Abstract
The lysine 63 deubiquitinase cylindromatosis (CYLD) is expressed at high levels in the brain and is considered to be involved in anxious and depressive behavior, cognitive inflexibility, and autism disorders. Previous research was limited in some brain regions, including the hippocampus, striatum, and amygdala. To better understand whether CYLD plays a role in adaptation to stress and which brain regions are involved, we analyzed the behavior of CYLD-knockout mice in the elevated plus maze (EPM) and light-dark box test (LDT) after acute restraint stress (ARS) and mapped their c-Fos immunoreactivity in brain sections. Here we report that CYLD deficiency leads to an unexpected reaction to ARS in mice, and is accompanied by significant neuronal activation of brain regions including the medial prefrontal cortex (mPFC), dorsal striatum (DS), nucleus accumbens (NAc), and basal lateral amygdala (BLA), but not ventral hippocampus (vHPC). Our findings show that CYLD participates in ARS-induced anxious behavior and that this involves multiple brain regions.
1. Introduction
Cylindromatosis (CYLD), a lysine-63 (K63) deubiquitinating enzyme that is also known to be a tumor suppressor, is involved in the nuclear factor kappa B (NF-κB) signaling pathway, which mediates the immune response, inflammation, and apoptosis (Kovalenko et al., Citation2003; Sun, Citation2010; Trompouki et al., Citation2003; Xu et al., Citation2016). Its loss-of-function mutations cause familial cylindromatosis (Bignell et al., Citation2000), while gain-of-function mutations cause frontotemporal dementia (Dobson-Stone et al., Citation2020) and amyotrophic lateral sclerosis (Tábuas-Pereira et al., Citation2020). It has been confirmed that CYLD is highly expressed in the brain (Jin et al., Citation2019; Mazarei et al., Citation2010), especially in the striatum, followed by the nucleus accumbens (NAc), amygdala, hypothalamus, prefrontal cortex (PFC) and hippocampus. Recent studies have shown that CYLD-knockout mice display anxiety-like behavior (Han et al., Citation2020), fear-memory deficit (Li et al., Citation2021), abnormal sociability, depression, cognitive inflexibility, and repetitive behavior (Colombo et al., Citation2021; Zajicek et al., Citation2022). It also has been discovered that CYLD is highly enriched in the postsynaptic density (PSD) (Ma et al., Citation2017), and the protein of CYLD is recruited to the PSD in response to Ca2+/calmodulin-dependent protein kinase II and N-methyl-D-aspartate (NMDA) receptor activation (Dosemeci et al., Citation2013; Thein et al., Citation2014; Zajicek & Yao, Citation2021). In addition, previous report suggested that CYLD positively regulates neuronal dendrite morphogenesis and spine formation in cultured mouse hippocampal neurons (Li et al., Citation2019) and has a role in neuronal activity and synaptic transmission in the basal lateral amygdala (BLA) of mice (Li et al., Citation2021). These findings may result from the abnormal dendritic pruning caused by CYLD via the Akt-mTOR-autophagy signaling pathway (Colombo et al., Citation2021; Zajicek et al., Citation2022).
Stress can lead to multiple adaptive processes across several regions of the brain, including mPFC, amygdala, and hippocampus (Joffe et al., 2022; Han et al., Citation2015; Godar et al., Citation2015), which result in emotional responses such as anxiety or depression (Liu et al., Citation2019; Zhou et al., Citation2019). Acute restraint stress (ARS) is an appropriate means of inducing stress in laboratory rodents, and has been shown to suppress Akt activity (Jin et al., Citation2015) and to promote inflammation via NF-κB-driven proinflammatory pathways (MacDowell et al., Citation2014). Rats are subjected to ARS and produce emergency changes in dendritic branches in BLA (Novaes et al., Citation2018) and exhibit anxiety-like behavior by decreasing gamma-aminobutyric acid (GABA) release, leading to reduced activation of GABAA receptors in zebrafish (Assad et al., Citation2020). Various types of abnormal behavior have been reported in CYLD-knockout mice over the past two years (Colombo et al., Citation2021; Han et al., Citation2020; Li et al., Citation2021; Zajicek et al., Citation2022). There is an interaction between genetic background and environment in the development of mental illness (Duncan et al., Citation2014). Therefore, we are interested to find out how the environmental challenges will affect CYLD-knockout mice.
In this study, we explored the impact of environmental challenges on Cyld−/− mice by evaluating whether and how CYLD-knockout mice respond to the acute challenge posed by a single episode of ARS. The elevated plus maze (EPM) test was employed to assess anxious behavior in CYLD-knockout mice following ARS. We also used c-Fos immunochemical staining to characterize activated brain areas in CYLD-knockout mice after ARS. It is upregulated soon after a stimulus is delivered, so c-Fos is known as an early response gene and shows activity-dependent expression; consequently, it has been widely used to identify stimulation-induced neuronal activation (Bullitt, Citation1990). Our data highlight several new brain regions for studying the role of CYLD in the central nervous system.
2. Material and methods
2.1. Animals
CYLD-knockout mice were generously gifted by Dr. Shao-cong Sun (University of Texas MD Anderson Cancer Center, Texas, USA). Cyld−/− mice and Cyld+/+ mice were generated by intercrossing Cyld± males and females and genotyped using PCR, as described previously (Reiley et al., Citation2007; Zhang et al., Citation2016). All animal procedures were approved by the Ethics Committee for Animal Research at South China Normal University, in accordance with the principles outlined in the National Institutes of Health Guide for the Care and Use of Laboratory Animals. Mice were housed (five mice per cage) in same-sex groups at room temperature (RT) (25 °C ± 1 °C) and relative humidity of 50%–60% with a 12/12-hour light-dark cycle and ad-libitum access to water and food. The experiments were undertaken when male and female mice were 8–12 weeks old.
2.2. Acute restraint stress (ARS)
The ARS protocol was performed as previously described (Tu et al., Citation2019). Briefly, mice were placed for 120 min in commercially available acrylic tubes (50 ml), with small openings on both sides. Following ARS, mice underwent EPM and LDT tests sequentially (shown in ).
Figure 1. CYLD deficiency leads to an unexpected reaction to acute restraint stress (ARS) in mice. The scheme shows the experimental design using ARS, behavioral test, and c-Fos immunohistochemistry (a). in the EPM, Cyld−/− +ARS mice spent significantly more time in the open arms (B) and made significantly more entries into the open arms (C). in the LDT, Cyld−/− +ARS mice spent significantly more time in the light box (D) and made significantly more transitions (E). Data are represented as mean ± SEM. *, p < .05; **, p < .01; n = 10 mice per group.
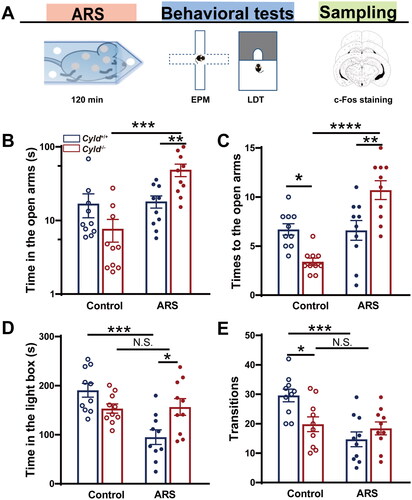
2.3. Elevated-plus maze (EPM) test
The EPM is widely used in rodents to assess the effect on the behavior of anti-anxiety pharmacological agents and to define brain regions that underly anxious behavior (Walf & Frye, Citation2007). The EPM apparatus is a white maze elevated 60 cm above the floor, with two open arms (30 × 5 cm), two closed arms (30 × 5 × 15 cm), and a central region (5 × 5 cm). Mice were placed in the central area facing the open arms and left to explore for 6 min, as previously described (Han et al., Citation2020). The movement trace of each mouse was analyzed for the number of entries to open and closed arms and for the time spent in both arms.
2.5. Light-dark box test (LDT)
The LDT apparatus (Anhui Zhenghua instruments) was 13 cm high and 30 cm long, divided into two boxes with a small doorway between them, which allowed mice to explore freely in both boxes. The light box was illuminated (390 lux) with an ordinary digital camera, and the dark box was non-illuminated (2 lux) but was imaged using an infrared camera. As previously described (Han et al., Citation2020), mice were placed in the dark box and the small doorway was opened after 3 s. Mice were allowed to move freely for 10 min and time spent in the light box and the number of transitions between boxes was automatically recorded. At the end of the experiment, the box was cleaned with alcohol to remove odor before the next test.
2.5. Immunohistochemistry
After the EPM and LTD test, mice were anesthetized and perfused transcardially with 4% paraformaldehyde, as previously described (Han et al., Citation2020). Then brains were removed, postfixed for 12 h in PFA, and dehydrated with 15% sucrose overnight and then with 30% sucrose until tissue no longer floated in the sucrose solution. Brain tissue was sectioned into 30 μm slices using a freezing microtome (Leica, Germany) and the slices were dried at 42 °C overnight. The slices were then treated with 0.5% Triton-X 100 for membrane permeabilization and blocked with 5% BSA in 0.01 M PBS for 1.5 h at RT, followed by incubation with primary antibody rabbit polyclonal anti-c-Fos antibody (1:1000, Abcam, ab190289) overnight at 4 °C. The following day, slices were washed with PBS, incubated with Cy3-labelled anti-rabbit secondary antibody (1:1000; Absin, abs20024) for one hour at RT, and then given three ten-minute washes in PBS. After washing, slices were incubated with DAPI solution (1:5 for 5 min, Beyotime, C1005) for nuclear labeling and then sequentially coverslipped with an anti-fade mounting medium. Images were photographed using the 200× objective lens of an EVOS fluorescence microscope (Invitrogen, USA). The microscope can stitch multiple 200× small images into a large image of the entire brain slice. The mouse brain atlas (Paxinos and Franklin, Citation2001) was used to identify the anatomical location of the several brain subregions known to be important in anxiety and stress responses (Calhoon & Tye, Citation2015), including the medial prefrontal cortex (mPFC), dorsal striatum (DS), nucleus accumbens (NAc), basal lateral amygdala (BLA) and ventral hippocampus (vHPC). Three sections (mPFC: bregma +1.5 to +2.0 mm; DS: bregma 0 to +1.5 mm; NAc: bregma +0.6 to +1.9 mm; BLA: bregma −0.8 to −2.0 mm; vHPC: bregma −2.8 to −3.5 mm) of every mouse were chosen (three brain slices are spaced the same distance from front to back). The number of c-Fos-positive cells was manually calculated within each region of interest area (light blue area of ) using the point tool of Image J software (National Institutes of Health, Bethesda, MD, USA).
Figure 2. Effect of ARS on c-Fos expression in the mPFC of Cyld−/− mice. Schematic of the mPFC between1.5 and 2.0 mm from the Bregma point. Light blue area: region of mPFC was counted the cell number; Black box: region of representative 200× small images (a). There were significantly more c-Fos-positive cells in the mPFC of Cyld−/− mice subjected to ARS (B). Representative insets show a magnified area within the mPFC of Cyld+/+ mice, Cyld−/− mice, Cyld+/+ +ARS mice, and Cyld−/− +ARS mice (C). Data are represented as mean ± SEM. *, p < .05, ****, p < .0001; control groups n = 6 slice/3 mice; ARS groups n = 10–12 slice/6 mice.
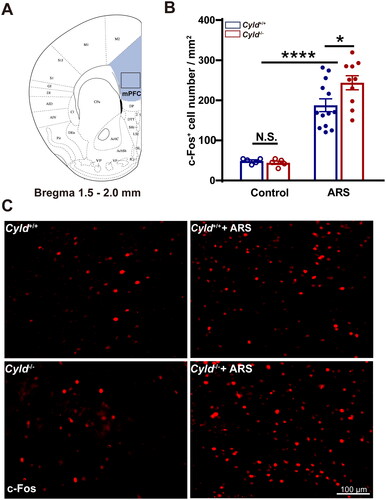
Figure 3. Effect of ARS on c-Fos expression in the DS of Cyld−/− mice. Schematic of the DS between 0 and 1.5 mm from the Bregma point. Light blue area: region of DS was counted the cell number; Black box: region of representative 200× images (a). There were significantly more c-Fos-positive cells in the DS of Cyld−/− mice subjected to ARS (B). Representative insets show a magnified area within the DS of Cyld+/+ mice, Cyld−/− mice, Cyld+/+ +ARS mice, and Cyld−/− +ARS mice (C). Data are represented as mean ± SEM. ****, p < .0001; control groups n = 6 slice/3 mice; ARS groups n = 15 slice/6 mice.
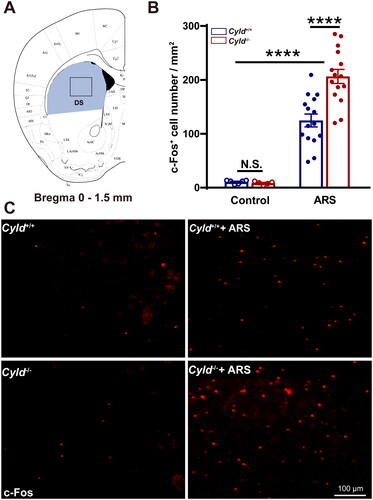
Figure 4. Effect of ARS on c-Fos expression in the NAc of Cyld−/− mice. Schematic of the NAc between 0.6 and 1.9 mm from the Bregma point. Light blue area: region of NAc was counted the cell number; Black box: region of representative 200× images (a). There were significantly more c-Fos-positive cells in the NAc of Cyld−/− mice subjected to ARS (B). Representative insets show a magnified area within the NAc of Cyld+/+ mice, Cyld−/− mice, Cyld+/+ +ARS mice, and Cyld−/− +ARS mice (C). Data are represented as mean ± SEM. ***, p < .001, ****, p < .0001; control groups n = 6 slice/3 mice; ARS groups n = 14 slice/6 mice.
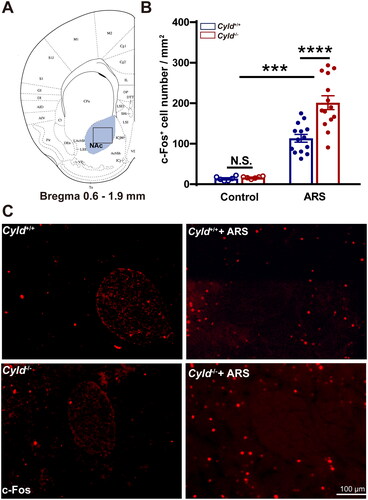
Figure 5. Effect of ARS on c-Fos expression in the BLA of Cyld−/− mice. Schematic of the BLA between −0.8 and −2.0 mm from the Bregma point. Light blue area: region of BLA was counted the cell number; Black box: region of representative 200× images (a). There were significantly more c-Fos-positive cells in the BLA of Cyld−/− mice subjected to ARS (B). Representative insets show a magnified area within the BLA of Cyld+/+ mice, Cyld−/− mice, Cyld+/+ +ARS mice, and Cyld−/− +ARS mice (C). Data are represented as mean ± SEM. *, p < .05, ****, p < .0001; control groups n = 6 slice/3 mice; ARS groups n = 10 slice/6 mice.
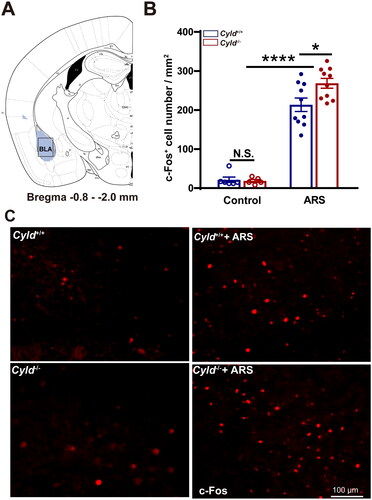
Figure 6. Effect of ARS on c-Fos expression in the vHPC of Cyld−/− mice. Schematic of the vHPC between −2.8 and −3.5 mm from the Bregma point. Light blue area: region of vHPC was counted the cell number; Black box: region of representative 200× images (A). There were significantly more c-Fos-positive cells in the vHPC of Cyld−/− mice subjected to ARS (B). Representative insets show a magnified area within the vHPC of Cyld+/+ mice, Cyld−/− mice, Cyld+/+ +ARS mice, and Cyld−/− +ARS mice (C). Data are represented as mean ± SEM. N.S., not significant, ****, p < .0001; control groups n = 6 slice/3 mice; ARS groups n = 10 slice/6 mice.
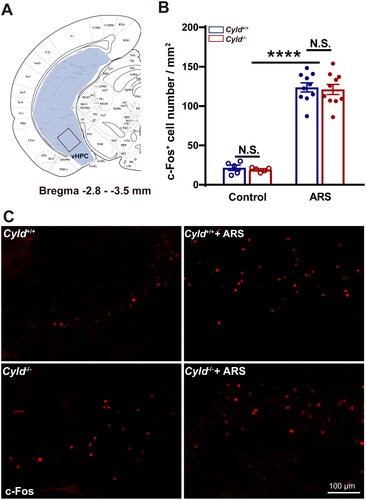
2.6. Statistical analysis
Prism 7.0 for Windows (GraphPad Software, USA) was employed for graphing and data analysis. All data are presented as mean ± standard error of the mean (mean ± SEM). Results with a normal distribution (p > .05 in Kolmogorov–Smirnov tests) were analyzed using Student two-tailed t-tests to compare two groups, or two-way ANOVA followed by a Tukey’s post-hoc analysis for multiple comparisons. Data that did not conform to a normal distribution were converted by LG arithmetic. For all comparisons, p < .05 was set as statistically significant.
3. Results
3.1. CYLD deficiency leads to an unexpected reaction to ARS in mice
Our previous studies have shown that Cyld−/−mice display anxiety-like behavior (Han et al., Citation2020). We hypothesized that CYLD plays a crucial role in anxiety induced by stress. To support this hypothesis, C57BL/6J mice were subjected to ARS (Figure S1(A)). In the EPM, ARS mice spent significantly less time in the open arms (Figure S1(C)) and made significantly less entries into the open arms (Figure S1(D)). A significantly lower level of Cyld mRNA expression was shown in mPFC and DS of ARS mice (Figure S1(E and F)). These data suggest that ARS is likely to lead anxious behavior and down-regulate Cyld mRNA expression. So, we initially speculated that ARS might aggravate anxiety-like behavior in Cyld−/− mice. As shown in , the EPM and LDT were used to assess anxious behavior in CYLD-knockout mice after ARS. For the EPM, the time spent in the open arms of Cyld−/− mice in response to ARS was significantly increased, and the time in Cyld−/− +ARS mice was significantly increased than Cyld+/+ +ARS mice (; two-way ANOVA: treatment effect: F (1, 36) =12.41, p < .01; genotype effect: F (1, 36) = 3.185, p > .05; interaction effect: F (1, 36) = 11.06, p < .01. Tukey’s post hoc test: Cyld−/− vs. Cyld−/− +ARS p ≤ .0001; Cyld−/− +ARS vs. Cyld+/+ +ARS p <.01). Similar results were found when we analyze the times to the open arms (; two-way ANOVA: treatment effect: F (1, 36) = 21.7, p < .0001; genotype effect: F (1, 36) = 0.2679, p > .05; interaction effect: F (1, 36) = 22.92, p < .0001. Tukey’s post hoc test: Cyld+/+ vs. Cyld−/− p < 0.01; Cyld−/− vs. Cyld−/− +ARS p < 0.0001; Cyld−/− +ARS vs. Cyld+/+ +ARS p < .01). For the LTD, the time in the light box of Cyld+/+ mice in response to ARS was significantly decreased, but the time in Cyld−/− +ARS mice and Cyld−/− mice were comparable; while the time in response to ARS was significantly increased in Cyld−/− mice than in Cyld+/+ mice (; two-way ANOVA: treatment effect: F (1, 36) =10.65, p < .01; genotype effect: F (1, 36) =0.7298, p > .05; interaction effect: F (1, 36) = 12.15, p < .01. Tukey’s post hoc test: Cyld+/+ vs. Cyld+/++ARS p < .0001; Cyld−/− vs. Cyld−/− +ARS p > .05; Cyld−/− +ARS vs. Cyld+/+ +ARS p < .05). Similar results were found when we analyze the transitions (; two-way ANOVA: treatment effect: F (1, 36) = 12.14, p < .01; genotype effect: F (1, 36) = 1.7, p > .05; interaction effect: F (1, 36) = 8.324, p < .01. Tukey’s post hoc test: Cyld+/+ vs. Cyld+/+ +ARS p < .001; Cyld−/− vs. Cyld−/− +ARS p > .05; Cyld−/− vs. Cyld+/+ p < .05). These data suggest that ARS is likely to lead anxious behavior in Cyld+/+ mice, not Cyld−/− mice. Therefore, CYLD deficiency leads to unexpected response to ARS in mice.
3.2. Effect of ARS on c-Fos expression in the brain of Cyld−/− mice
The expression of c-Fos can be used as a sign of neuronal activation by stimulation and therefore represents neuronal excitability (Bullitt, Citation1990). To elucidate which brain regions are involved in an unexpected reaction to ARS in CYLD-knockout mice, a detailed investigation of c-Fos immunoreactivity was carried out for each region of interest as illustrated in . Of the brain regions affected by stress, the mPFC, amygdala, and hippocampus are amongst the most well-studied for their roles in the control of the HPA axis response to stress (Kinlein et al., Citation2015; Kinlein et al., Citation2022). Besides, considering the high expression of CYLD in the DS and NAc, we also detected the expression of c-Fos in these two regions.
We firstly quantified the number of c-Fos+ cells in the mPFC. To minimize the effect of topographical variability on the quantification of c-Fos+ cells in the mPFC, selected sections were acquired at similar rostrocaudal levels and spaced the same distance (1.5–2.0 mm from the Bregma point, ). Representative 200× images of c-Fos immunoreactivity in the mPFC are presented in . The number of c-Fos+ cells in mPFC of Cyld+/+ and Cyld−/− mice were comparable in the absence of ARS; while the number of c-Fos+ cells in response to ARS in Cyld−/− mice was significantly increased than in Cyld+/+ mice (; two-way ANOVA: treatment effect: F (1, 29) = 85.14, p < .0001; genotype effect: F (1, 29) = 1.996, p > .05; interaction effect: F (1, 29) = 2.731, p > .05. Tukey’s post hoc test: Cyld+/+ vs. Cyld−/− p > .05; Cyld+/+ vs. Cyld+/++ARS p < .0001; Cyld−/− vs. Cyld−/− +ARS p < .0001; Cyld−/− +ARS vs. Cyld+/+ +ARS p < .05). Similar results were found when we analyze the number of c-Fos+ cells of Cyld−/− mice in response to ARS in the DS (; two-way ANOVA: treatment effect: F (1, 37) = 109.7, p < .0001; genotype effect: F (1, 37) = 7.14, p < .05; interaction effect: (F (1, 37) = 7.933, p < .01. Tukey’s post hoc test: Cyld+/+ vs. Cyld−/− p > .05; Cyld+/+ vs. Cyld+/++ARS p < .0001; Cyld−/− vs. Cyld−/− +ARS p < .0001; Cyld−/− +ARS vs. Cyld+/+ +ARS p < .0001), NAc (; two-way ANOVA: treatment effect: F (1, 36) = 88.22, p < .0001; genotype effect: F (1, 36) = 8.956, p < .01; interaction effect: F (1, 36) = 7.932, p < .01. Tukey’s post hoc test: Cyld+/+ vs. Cyld−/− p > .05; Cyld+/+ vs. Cyld+/+ +ARS p < .01; Cyld−/− vs. Cyld−/− +ARS p < .0001; Cyld−/− +ARS vs. Cyld+/+ +ARS p < .0001) and BLA (; two-way ANOVA: treatment effect: F (1, 27) = 219.9, p < .0001; genotype effect: F (1, 27) = 3.033, p > .05; interaction effect: F (1, 27) = 3.757, p > .05. Tukey’s post hoc test: Cyld+/+ vs. Cyld−/− p > .05; Cyld+/+ vs. Cyld+/++ARS p < .0001; Cyld−/− vs. Cyld−/− +ARS p < .0001; Cyld−/− +ARS vs. Cyld+/+ +ARS p < .05), but not vHPC (; two-way ANOVA: treatment effect: F (1, 26) = 255.4, p < .0001; genotype effect: F (1, 26) = 0.155, p > .05; interaction effect: F (1, 26) = 0.0003, p > .05. Tukey’s post hoc test: Cyld+/+ vs. Cyld−/− p > .05; Cyld+/+ vs. Cyld+/++ARS p < .0001; Cyld−/− vs. Cyld−/− +ARS p < .0001; Cyld−/− +ARS vs. Cyld+/+ +ARS p > .05). These results suggested that ARS induced significant activation of several brain regions including mPFC, DS, NAc, and BLA, not vHPC in Cyld-knockout mice.
4. Discussion
The study provides evidence that ARS induces anxious behavior in Cyld+/+ mice, but not in Cyld−/− counterparts. Indeed, we found that ARS induces significant activation of several brain regions including mPFC, DS, NAc, and BLA in Cyld-knockout mice. The study, therefore, highlights several areas of the brain that should be investigated to further understand the role of CYLD in the brain and the adaptive function of CYLD under stress.
ARS is an appropriate means of inducing stress in laboratory rodents, which result in emotional responses such as anxiety or depression (Liu et al., Citation2019; Zhou et al., Citation2019). Prior to exposure to ARS, Cyld−/− mice exhibited anxiety-like behavior in EPM and LDT compared with Cyld+/+ mice. However, although ARS induces anxious behavior in the LDT of Cyld+/+ mice, this did not occur in the EPM of Cyld+/+ mice. The results of EPM in the study are also not exactly consistent with LDT, and similar results are in our previous article (Han et al., Citation2020). Previous studies found EPM results were not stable or reliable when animals have had previous behavioral experience (Smith et al., Citation2016; Yaeger et al., Citation2020, Citation2022). Although it is also likely that exposing the EPM immediately prior to the LDT interfered with the expression of behavior in the LDT. Considering the reduction principle of animal ethics, the order of behavioral tests of the same mouse in the study was arranged such that the least stressful tests (EPM) with minimal carry-over effects were performed before the more stressful tests (LDT). Besides, the tests of OFT and EPM for anxiety and/or depression in rodents have failed to faithfully translate to successful clinical trials (Haller and Alicki, Citation2012; Haller et al., Citation2013). Therefore, more effective models should be carefully designed from an ecological and behavioral perspective.
Our previous studies have shown that Cyld−/− mice display anxiety-like behavior (Han et al., Citation2020) and fear-memory deficit (Li et al., Citation2021). Other studies of Cyld−/− mice point to their autistic symptoms, including abnormal sociability, depression, cognitive inflexibility, and repetitive behavior (Colombo et al., Citation2021; Zajicek et al., Citation2022). Accordingly, we initially speculated that ARS might aggravate anxious behavior in Cyld−/− mice. However, anxious behavior did not induce by ARS in EPM and LDT of Cyld−/− mice. In other words, the response of Cyld−/− mice to ARS was abnormal. In rats, the anxiety associated with ARS might be caused by increased dendritic-spine density in the BLA complex (Novaes et al., Citation2018) or hippocampus CA1 (Aguayo et al., Citation2018). However, in Cyld−/− mice, the increase in dendritic branches and spine density is thought to be due to abnormal dendritic pruning (Colombo et al., Citation2021; Li et al., Citation2019; Zajicek et al., Citation2022). Furthermore, although ARS suppresses Akt activity and GABAergic transmission (Assad et al., Citation2020; Jin et al., Citation2015), both Akt activity and GABA receptor levels are higher in Cyld−/− mice (Zajicek et al., Citation2022; Zhang et al., Citation2016).
Here, we confirm that exposure to ARS induced a distinct pattern of c-Fos protein expression activating most of the brain regions analyzed. These findings are in agreement with previous studies reporting increased neuronal activation in a large range of areas following ARS exposure especially in regions involved in the processing of anxiety and stressful stimuli (Azevedo et al., Citation2020; Kovács et al., Citation2018; Ma et al., Citation2014). Besides, significant activation of several brain regions including mPFC, DS, NAc, and BLA was in Cyld−/− mice than that in Cyld+/+ mice response to ARS. Previous studies discovered that Akt activity are higher in Cyld−/− mice (Zajicek et al., Citation2022; Zhang et al., Citation2016), and the activation of the PI3K-Akt pathway can induce the expression of c-Fos (Mutti et al., Citation2022; Wang, Citation2021). Consequently, we speculate that CYLD knockout may lead to significant activation of ARS-induced neurons by increasing Akt activity. Although recent studies have shown a decrease in both the action potential (AP) firing rate and excitatory postsynaptic currents (EPSCs) in principal neurons in the BLA, GABAergic-projection medium spiny neurons (MSNs) in the dorsal lateral striatum and hippocampal CA1 neuron of Cyld−/− mice (Chen et al., Citation2023; Li et al., Citation2021; Tan et al., Citation2023), these results were found on mice that did not receive stress.
Immunofluorescent c-Fos staining shows that a reduced c-Fos expression in mPFC of Cyld+/+mice induced by ARS in the study. The mPFC primarily receives inputs from the amygdala, hypothalamus, and periaqueductal gray matter, and regulates emotional processes, attention, and executive functions (Barbas, Citation2000). Recent studies demonstrated that increased c-Fos expression or excitatory neuronal activation in mPFC can decrease anxiety-like behavior. For example, acute chemicogenetic activation of Ca2+/calmodulin-dependent protein kinase α (CamKIIα)-positive excitatory neurons in mPFC evokes a significant decrease in anxious behavior in the EPM task, accompanied by enhanced c-Fos expression in the mPFC (Pati et al., Citation2018). On the other hand, earlier pharmacological studies found that a reduction in mPFC activity results in a decline or no change in anxious behavior in rodent models (Bi et al., Citation2013; Saitoh et al., Citation2014). These contradictory findings may result from differential effects on distinct neuron types and their downstream projections.
Our previous study demonstrated that microglial activation in the DS participates in anxious behavior in Cyld-knockout mice (Han et al., Citation2020). The DS is generally considered to be critical for goal-directed behavior and habitual behavior (Burton et al., Citation2015). However, a considerable amount of research suggests that DS may play a key role in anxiety disorders, which are the most prevalent psychiatric conditions (Craske & Stein Citation2016; Stein et al., Citation2017). In this study, we observed increased c-Fos expression in the DS of Cyld−/− mice after exposure to ARS. Consistent with this finding, chronic stress is known to provoke highly elevated firing rates in striosomal output neurons, which is associated with abnormal cost-benefit integration in decision-making (Friedman et al., Citation2017). Indirect-pathway medium spiny projection neurons in the dorsomedial striatum control approach-avoidance conflicts in exploratory tasks, which highlights this neural population as a potential target for reducing avoidance in anxiety disorders (LeBlanc et al., Citation2020). Mice with neuronal inactivation in the dorsomedial striatum spend more time in the open arms of the EPM (Qiao et al., Citation2017). This accumulated evidence collectively demonstrates that neural circuits in the DS are involved in the anxiety mechanism. Previous studies have identified CYLD is highly expressed in the DS, followed by NAc, amygdala, hypothalamus, PFC, and hippocampus (Jin et al., Citation2019; Mazarei et al., Citation2010). It is well known that NAc mainly regulates aversive behavior via indirect-pathway medium spiny projection neurons received from the mPFC and limbic regions (Hikida et al., Citation2010). As observed in DS, the number c-Fos+ cell was also increased in the NAc of Cyld−/− mice after ARS.
The BLA and the central amygdala (CeA) are primarily involved in anxiety and fear. Our laboratory has previously demonstrated that the number of c-Fos neurons responding to the tone-cued fear test is reduced in the BLA of Cyld−/− mice (Li et al., Citation2021). In the study, we observed an increase in c-Fos expression in the BLA of Cyld−/− mice after ARS. Paradoxically, optogenetically activated BLA glutamatergic neurons did not influence anxious behavior in stressed mice (Xiao et al., Citation2020). The BLA contains principal glutamatergic neurons, inhibitory interneurons, and distinct neuronal circuits (Krabbe et al., Citation2018), so it mediates both anxiogenic and anxiolytic behavioral effects. The BLA glutamatergic neurons project to many regions, such as the CeA, NAc, vHIP, mPFC, and the bed nucleus of the stria terminalis. The activation of BLA projection neurons leads to an increase in anxiety, while activation of BLA excitatory axonal projections is anxiolytic (Calhoon & Tye, Citation2015; Tovote et al., Citation2015).
In the study, c-Fos expression was not changed in the vHPC of Cyld−/− mice in response to ARS. The vHPC regulates emotional behavior by receiving projections from mPFC, amygdala, NAc, and hypothalamus (Jimenez et al., Citation2018; Zhang et al., Citation2019). The vHPC is particularly vulnerable to stress (Gomes et al., Citation2019), because most hippocampal neurons (about 90%) are excitatory pyramidal neurons; thus, the vulnerability of the hippocampus can be attributed to the relative ease with which its excitatory-inhibitory balance is perturbed. Colombo et al. demonstrated that hippocampus CA1 neurons exhibit disrupted network excitability, reduced long-term potentiation, and decreased total dendrite length and spine numbers in Cyld−/− mice (Colombo et al., Citation2021). In contrast, there were no differences in several functional and morphological parameters of striatal medium spiny projection neurons, although CYLD is highly expressed in this region. Therefore, these findings indicate that the function of CYLD is region-specific and affects particular neuron populations. In the study, activation of hippocampal neurons by stress may have counteracted the neuronal inactivation caused by CYLD knockout, resulting in no net change.
To summarize, CYLD deficiency leads to an unexpected reaction to ARS in mice, and it is accompanied by significant neuronal activation of specific brain regions including mPFC, DS, NAc, and BLA. The study provides insight into the role of CYLD in multiple areas of the brain and reminds us that future research should pay more attention to the regional and cellular heterogeneity of CYLD function.
Author contributions
Yuan-Yuan HAN designed and performed immunostaining and behavioral experiments. Jian-Wen ZHOU and Zhi-Wei GUO conducted elevated-plus maze test. Zhuo-Qing WU and Zai-yong Zhang conducted c-Fos photographing and calculation. Yuan-Yuan HAN analyzed the data and wrote the paper. De-Xiang provided with suggestions on manuscript. Cheng LONG supervised the study, designed experiments and critical revision of the manuscript.
Supplemental Material
Download MS Word (426.2 KB)Disclosure statement
No potential conflict of interest was reported by the author(s).
Additional information
Funding
Notes on contributors
Yuan-Yuan Han
Yuan-Yuan Han Ph.D graduated from South China Normal University, majoring in Neurobiology. She is currently doing postdoctoral research in Guangzhou Panyu District Hospital, who focuses on the immune and neural mechanisms of mental illness.
Jian-Wen Zhou
Jian-Wen Zhou is studying for a master’s degree at South China Normal University, who focus the regulation of striatum CYLD in anxiety.
Zhi-Wei Guo
Zhi-Wei Guo is a bachelor in South China Normal University, who studies anxiety and depression in mouse model of breast cancer.
Zhuo-Qing Wu
Zhuo-Qing Wu is studying for a master’s degree at South China Normal University, who mainly studies biology teaching.
Zai-Yong Zhang
Zai-Yong Zhang, Ph.D is the deputy chief physician of Guangzhou Panyu District Hospital, and he studies on coronary heart disease intervention and comprehensive management of heart patients.
De-xiang Liu
De-Xiang Liu is the chief physician of Guangzhou Panyu District Hospital, who specializes in magnetic resonance techniques and diagnostics.
Cheng Long
Cheng Long, Ph.D is a professor at South China Normal University, who focuses on neurodegenerative diseases and neural signal transduction. He presided over the National Natural Science Foundation of China, Guangdong Provincial Natural Science Foundation, Guangdong Provincial Department of Education Intelligence Introduction Special Project and Guangdong Provincial Department of Education Graduate Demonstration Course and other projects. He has published more than 80 research papers in domestic and foreign academic journals.
References
- Aguayo, F. I., Tejos-Bravo, M., Díaz-Véliz, G., Pacheco, A., García-Rojo, G., Corrales, W., Olave, F. A., Aliaga, E., Ulloa, J. L., Avalos, A. M., Román-Albasini, L., Rojas, P. S., & Fiedler, J. L. (2018). Hippocampal memory recovery after acute stress: A behavioral, morphological and molecular study. Frontiers in Molecular Neuroscience, 11, 1. https://doi.org/10.3389/fnmol.2018.00283
- Assad, N., Luz, W. L., Santos-Silva, M., Carvalho, T., Moraes, S., Picanço-Diniz, D. L. W., Bahia, C. P., Oliveira Batista, E. d J., da Conceição Passos, A., Oliveira, K. R. H. M., & Herculano, A. M. (2020). Acute restraint stress evokes anxiety-like behavior mediated by telencephalic inactivation and GabAergic dysfunction in zebrafish brains. Scientific Reports, 10(1), 5551. https://doi.org/10.1038/s41598-020-62077-w
- Azevedo, E. P., Tan, B., Pomeranz, L. E., Ivan, V., Fetcho, R., Schneeberger, M., Doerig, K. R., Liston, C., Friedman, J. M., & Stern, S. A. (2020). A limbic circuit selectively links active escape to food suppression. ELife, 9, e58894. https://doi.org/10.7554/eLife.58894
- Barbas, H. (2000). Connections underlying the synthesis of cognition, memory, and emotion in primate prefrontal cortices. Brain Research Bulletin, 52(5), 319–12. https://doi.org/10.1016/s0361-9230(99)00245-2
- Bi, L.-L., Wang, J., Luo, Z.-Y., Chen, S.-P., Geng, F., Chen, Y-h., Li, S.-J., Yuan, C-h., Lin, S., & Gao, T.-M. (2013). Enhanced excitability in the infralimbic cortex produces anxiety-like behaviors. Neuropharmacology, 72, 148–156. https://doi.org/10.1016/j.neuropharm.2013.04.048
- Bignell, G. R., Warren, W., Seal, S., Takahashi, M., Rapley, E., Barfoot, R., Green, H., Brown, C., Biggs, P. J., Lakhani, S. R., Jones, C., Hansen, J., Blair, E., Hofmann, B., Siebert, R., Turner, G., Evans, D. G., Schrander-Stumpel, C., Beemer, F. A., … Rasmussen, S. (2000). Identification of the familial cylindromatosis tumour-suppressor gene. Nature Genetics, 25(2), 160–165. https://doi.org/10.1038/76006
- Bullitt, E. (1990). Expression of c-fos-like protein as a marker for neuronal activity following noxious stimulation in the rat. The Journal of Comparative Neurology, 296(4), 517–530. https://doi.org/10.1002/cne.902960402
- Burton, A. C., Nakamura, K., & Roesch, M. R. (2015). From ventral-medial to dorsal-lateral striatum: neural correlates of reward-guided decision-making. Neurobiology of Learning and Memory, 117, 51–59. https://doi.org/10.1016/j.nlm.2014.05.003
- Calhoon, G. G., & Tye, K. M. (2015). Resolving the neural circuits of anxiety. Nature Neuroscience, 18(10), 1394–1404. https://doi.org/10.1038/nn.4101
- Chen, S.-Y., Liu, K.-F., Tan, S.-Y., Chen, X.-S., Li, H.-D., Li, J.-J., Zhou, J.-W., Yang, L., & Long, C. (2023). Deubiquitinase CYLD regulates excitatory synaptic transmission and short-term plasticity in the hippocampus. Brain Research, 1806, 148313. https://doi.org/10.1016/j.brainres.2023.148313
- Colombo, E., Horta, G., Roesler, M. K., et al. (2021). The K63 deubiquitinase CYLD modulates autism-like behaviors and hippocampal plasticity by regulating autophagy and mTOR signaling. Proceedings of the National Academy of Sciences of the United States of America, 118(47), e2110755118. https://doi.org/10.1073/pnas.2110755118
- Craske, M. G., & Stein, M. B. (2016). Anxiety. Lancet, 388(10063), 3048–3059. https://doi.org/10.1016/S0140-6736(16)30381-6
- Dobson-Stone, C., Hallupp, M., Shahheydari, H., Ragagnin, A. M. G., Chatterton, Z., Carew-Jones, F., Shepherd, C. E., Stefen, H., Paric, E., Fath, T., Thompson, E. M., Blumbergs, P., Short, C. L., Field, C. D., Panegyres, P. K., Hecker, J., Nicholson, G., Shaw, A. D., Fullerton, J. M., … Kwok, J. B. (2020). CYLD is a causative gene for frontotemporal dementia - amyotrophic lateral sclerosis. Brain, 143(3), 783–799. https://doi.org/10.1093/brain/awaa039
- Dosemeci, A., Thein, S., Yang, Y., Reese, T. S., & Tao-Cheng, J.-H. (2013). CYLD, a deubiquitinase specific for lysine63-linked polyubiquitins, accumulates at the postsynaptic density in an activity-dependent manner. Biochemical and Biophysical Research Communications, 430(1), 245–249. https://doi.org/10.1016/j.bbrc.2012.10.131
- Duncan, L. E., Pollastri, A. R., & Smoller, J. W. (2014). Mind the gap: why many geneticists and psychological scientists have discrepant views about gene-environment interaction (G × E) research. The American Psychologist, 69(3), 249–268. https://doi.org/10.1037/a0036320
- Friedman, A., Homma, D., Bloem, B., Gibb, L. G., Amemori, K.-I., Hu, D., Delcasso, S., Truong, T. F., Yang, J., Hood, A. S., Mikofalvy, K. A., Beck, D. W., Nguyen, N., Nelson, E. D., Toro Arana, S. E., Vorder Bruegge, R. H., Goosens, K. A., & Graybiel, A. M. (2017). Chronic stress alters striosome-circuit dynamics, leading to aberrant decision-making. Cell, 171(5), 1191–1205 e1128. https://doi.org/10.1016/j.cell.2017.10.017
- Godar, S. C., Bortolato, M., Richards, S. E., et al. (2015). Monoamine oxidase A is required for rapid dendritic remodeling in response to stress. The International Journal of Neuropsychopharmacology, 18(9), pyv035. https://doi.org/10.1093/ijnp/pyv035
- Gomes, F. V., Zhu, X., & Grace, A. A. (2019). Stress during critical periods of development and risk for schizophrenia. Schizophrenia Research, 213, 107–113. https://doi.org/10.1016/j.schres.2019.01.030
- Haller, J., & Alicki, M. (2012). Current animal models of anxiety, anxiety disorders, and anxiolytic drugs. Current Opinion in Psychiatry, 25(1), 59–64. https://doi.org/10.1097/YCO.0b013e32834de34f
- Haller, J., Aliczki, M., & Gyimesine Pelczer, K. (2013). Classical and novel approaches to the preclinical testing of anxiolytics: A critical evaluation. Neuroscience and Biobehavioral Reviews, 37(10 Pt 1), 2318–2330. https://doi.org/10.1016/j.neubiorev.2012.09.001
- Han, Y.-Y., Jin, K., Pan, Q.-S., Li, B., Wu, Z.-Q., Gan, L., Yang, L., & Long, C. (2020). Microglial activation in the dorsal striatum participates in anxiety-like behavior in Cyld knockout mice. Brain, Behavior, and Immunity, 89, 326–338. https://doi.org/10.1016/j.bbi.2020.07.011
- Han, A., Yeo, H., Park, M.-J., Kim, S. H., Choi, H. J., Hong, C.-W., & Kwon, M.-S. (2015). IL-4/10 prevents stress vulnerability following imipramine discontinuation. Journal of Neuroinflammation, 12, 197. https://doi.org/10.1186/s12974-015-0416-3
- Hikida, T., Kimura, K., Wada, N., Funabiki, K., & Nakanishi, S. (2010). Distinct roles of synaptic transmission in direct and indirect striatal pathways to reward and aversive behavior. Neuron, 66(6), 896–907. https://doi.org/10.1016/j.neuron.2010.05.011
- Jimenez, J. C., Su, K., Goldberg, A. R., Luna, V. M., Biane, J. S., Ordek, G., Zhou, P., Ong, S. K., Wright, M. A., Zweifel, L., Paninski, L., Hen, R., & Kheirbek, M. A. (2018). Anxiety cells in a hippocampal-hypothalamic circuit. Neuron, 97(3), 670–683 e676. https://doi.org/10.1016/j.neuron.2018.01.016
- Jin, Y., Kanno, T., & Nishizaki, T. (2015). Acute restraint stress impairs induction of long-term potentiation by activating GSK-3beta. Neurochemical Research, 40(1), 36–40. https://doi.org/10.1007/s11064-014-1462-4
- Jin, C., Kim, S., Kang, H., Yun, K. N., Lee, Y., Zhang, Y., Kim, Y., Kim, J. Y., & Han, K. (2019). Shank3 regulates striatal synaptic abundance of Cyld, a deubiquitinase specific for Lys63-linked polyubiquitin chains. Journal of Neurochemistry, 150(6), 776–786. https://doi.org/10.1111/jnc.14796
- Joffe, M. E., Maksymetz, J., Luschinger, J. R., Dogra, S., Ferranti, A. S., Luessen, D. J., Gallinger, I. M., Xiang, Z., Branthwaite, H., Melugin, P. R., Williford, K. M., Centanni, S. W., Shields, B. C., Lindsley, C. W., Calipari, E. S., Siciliano, C. A., Niswender, C. M., Tadross, M. R., Winder, D. G., & Conn, P. J. (2022). Acute restraint stress redirects prefrontal cortex circuit function through mGlu5 receptor plasticity on somatostatin-expressing interneurons. Neuron, 110(6), 1068–1083 e1065. https://doi.org/10.1016/j.neuron.2021.12.027
- Kinlein, S. A., Wilson, C. D., & Karatsoreos, I. N. (2015). Dysregulated hypothalamic–pituitary–adrenal axis function contributes to altered endocrine and neurobehavioral responses to acute stress. Frontiers in Psychiatry, 6, 31. https://doi.org/10.3389/fpsyt.2015.00031
- Kinlein, S. A., Wallace, N. K., Savenkova, M. I., & Karatsoreos, I. N. (2022). Chronic hypothalamic-pituitary-adrenal axis disruption alters glutamate homeostasis and neural responses to stress in male C57Bl6/N mice. Neurobiology of Stress, 19, 100466.https://doi.org/10.1016/j.ynstr.2022.100466
- Kovács, L. Á., Schiessl, J. A., Nafz, A. E., Csernus, V., & Gaszner, B. (2018). Both basal and acute restraint stress-induced c-Fos expression is influenced by age in the extended amygdala and brainstem stress centers in male rats. Frontiers in Aging Neuroscience, 10, 248. https://doi.org/10.3389/fnagi.2018.00248
- Kovalenko, A., Chable-Bessia, C., Cantarella, G., Israël, A., Wallach, D., & Courtois, G. (2003). The tumour suppressor CYLD negatively regulates NF-kappaB signalling by deubiquitination. Nature, 424(6950), 801–805. https://doi.org/10.1038/nature01802
- Krabbe, S., Gründemann, J., & Lüthi, A. (2018). Amygdala inhibitory circuits regulate associative fear conditioning. Biological Psychiatry, 83(10), 800–809. https://doi.org/10.1016/j.biopsych.2017.10.006
- LeBlanc, K. H., London, T. D., Szczot, I., Bocarsly, M. E., Friend, D. M., Nguyen, K. P., Mengesha, M. M., Rubinstein, M., Alvarez, V. A., & Kravitz, A. V. (2020). Striatopallidal neurons control avoidance behavior in exploratory tasks. Molecular Psychiatry, 25(2), 491–505. https://doi.org/10.1038/s41380-018-0051-3
- Li, H.-D., Li, D.-N., Yang, L., & Long, C. (2021). Deficiency of the CYLD impairs fear memory of mice and disrupts neuronal activity and synaptic transmission in the basolateral amygdala. Frontiers in Cellular Neuroscience, 15, 740165. https://doi.org/10.3389/fncel.2021.740165
- Li, J., Sekine-Aizawa, Y., Ebrahimi, S., Tanaka, S., & Okabe, S. (2019). Tumor suppressor protein CYLD regulates morphogenesis of dendrites and spines. The European Journal of Neuroscience, 50(4), 2722–2739. https://doi.org/10.1111/ejn.14421
- Liu, L., Liu, H., Hou, Y., Shen, J., Qu, X., & Liu, S. (2019). Temporal effect of electroacupuncture on anxiety-like behaviors and c-Fos expression in the anterior cingulate cortex in a rat model of post-traumatic stress disorder. Neuroscience Letters, 711, 134432. https://doi.org/10.1016/j.neulet.2019.134432
- MacDowell, K. S., Caso, J. R., Martin-Hernandez, D., et al. (2014). Paliperidone prevents brain toll-like receptor 4 pathway activation and neuroinflammation in rat models of acute and chronic restraint stress. The International Journal of Neuropsychopharmacology. 18(3), pyu070.
- Ma, Y., Matsuwaki, T., Yamanouchi, K., & Nishihara, M. (2014). Differential roles of cyclooxygenase-2-related signaling in regulating hypothalamic neuronal activity under various acute stresses. The Journal of Veterinary Medical Science, 76(2), 219–227. https://doi.org/10.1292/jvms.13-0234
- Ma, Q., Ruan, H., Peng, L., et al. (2017). Proteasome-independent polyubiquitin linkage regulates synapse scaffolding, efficacy, and plasticity. Proceedings of the National Academy of Sciences of the United States of America, 114, E8760–E8769.
- Mazarei, G., Neal, S. J., Becanovic, K., Luthi-Carter, R., Simpson, E. M., & Leavitt, B. R. (2010). Expression analysis of novel striatal-enriched genes in Huntington disease. Human Molecular Genetics, 19(4), 609–622. https://doi.org/10.1093/hmg/ddp527
- Mutti, V., Bono, F., Tomasoni, Z., Bontempi, L., Guglielmi, A., Bolognin, S., Schwamborn, J. C., Missale, C., & Fiorentini, C. (2022). Structural plasticity of dopaminergic neurons requires the activation of the D3R-nAChR heteromer and the PI3K-ERK1/2/Akt-induced expression of c-Fos and p70S6K signaling pathway. Molecular Neurobiology, 59(4), 2129–2149. https://doi.org/10.1007/s12035-022-02748-z
- Novaes, L. S., Dos Santos, N. B., Perfetto, J. G., Goosens, K. A., & Munhoz, C. D. (2018). Environmental enrichment prevents acute restraint stress-induced anxiety-related behavior but not changes in basolateral amygdala spine density. Psychoneuroendocrinology, 98, 6–10. https://doi.org/10.1016/j.psyneuen.2018.07.031
- Pati, S., Sood, A., Mukhopadhyay, S., & Vaidya, V. A. (2018). Acute pharmacogenetic activation of medial prefrontal cortex excitatory neurons regulates anxiety-like behaviour. Journal of Biosciences, 43(1), 85–95. https://doi.org/10.1007/s12038-018-9732-y
- Paxinos, G., Franklin, K. (2001). The mouse brain in stereotoxic coordinates. Academic Press.
- Qiao, Y., Wang, X., Ma, L., Li, S., & Liang, J. (2017). Functional inactivation of dorsal medial striatum alters behavioral flexibility and recognition process in mice. Physiology & Behavior, 179, 467–477. https://doi.org/10.1016/j.physbeh.2017.07.026
- Reiley, W. W., Jin, W., Lee, A. J., Wright, A., Wu, X., Tewalt, E. F., Leonard, T. O., Norbury, C. C., Fitzpatrick, L., Zhang, M., & Sun, S.-C. (2007). Deubiquitinating enzyme CYLD negatively regulates the ubiquitin-dependent kinase Tak1 and prevents abnormal T cell responses. The Journal of Experimental Medicine, 204(6), 1475–1485. https://doi.org/10.1084/jem.20062694
- Saitoh, A., Ohashi, M., Suzuki, S., Tsukagoshi, M., Sugiyama, A., Yamada, M., Oka, J.-I., Inagaki, M., & Yamada, M. (2014). Activation of the prelimbic medial prefrontal cortex induces anxiety-like behaviors via N-Methyl-D-aspartate receptor-mediated glutamatergic neurotransmission in mice. Journal of Neuroscience Research, 92(8), 1044–1053. https://doi.org/10.1002/jnr.23391
- Smith, J. P., Prince, M. A., Achua, J. K., Robertson, J. M., Anderson, R. T., Ronan, P. J., & Summers, C. H. (2016). Intensity of anxiety is modified via complex integrative stress circuitries. Psychoneuroendocrinology, 63, 351–361. https://doi.org/10.1016/j.psyneuen.2015.10.016
- Stein, D. J., Scott, K. M., de Jonge, P., & Kessler, R. C. (2017). Epidemiology of anxiety disorders: from surveys to nosology and back. Dialogues in Clinical Neuroscience, 19(2), 127–136. https://doi.org/10.31887/DCNS.2017.19.2/dstein
- Sun, S. C. (2010). CYLD: a tumor suppressor deubiquitinase regulating NF-kappaB activation and diverse biological processes. Cell Death and Differentiation, 17(1), 25–34. https://doi.org/10.1038/cdd.2009.43
- Tábuas-Pereira, M., Santana, I., Kun-Rodrigues, C., Bras, J., & Guerreiro, R. (2020). CYLD variants in frontotemporal dementia associated with severe memory impairment in a Portuguese cohort. Brain, 143(8), e67. https://doi.org/10.1093/brain/awaa183
- Tan, S.-Y., Jiang, J.-X., Huang, H.-X., Mo, X.-P., Feng, J.-R., Chen, Y., Yang, L., & Long, C. (2023). Neural mechanism underlies CYLD modulation of morphology and synaptic function of medium spiny neurons in dorsolateral striatum. Frontiers in Molecular Neuroscience, 16, 1107355. https://doi.org/10.3389/fnmol.2023.1107355
- Thein, S., Tao-Cheng, J.-H., Li, Y., Bayer, K. U., Reese, T. S., & Dosemeci, A. (2014). CaMKII mediates recruitment and activation of the deubiquitinase CYLD at the postsynaptic density. PLOS One, 9(3), e91312. https://doi.org/10.1371/journal.pone.0091312
- Tovote, P., Fadok, J. P., & Luthi, A. (2015). Neuronal circuits for fear and anxiety. Nature Reviews Neuroscience, 16(6), 317–331. https://doi.org/10.1038/nrn3945
- Trompouki, E., Hatzivassiliou, E., Tsichritzis, T., Farmer, H., Ashworth, A., & Mosialos, G. (2003). CYLD is a deubiquitinating enzyme that negatively regulates NF-kappaB activation by TNFR family members. Nature, 424(6950), 793–796. https://doi.org/10.1038/nature01803
- Tu, B.-X., Wang, L.-F., Zhong, X.-L., Hu, Z.-L., Cao, W.-Y., Cui, Y.-H., Li, S.-J., Zou, G.-J., Liu, Y., Zhou, S.-F., Zhang, W.-J., Su, J.-Z., Yan, X.-X., Li, F., & Li, C.-Q. (2019). Acute restraint stress alters food-foraging behavior in rats: Taking the easier Way while suffered. Brain Research Bulletin, 149, 184–193. https://doi.org/10.1016/j.brainresbull.2019.04.021
- Walf, A. A., & Frye, C. A. (2007). The use of the elevated plus maze as an assay of anxiety-related behavior in rodents. Nature Protocols, 2(2), 322–328. https://doi.org/10.1038/nprot.2007.44
- Wang, Z. (2021). Regulation of cell cycle progression by growth factor-induced cell signaling. Cells, 10(12), 3327. https://doi.org/10.3390/cells10123327
- Xiao, Q., Xu, X., & Tu, J. (2020). Chronic optogenetic manipulation of basolateral amygdala astrocytes rescues stress-induced anxiety. Biochemical and Biophysical Research Communications, 533(4), 657–664. https://doi.org/10.1016/j.bbrc.2020.09.106
- Xu, C., Yang, L., Yuan, Y., Du, F., Wang, S., Wang, X., Zhu, L., Zhang, B., & Weaver, D. (2016). Up-regulation of CYLD enhances Listeria monocytogenes induced apoptosis in THP-1 cells. Microbial Pathogenesis, 90, 50–54. https://doi.org/10.1016/j.micpath.2015.10.007
- Yaeger, J. D., Krupp, K. T., Gale, J. J., & Summers, C. H. (2020). Counterbalanced microcircuits for Orx1 and Orx2 regulation of stress reactivity. Medicine in Drug Discovery, 8, 100059. https://doi.org/10.1016/j.medidd.2020.100059
- Yaeger, J. D. W., Krupp, K. T., Summers, T. R., & Summers, C. H. (2022). Contextual generalization of social stress learning is modulated by orexin receptors in basolateral amygdala. Neuropharmacology, 215, 109168. https://doi.org/10.1016/j.neuropharm.2022.109168
- Zajicek, A. S., Ruan, H., Dai, H., Skolfield, M. C., Phillips, H. L., Burnette, W. J., Javidfar, B., Sun, S.-C., Akbarian, S., & Yao, W.-D. (2022). Cylindromatosis drives synapse pruning and weakening by promoting macroautophagy through Akt-mTOR signaling. Molecular Psychiatry, 27(5), 2414–2424. https://doi.org/10.1038/s41380-022-01571-1
- Zajicek, A., & Yao, W. D. (2021). Remodeling without destruction: non-proteolytic ubiquitin chains in neural function and brain disorders. Molecular Psychiatry, 26(1), 247–264. https://doi.org/10.1038/s41380-020-0849-7
- Zhang, J., Chen, M., Li, B., Lv, B., Jin, K., Zheng, S., Yang, L., & Long, C. (2016). Altered striatal rhythmic activity in cylindromatosis knock-out mice due to enhanced GABAergic inhibition. Neuropharmacology, 110(Pt A), 260–267. https://doi.org/10.1016/j.neuropharm.2016.06.021
- Zhang, J.-Y., Liu, T.-H., He, Y., Pan, H.-Q., Zhang, W.-H., Yin, X.-P., Tian, X.-L., Li, B.-M., Wang, X.-D., Holmes, A., Yuan, T.-F., & Pan, B.-X. (2019). Chronic stress remodels synapses in an amygdala circuit-specific manner. Biological Psychiatry, 85(3), 189–201. https://doi.org/10.1016/j.biopsych.2018.06.019
- Zhou, H.-Y., He, J.-G., Hu, Z.-L., Xue, S.-G., Xu, J.-F., Cui, Q.-Q., Gao, S.-Q., Zhou, B., Wu, P.-F., Long, L.-H., Wang, F., & Chen, J.-G. (2019). A-kinase anchoring protein 150 and protein kinase A complex in the basolateral amygdala contributes to depressive-like behaviors induced by chronic restraint stress. Biological Psychiatry, 86(2), 131–142. https://doi.org/10.1016/j.biopsych.2019.03.967