Laboratory Animals. Eighth Edition. Guide for the Care and Use of Laboratory Animals. https://doi.org/10.17226/12910
- Couto-Pereira, N., de, S., Ferreira, C. F., Lampert, C., Arcego, D. M., Toniazzo, A. P., Bernardi, J. R., da Silva, D. C., von Poser Toigo, E., Diehl, L. A., Krolow, R., Silveira, P. P., & Dalmaz, C. (2016). Neonatal interventions differently affect maternal care quality and have sexually dimorphic developmental effects on corticosterone secretion. International Journal of Developmental Neuroscience : The Official Journal of the International Society for Developmental Neuroscience, 55, 72–81. https://doi.org/10.1016/J.IJDEVNEU.2016.10.001
- Cunningham, M. G., Bhattacharyya, S., & Benes, F. M. (2002). Amygdalo-cortical sprouting continues into early adulthood: Implications for the development of normal and abnormal function during adolescence. The Journal of Comparative Neurology, 453(2), 116–130. https://doi.org/10.1002/CNE.10376
- Curley, J. P., & Champagne, F. A. (2016). Influence of maternal care on the developing brain: Mechanisms, temporal dynamics and sensitive periods. Frontiers in Neuroendocrinology, 40, 52–66. https://doi.org/10.1016/J.YFRNE.2015.11.001
- Cutuli, D., Caporali, P., Gelfo, F., Angelucci, F., Laricchiuta, D., Foti, F., de Bartolo, P., Bisicchia, E., Molinari, M., Vecchioli, S. F., & Petrosini, L. (2015). Pre-reproductive maternal enrichment influences rat maternal care and offspring developmental trajectories: Behavioral performances and neuroplasticity correlates. Frontiers in Behavioral Neuroscience, 9, 66. https://doi.org/10.3389/FNBEH.2015.00066/ABSTRACT
- Day, J. J., & Sweatt, J. D. (2010). DNA methylation and memory formation. Nature Neuroscience, 13(11), 1319–1323. https://doi.org/10.1038/NN.2666
- Deshpande, S. S., Nemani, H., Arumugam, G., Ravichandran, A., & Balasinor, N. H. (2020). High-fat diet-induced and genetically inherited obesity differentially alters DNA methylation profile in the germline of adult male rats. Clinical Epigenetics, 12(1), 21. https://doi.org/10.1186/S13148-020-00974-7/FIGURES/8
- Dias, B. G., & Ressler, K. J. (2014). Parental olfactory experience influences behavior and neural structure in subsequent generations. Nature Neuroscience, 17(1), 89–96. https://doi.org/10.1038/nn.3594
- Doherty, T. S., Forster, A., & Roth, T. L. (2016). Global and gene-specific DNA methylation alterations in the adolescent amygdala and hippocampus in an animal model of caregiver maltreatment. Behavioural Brain Research, 298(Pt A), 55–61. https://doi.org/10.1016/J.BBR.2015.05.028
- Feng, J., & Fan, G. (2009). The role of DNA methylation in the central nervous system and neuropsychiatric disorders. Int Rev Neurobiol, 89, 67–84. https://doi.org/10.1016/S0074-7742(09)89004-1
- Fleming, A. S., & Rosenblatt, J. S. (1974). Maternal behavior in the virgin and lactating rat. Journal of Comparative and Physiological Psychology, 86(5), 957–972. https://doi.org/10.1037/H0036414
- Francis, D., Diorio, J., Liu, D., & Meaney, M. J. (1999). Nongenomic transmission across generations of maternal behavior and stress responses in the rat. Science, 286(5442), 1155–1158. 1979) https://doi.org/10.1126/SCIENCE.286.5442.1155/ASSET/605B2B0B-68C5-421F-87C2-68E1A0590418/ASSETS/GRAPHIC/SE449797302A.JPEG
- Franklin, T. B., Linder, N., Russig, H., Thöny, B., & Mansuy, I. M. (2011). Influence of early stress on social abilities and serotonergic functions across generations in mice. PLOS One, 6(7), e21842. https://doi.org/10.1371/JOURNAL.PONE.0021842
- Franklin, T. B., Russig, H., Weiss, I. C., Gräff, J., Linder, N., Michalon, A., Vizi, S., & Mansuy, I. M. (2010). Epigenetic transmission of the impact of early stress across generations. Biological Psychiatry, 68(5), 408–415., https://doi.org/10.1016/J.BIOPSYCH.2010.05.036
- Fries, G. R., Li, Q., McAlpin, B., Rein, T., Walss-Bass, C., Soares, J. C., & Quevedo, J. (2016). The role of DNA methylation in the pathophysiology and treatment of bipolar disorder. Neuroscience and Biobehavioral Reviews, 68, 474–488. https://doi.org/10.1016/J.NEUBIOREV.2016.06.010
- Gapp, K., Bohacek, J., Grossmann, J., Brunner, A. M., Manuella, F., Nanni, P., & Mansuy, I. M. (2016). Potential of environmental enrichment to prevent transgenerational effects of paternal trauma. Neuropsychopharmacology : Official Publication of the American College of Neuropsychopharmacology, 41(11), 2749–2758. https://doi.org/10.1038/npp.2016.87
- Gapp, K., Soldado-Magraner, S., Alvarez-Sánchez, M., Bohacek, J., Vernaz, G., Shu, H., Franklin, T. B., Wolfer, D., & Mansuy, I. M. (2014). Early life stress in fathers improves behavioural flexibility in their offspring. Nature Communications, 5(1), 5466. https://doi.org/10.1038/ncomms6466
- García-Cabezas, M. Á., & Zikopoulos, B. (2019). Evolution, development, and organization of the cortical connectome. PLoS Biology, 17(5), e3000259. https://doi.org/10.1371/JOURNAL.PBIO.3000259
- Gemmel, M., Harmeyer, D., Bögi, E., Fillet, M., Hill, L. A., Hammond, G. L., Charlier, T. D., & Pawluski, J. L. (2018). Perinatal fluoxetine increases hippocampal neurogenesis and reverses the lasting effects of pre-gestational stress on serum corticosterone, but not on maternal behavior, in the rat dam. Behavioural Brain Research, 339, 222–231. https://doi.org/10.1016/J.BBR.2017.11.038
- Gemmel, M., Rayen, I., van Donkelaar, E., Loftus, T., Steinbusch, H. W., Kokras, N., Dalla, C., & Pawluski, J. L. (2016). Gestational stress and fluoxetine treatment differentially affect plasticity, methylation and serotonin levels in the PFC and hippocampus of rat dams. Neuroscience, 327, 32–43. https://doi.org/10.1016/J.NEUROSCIENCE.2016.03.068
- Goren, S., & Dekel, N. (1994). Maintenance of meiotic arrest by a phosphorylated p34cdc2 is independent of cyclic adenosine 3′,5′-monophosphate. Biology of Reproduction, 51(5), 956–962. https://doi.org/10.1095/BIOLREPROD51.5.956
- Gu, T. P., Guo, F., Yang, H., Wu, H. P., Xu, G. F., Liu, W., Xie, Z. G., Shi, L., He, X., Jin, S. G., Iqbal, K., Shi, Y. G., Deng, Z., Szabó, P. E., Pfeifer, G. P., Li, J., & Xu, G. L. (2011). The role of Tet3 DNA dioxygenase in epigenetic reprogramming by oocytes. Nature, 477(7366), 606–610. https://doi.org/10.1038/NATURE10443
- Hua, L., Chen, W., Meng, Y., Qin, M., Yan, Z., Yang, R., Liu, Q., Wei, Y., Zhao, Y., Yan, L., & Qiao, J. (2022). The combination of DNA methylome and transcriptome revealed the intergenerational inheritance on the influence of advanced maternal age. Clinical and Translational Medicine, 12(9), e990. https://doi.org/10.1002/ctm2.990
- Hummel, M., Cummons, T., Lu, P., Mark, L., Harrison, J. E., Kennedy, J. D., & Whiteside, G. T. (2010). Pain is a salient “stressor” that is mediated by corticotropin-releasing factor-1 receptors. Neuropharmacology, 59(3), 160–166. https://doi.org/10.1016/J.NEUROPHARM.2010.05.001
- Huntriss, J., Hinkins, M., Oliver, B., Harris, S. E., Beazley, J. C., Rutherford, A. J., Gosden, R. G., Lanzendorf, S. E., & Picton, H. M. (2004). Expression of mRNAs for DNA methyltransferases and methyl-CpG-binding proteins in the human female germ line, preimplantation embryos, and embryonic stem cells. Molecular Reproduction and Development, 67(3), 323–336. https://doi.org/10.1002/MRD.20030
- John, R. M. (2019). Prenatal adversity modulates the quality of maternal care via the exposed offspring. bioessays, 41(6), 1900025. https://doi.org/10.1002/bies.201900025
- Kajta, M., Wnuk, A., Rzemieniec, J., Lason, W., Mackowiak, M., Chwastek, E., Staniszewska, M., Nehring, I., & Wojtowicz, A. K. (2019). Triclocarban disrupts the epigenetic status of neuronal cells and induces AHR/CAR-mediated apoptosis. Molecular Neurobiology, 56(5), 3113–3131. https://doi.org/10.1007/S12035-018-1285-4/FIGURES/11
- Kiryanova, V., Meunier, S. J., Vecchiarelli, H. A., Hill, M. N., & Dyck, R. H. (2016). Effects of maternal stress and perinatal fluoxetine exposure on behavioral outcomes of adult male offspring. Neuroscience, 320, 281–296. https://doi.org/10.1016/J.NEUROSCIENCE.2016.01.064
- Kobayashi, H., Sakurai, T., Imai, M., Takahashi, N., Fukuda, A., Yayoi, O., Sato, S., Nakabayashi, K., Hata, K., Sotomaru, Y., Suzuki, Y., & Kono, T. (2012). Contribution of intragenic DNA methylation in mouse gametic DNA methylomes to establish oocyte-specific heritable marks. PLOS Genetics, 8(1), e1002440. https://doi.org/10.1371/JOURNAL.PGEN.1002440
- Kosten, T. A., & Kehoe, P. (2010). Immediate and enduring effects of neonatal isolation on maternal behavior in rats. International Journal of Developmental Neuroscience : The Official Journal of the International Society for Developmental Neuroscience, 28(1), 53–61. https://doi.org/10.1016/J.IJDEVNEU.2009.09.005
- Kott, J. M., Mooney-Leber, S. M., Li, J., & Brummelte, S. (2018). Elevated stress hormone levels and antidepressant treatment starting before pregnancy affect maternal care and litter characteristics in an animal model of depression. Behavioural Brain Research, 348, 101–114. https://doi.org/10.1016/J.BBR.2018.04.006
- Lauby, S. C., Fleming, A. S., & McGowan, P. O. (2021). Beyond maternal care: The effects of extra-maternal influences within the maternal environment on offspring neurodevelopment and later-life behavior. Neuroscience and Biobehavioral Reviews, 127, 492–501. https://doi.org/10.1016/J.NEUBIOREV.2021.04.021
- Lee, H. J., Kim, J. W., Yim, S. v., Kim, M. J., Kim, S. A., Kim, Y. J., Kim, C. J., & Chung, J. H. (2001). Fluoxetine enhances cell proliferation and prevents apoptosis in dentate gyrus of maternally separated rats. Molecular Psychiatry, 6(6), 725–728. https://doi.org/10.1038/sj.mp.4000947
- Lei, L., Wu, X., Gu, H., Ji, M., & Yang, J. (2020). Differences in DNA methylation reprogramming underlie the sexual dimorphism of behavioral disorder caused by prenatal stress in rats. Frontiers in Neuroscience, 14, 573107. https://doi.org/10.3389/FNINS.2020.573107
- Liu, D., Diorio, J., Day, J. C., Francis, D. D., & Meaney, M. J. (2000). Maternal care, hippocampal synaptogenesis and cognitive development in rats. Nature Neuroscience, 3(8)2000, 799–806. 8 3, https://doi.org/10.1038/77702
- Livak, K. J., & Schmittgen, T. D. (2001). Analysis of relative gene expression data using real-time quantitative PCR and the 2 − ΔΔCT method. Methods), 25(4), 402–408. https://doi.org/10.1006/METH.2001.1262
- McGowan, P. O., Suderman, M., Sasaki, A., Huang, T. C. T., Hallett, M., Meaney, M. J., & Szyf, M. (2011). Broad epigenetic signature of maternal care in the brain of adult rats. PLOS One,.6(2), e14739. https://doi.org/10.1371/journal.pone.0014739
- McLeod, J., Sinal, C. J., & Perrot-Sinal, T. S. (2007). Evidence for non-genomic transmission of ecological information via maternal behavior in female rats. Genes, Brain, and Behavior, 6(1), 19–29. https://doi.org/10.1111/J.1601-183X.2006.00214.X
- Miller, C. A., & Sweatt, J. D. (2007). Covalent modification of DNA regulates memory formation. Neuron, 53(6), 857–869. https://doi.org/10.1016/J.NEURON.2007.02.022
- Millstein, R. A., & Holmes, A. (2007). Effects of repeated maternal separation on anxiety- and depression-related phenotypes in different mouse strains. Neuroscience and Biobehavioral Reviews, 31(1), 3–17. https://doi.org/10.1016/J.NEUBIOREV.2006.05.003
- Moore, L. D., Le, T., & Fan, G. (2013). DNA methylation and its basic function. Neuropsychopharmacology : Official Publication of the American College of Neuropsychopharmacology, 2013 38(1)38, 23–38. https://doi.org/10.1038/npp.2012.112
- Mueller, B. R., & Bale, T. L. (2008). Sex-Specific Programming of Offspring Emotionality after Stress Early in Pregnancy. The Journal of Neuroscience : The Official Journal of the Society for Neuroscience, 28(36), 9055–9065. https://doi.org/10.1523/JNEUROSCI.1424-08.2008
- Mychasiuk, R., Harker, A., Ilnytskyy, S., & Gibb, R. (2013). Paternal stress prior to conception alters DNA methylation and behaviour of developing rat offspring. Neuroscience, 241, 100–105. https://doi.org/10.1016/J.NEUROSCIENCE.2013.03.025
- Mychasiuk, R., Muhammad, A., & Kolb, B. (2016). Chronic stress induces persistent changes in global DNA methylation and gene expression in the medial prefrontal cortex, orbitofrontal cortex, and hippocampus. Neuroscience, 322, 489–499. https://doi.org/10.1016/J.NEUROSCIENCE.2016.02.053
- Myers, M. M., Brunelli, S. A., Squire, J. M., Shindeldecker, R. D., & Hofer, M. A. (1989). Maternal behavior of SHR rats and its relationship to offspring blood pressures. Developmental Psychobiology, 22(1), 29–53. https://doi.org/10.1002/DEV.420220104
- Núñez, M. J., Novío, S., Suárez, J. A., Balboa, J., & Freire-Garabal, M. (2010). Effects of psychological stress and fluoxetine on development of oral candidiasis in rats. Clinical and Vaccine Immunology, 17(4), 668–673. https://doi.org/10.1128/CVI.00380-09
- Palma-Gudiel, H., Córdova-Palomera, A., Leza, J. C., & Fañanás, L. (2015). Glucocorticoid receptor gene (NR3C1) methylation processes as mediators of early adversity in stress-related disorders causality: A critical review. Neuroscience and Biobehavioral Reviews, 55, 520–535. https://doi.org/10.1016/J.NEUBIOREV.2015.05.016
- Pan, P., Fleming, A. S., Lawson, D., Jenkins, J. M., & McGowan, P. O. (2014). Within- and between-litter maternal care alter behavior and gene regulation in female offspring. Behavioral Neuroscience, 128(6), 736–748. https://doi.org/10.1037/BNE0000014
- Pawluski, J. L., Charlier, T. D., Fillet, M., Houbart, V., Crispin, H. T., Steinbusch, H. W., & van den Hove, D. L. (2012). Chronic fluoxetine treatment and maternal adversity differentially alter neurobehavioral outcomes in the rat dam. Behavioural Brain Research, 228(1), 159–168. https://doi.org/10.1016/J.BBR.2011.11.043
- Pawluski, J. L., Rayen, I., Niessen, N. A., Kristensen, S., van Donkelaar, E. L., Balthazart, J., Steinbusch, H. W., & Charlier, T. D. (2012). Developmental fluoxetine exposure differentially alters central and peripheral measures of the HPA system in adolescent male and female offspring. Neuroscience, 220, 131–141. https://doi.org/10.1016/J.NEUROSCIENCE.2012.06.034
- Paxinos, G., & Watson, C. (2006). The rat brain in stereotaxic coordinates. 6th Edition. Academic Press.
- Petrussa, L., van de Velde, H., & de Rycke, M. (2014). Dynamic regulation of DNA methyltransferases in human oocytes and preimplantation embryos after assisted reproductive technologies. Molecular Human Reproduction, 20(9), 861–874. https://doi.org/10.1093/MOLEHR/GAU049
- Pfaffl, M. W., Tichopad, A., Prgomet, C., & Neuvians, T. P. (2004). Determination of stable housekeeping genes, differentially regulated target genes and sample integrity: BestKeeper – excel-based tool using pair-wise correlations. Biotechnology Letters, 26(6)26, 509–515. https://doi.org/10.1023/B:BILE.0000019559.84305.47
- Pisu, M. G., Boero, G., Biggio, F., Garau, A., Corda, D., Congiu, M., Concas, A., Porcu, P., & Serra, M. (2017). Juvenile social isolation affects maternal care in rats: Involvement of allopregnanolone. Psychopharmacology, 234(17), 2587–2596. https://doi.org/10.1007/S00213-017-4661-2/FIGURES/5
- Plank, A. C., Frey, S., Basedow, L. A., Solati, J., Canneva, F., von Hörsten, S., Kratz, O., Moll, G. H., & Golub, Y. (2021). Prenatally traumatized mice reveal hippocampal methylation and expression changes of the stress-related genes Crhr1 and Fkbp5. Translational Psychiatry, 11(1), 10. https://doi.org/10.1038/s41398-021-01293-y
- Pries, L. K., Gülöksüz, S., & Kenis, G. (2017). DNA methylation in schizophrenia. Adv Exp Med Biol, 978, 211–236. https://doi.org/10.1007/978-3-319-53889-1_12/TABLES/2
- Radford, E. J., Ito, M., Shi, H., Corish, J. A., Yamazawa, K., Isganaitis, E., Seisenberger, S., Hore, T. A., Reik, W., Erkek, S., Peters, A., Patti, M. E., & Ferguson-Smith, A. C. (2014). In utero effects. In utero undernourishment perturbs the adult sperm methylome and intergenerational metabolism. Science , 345(6198), 1255903. https://doi.org/10.1126/SCIENCE.1255903
- Raudenbush, S. W., & Bryk, A. S. (2002). Hierarchical linear models : Applications and data analysis methods. 485.
- Rayen, I., van den Hove, D. L., Prickaerts, J., Steinbusch, H. W., & Pawluski, J. L. (2011). Fluoxetine during development reverses the effects of prenatal stress on depressive-like behavior and hippocampal neurogenesis in adolescence. PLOS One,.6(9), e24003. https://doi.org/10.1371/journal.pone.0024003
- Rodrigues, G. M., Toffoli, L. v., Manfredo, M. H., Francis-Oliveira, J., Silva, A. S., Raquel, H. A., Martins-Pinge, M. C., Moreira, E. G., Fernandes, K. B., Pelosi, G. G., & Gomes, M. v (2015). Acute stress affects the global DNA methylation profile in rat brain: Modulation by physical exercise. Behavioural Brain Research, 279, 123–128. https://doi.org/10.1016/J.BBR.2014.11.023
- Romeo, R. D. (2017). The impact of stress on the structure of the adolescent brain: Implications for adolescent mental health. Brain Research, 1654(Pt B), 185–191. https://doi.org/10.1016/J.BRAINRES.2016.03.021
- Roth, T. L., Lubin, F. D., Funk, A. J., & Sweatt, J. D. (2009). Lasting epigenetic influence of early-life adversity on the Bdnf gene. Biological Psychiatry, 65(9), 760–769. https://doi.org/10.1016/J.BIOPSYCH.2008.11.028
- Roth, T. L., Matt, S., Chen, K., & Blaze, J. (2014). Bdnf DNA methylation modifications in the hippocampus and amygdala of male and female rats exposed to different caregiving environments outside the homecage. Developmental Psychobiology, 56(8), 1755–1763. https://doi.org/10.1002/DEV.21218
- Roth, T. L., & Sullivan, R. M. (2005). Memory of early maltreatment: Neonatal behavioral and neural correlates of maternal maltreatment within the context of classical conditioning. Biological Psychiatry, 57(8), 823–831. https://doi.org/10.1016/J.BIOPSYCH.2005.01.032
- Seisenberger, S., Andrews, S., Krueger, F., Arand, J., Walter, J., Santos, F., Popp, C., Thienpont, B., Dean, W., & Reik, W. (2012). The dynamics of genome-wide DNA methylation reprogramming in mouse primordial germ cells. Molecular Cell, 48(6), 849–862. https://doi.org/10.1016/J.MOLCEL.2012.11.001
- Sengupta, P. (2013). The laboratory rat: Relating its age with human’s. Int J Prev Med, 4, 624.
- Shachar-Dadon, A., Schulkin, J., & Leshem, M. (2009). Adversity before conception will affect adult progeny in rats. Developmental Psychology, 45(1), 9–16. https://doi.org/10.1037/A0014030
- Shkolnik, K., Tadmor, A., Ben-Dor, S., Nevo, N., Galiani, D., & Dekel, N. (2011). Reactive oxygen species are indispensable in ovulation. Proceedings of the National Academy of Sciences of the United States of America, 108(4), 1462–1467. https://doi.org/10.1073/PNAS.1017213108
- Stamatakis, A., Kalpachidou, T., Raftogianni, A., Zografou, E., Tzanou, A., Pondiki, S., & Stylianopoulou, F. (2015). Rat dams exposed repeatedly to a daily brief separation from the pups exhibit increased maternal behavior, decreased anxiety and altered levels of receptors for estrogens (ERα, ERβ), oxytocin and serotonin (5-HT1A) in their brain. Psychoneuroendocrinology, 52, 212–228. https://doi.org/10.1016/J.PSYNEUEN.2014.11.016
- St-Cyr, S., Abuaish, S., Sivanathan, S., & McGowan, P. O. (2017). Maternal programming of sex-specific responses to predator odor stress in adult rats. Hormones and Behavior, 94, 1–12. https://doi.org/10.1016/J.YHBEH.2017.06.005
- Steenwyk, G., van, K., Gapp, A., Jawaid, P.-L., Germain, F., Manuella, D. K., Tanwar, N., Zamboni, N., Gaur, A., Efimova, K. M., Thumfart, E. A., Miska, I., & Mansuy, M. (2020). Involvement of circulating factors in the transmission of paternal experiences through the germline. The EMBO Journal, 39(23), e104579. https://doi.org/10.15252/EMBJ.2020104579
- Sterrenburg, L., Gaszner, B., Boerrigter, J., Santbergen, L., Bramini, M., Elliott, E., Chen, A., Peeters, B., Roubos, E. W., & Kozicz, T. (2011). Chronic stress induces sex-specific alterations in methylation and expression of corticotropin-releasing factor gene in the rat. PLOS One,.6(11), e28128. https://doi.org/10.1371/journal.pone.0028128
- Tang, W. W. C., Dietmann, S., Irie, N., Leitch, H. G., Floros, V. I., Bradshaw, C. R., Hackett, J. A., Chinnery, P. F., & Surani, M. A. (2015). A unique gene regulatory network resets the human germline epigenome for development. Cell, 161(6), 1453–1467. https://doi.org/10.1016/J.CELL.2015.04.053
- Thomassin, H., Flavin, M., Espinás, M. L., & Grange, T. (2001). Glucocorticoid-induced DNA demethylation and gene memory during development. The EMBO Journal, 20(8), 1974–1983. https://doi.org/10.1093/EMBOJ/20.8.1974
- Tottenham, N., & Galván, A. (2016). Stress and the adolescent brain: Amygdala-prefrontal cortex circuitry and ventral striatum as developmental targets. Neuroscience and Biobehavioral Reviews, 70, 217–227. https://doi.org/10.1016/J.NEUBIOREV.2016.07.030
- van der Doelen, R. H. A., Arnoldussen, I. A., Ghareh, H., van Och, L., Homberg, J. R., & Kozicz, T. (2015). Early life adversity and serotonin transporter gene variation interact to affect DNA methylation of the corticotropin-releasing factor gene promoter region in the adult rat brain. Development and Psychopathology, 27(1), 123–135. https://doi.org/10.1017/S0954579414001345
- van Hasselt, F. N., Tieskens, J. M., Trezza, V., Krugers, H. J., Vanderschuren, L., & Joëls, M. (2012). Within-litter variation in maternal care received by individual pups correlates with adolescent social play behavior in male rats. Physiology & Behavior, 106(5), 701–706. https://doi.org/10.1016/J.PHYSBEH.2011.12.007
- van Steenwyk, G., Roszkowski, M., Manuella, F., Franklin, T. B., & Mansuy, I. M. (2018). Transgenerational inheritance of behavioral and metabolic effects of paternal exposure to traumatic stress in early postnatal life: Evidence in the 4th generation. Environmental Epigenetics, 4(2), dvy023. https://doi.org/10.1093/EEP/DVY023
- Weaver, I. C. G., Cervoni, N., Champagne, F. A., D’Alessio, A. C., Sharma, S., Seckl, J. R., Dymov, S., Szyf, M., & Meaney, M. J. (2004). Epigenetic programming by maternal behavior. Nature Neuroscience, 7(8), 847–854. https://doi.org/10.1038/NN1276
- Wnuk, A., Przepiórska, K., Pietrzak, B. A., & Kajta, M. (2021). Post-treatment with amorfrutin B evokes PPARγ-mediated neuroprotection against hypoxia and ischemia. Biomedicines, 9(8)2021, 854. Page 854 9, https://doi.org/10.3390/biomedicines9080854
- Yehuda, R., Daskalakis, N. P., Lehrner, A., Desarnaud, F., Bader, H. N., Makotkine, I., Flory, J. D., Bierer, L. M., & Meaney, M. J. (2014). Influences of maternal and paternal PTSD on epigenetic regulation of the glucocorticoid receptor gene in Holocaust survivor offspring. The American Journal of Psychiatry, 171(8), 872–880. https://doi.org/10.1176/APPI.AJP.2014.13121571
- Zaidan, H., & Gaisler-Salomon, I. (2015). Prereproductive stress in adolescent female rats affects behavior and corticosterone levels in second-generation offspring. Psychoneuroendocrinology, 58, 120–129. https://doi.org/10.1016/j.psyneuen.2015.04.013
- Zaidan, H., Galiani, D., & Gaisler-Salomon, I. (2021). Pre-reproductive stress in adolescent female rats alters oocyte microRNA expression and offspring phenotypes: pharmacological interventions and putative mechanisms. Translational Psychiatry, 11(1), 113. https://doi.org/10.1038/s41398-021-01220-1
- Zaidan, H., Leshem, M., & Gaisler-Salomon, I. (2013). Prereproductive stress to female rats alters corticotropin releasing factor type 1 expression in ova and behavior and brain corticotropin releasing factor type 1 expression in offspring. Biological Psychiatry, 74(9), 680–687. https://doi.org/10.1016/j.biopsych.2013.04.014
- Zaidan, H., Ramaswami, G., Golumbic, Y. N., Sher, N., Malik, A., Barak, M., Galiani, D., Dekel, N., Li, J. B., & Gaisler-Salomon, I. (2018). A-to-I RNA editing in the rat brain is age-dependent, region-specific and sensitive to environmental stress across generations. BMC Genomics,.19(1), 1–16. https://doi.org/10.1186/s12864-017-4409-8
- Zhang, T. Y., Hellstrom, I. C., Bagot, R. C., Wen, X., Diorio, J., & Meaney, M. J. (2010). Maternal care and DNA methylation of a glutamic acid decarboxylase 1 promoter in rat hippocampus. The Journal of Neuroscience, 30(39), 13130–13137. https://doi.org/10.1523/JNEUROSCI.1039-10.2010
- Zheng, X., Li, Z., Wang, G., Wang, H., Zhou, Y., Zhao, X., Cheng, C. Y., Qiao, Y., & Sun, F. (2021). Sperm epigenetic alterations contribute to inter- and transgenerational effects of paternal exposure to long-term psychological stress via evading offspring embryonic reprogramming. Cell Discovery, 7(1), 101. https://doi.org/10.1038/s41421-021-00343-5