Abstract
Stress is ever present in our modern, performance-oriented and demanding society, which causes adverse stress reactions of the body and affects health seriously. Chronic stress has been recognized as a significant risk factor leading to cognitive impairment, but the underlying mechanism is far from fully understood. Norepinephrine (NE), a pivotal stress-induced hormone, has been found to induce cell apoptosis. However, the function and the key downstream mediator of NE on the regulation of hippocampal neurons still need further exploration. In this study, we explored the role of NE in neuronal apoptosis and its association with MALAT1. Flow cytometry assay and automated western bot assay were carried out to evaluate the cell apoptosis. The data showed that the rate of apoptosis rate and the levels of apoptotic proteins (cleaved-Caspase3 and cleaved-PARP) were significantly increased in HT22 cells after a high dose of NE treatment, suggesting a facilitative role of NE on hippocampal neuronal apoptosis. Besides, a high level of NE up-regulated the expression of MALAT1 in HT22 cells. Then, a lentivirus expressing MALAT1 shRNA was constructed to investigate the role of MALAT1 in cell apoptosis and the results revealed that MALAT1 depletion decreased the cell apoptosis. Moreover, the knockdown of MALAT1 abolished the discrepancy in apoptosis between NE-treated cells and control cells. In conclusion, a high level of the stress-induced hormone NE promoted apoptosis of hippocampal neurons by elevating the expression of MALAT1. Our findings provide new experimental data supporting the epigenetic mechanisms in the regulation of stress response and may provide a potential therapeutic target for stress-related cognition dysfunction.
1. Introduction
With the increasing pressure of life and work in modern society, many people are suffering from persistent strong mental and physical stress, which causes adverse stress reactions in the body and negatively affects health. Epidemiological investigations and experimental studies have suggested that chronic stress is a common risk factor for 75–90% of chronic noncommunicable diseases (Liu et al., Citation2017). Chronic stress can induce aberrantly persistent activation of the sympathetic nervous system (SNS), leading to the simultaneous elevation of catecholamines (Krizanova et al., Citation2016). It has been reported that norepinephrine (NE) can induce the apoptosis of endothelial cells (Fu et al., Citation2004), cardiac myocytes (Jindal & Goswami, Citation2011) and alveolar epithelial cells (Dincer et al., Citation2001). The brain, as the control center of cognitive function, is one of the most vulnerable organs under stress conditions. Studies have shown that chronic stress induces learning and memory dysfunction (Ma et al., Citation2023; Xie et al., Citation2016), but the underlying mechanism is still unclear. Hippocampal neuronal damage has been observed in the brains of mice under chronic stress (Wang et al., Citation2022b). However, the function and the key downstream mediator of NE, as a pivotal stress-induced hormone, on the regulation of hippocampal neurons need to be further explored. Further studies might provide new therapeutic targets for stress-related cognitive impairment.
Recently, epigenetic mechanisms have been found to play a vital role in stress-regulated gene expression. Stress has a clear impact on many types of molecular epigenetic mechanisms, from histone modifications to DNA methylation and hydroxymethylation and expression of noncoding RNA (ncRNA) (Griffiths & Hunter, Citation2014; Wang et al., Citation2022a). Long non-coding RNAs (lncRNAs) have been recognized as a class of epigenetic regulators with a potential wealth of biological functions, but findings associating them with stress-related cognitive dysfunction are still rare. Metastasis-associated lung adenocarcinoma transcript 1 (MALAT1) is characterized as a long polyadenylated ncRNA that is highly conserved in mammals and widely expressed in human tissues (Arun et al., Citation2020). MALAT1 was initially found to be overexpressed in various cancers and contribute to the regulation of tumor cell proliferation, apoptosis, metastasis, and other malignant behaviors (Goyal et al., Citation2021). Moreover, recent studies demonstrated that MALAT1 also plays a key role in neurodegenerative disease (Cai et al., Citation2020; Li et al., Citation2020). However, the function of MALAT1 in stress-induced neuronal damage has not been established. In the present study, we explored the role of stress-associated hormone NE and lncRNA MALAT1 in the regulation of neuronal apoptosis and elucidated that a high level of NE promoted neuronal apoptosis by raising the expression of MALAT1.
2. Materials and methods
2.1. Cell culture and intervention
The mouse hippocampal neuronal cell line, HT22, was purchased from BeNa Culture Collection (Beijing, China) and cultured in DMEM (Sigma, St. Louis MO, USA) containing 10% FBS (TIANHANG, Hangzhou, China) and 100 U/mL penicillin and streptomycin (Solarbio, Beijing, China). The cells were grown in a humidified incubator with 5% CO2 at 37 °C.
The short hairpin RNA (shRNA) oligonucleotides (oligos) targeting MALAT1 were synthesized by Genechem Co., Ltd. (Shanghai, China). The sequences of the synthesized oligos were as follows: 5′ – CCGGGGAAGTGAAAGACGAAGAAGACTCGAGTCTTCTTCGTCTTTCACTTCCTTTTTG − 3′ (forward) and 5′-AATTCAAAAAGGAAGTGAAAG
ACGAAGAAGACTCGAGTCTTCTTCGTCTTTCACTTCC−3′ (reverse). After annealing, the double-strand oligos were inserted into lentiviral GV493 vector (Genechem, Shanghai, China). To produce lentivirus, HEK-293T cells were co-transfected with the lentiviral vector described above and packaging vectors psPAX2 and VSVG using lipo2000 (Invitrogen, California, USA). The shRNA control virus was purchased from Genechem Co., Ltd. (Shanghai, China). Further, stable transfectants ofHT22 cells were established as previously described (Wang et al., Citation2022c).
NE (Sigma, St. Louis MO, USA) was dissolved in dimethyl sulfoxide (DMSO) (Sangon Biotech, Shanghai, China) at the concentration of 10, 50 and 100 mmol/L, respectively. Then, 1 μL/mL of NE was added to the culture medium for intervention. An equal volume of DMSO was used as control. After incubation for 48 h, the cells were collected for flow cytometry and Western blot analysis.
2.2. Flow cytometry analysis
Flow cytometry analysis was performed to measure cell apoptosis using the Annexin-V APC Apoptosis Detection Kit (Pepro Tech, New Jersey, USA). After incubation with NE or DMSO for 48 h, HT22 cells were washed with PBS, digested with 0.25% EDTA-free trypsin (Solarbio, Beijing, China) and collected by centrifuging at 800 g for 5 min. According to the manufacturer’s instructions, 100 μL of Annexin-V Binding Buffer, 5 μL of 7-AAD solution and 5 μL of Annexin-V APC were added to each sample, followed by incubation for 15 min at room temperature without light. Cell populations were detected by using NovoCyte Flow Cytometer (Agilent Technologies, California, USA) and data was analyzed by using NovoExpress software.
2.3. Protein quantification by automated Western blot (Wes)
Western blot was conducted using a fully automated protein expression analysis system Wes (ProteinSimple, Bio-Techne, Minnesota, USA) as previously reported (Liu et al., Citation2023; Wang et al., Citation2022b). The cells were lysed with Radio Immunoprecipitation Assay (RIPA) cell lysate (Solarbio, Beijing, China) containing protease inhibitors, and centrifuged at 12,000 g for 15 min at 4 °C to extract the supernatant. Then, the samples were quantified with a BCA protein quantification kit (Tiangen, Beijing, China) and adjusted to the concentration of 2 μg/μL by adding the Wes kit 0.1 × sample buffer. The cell samples, Mastermix, and antibodies were added respectively onto a WES plate (12–230 kDa, 25 capillaries) to conduct protein detection by WES Automatic Protein Expression Quantitative Analysis System according to the manufacturer’s instructions. Data were analysis using Compass for Wes software (version 4.10), and the grayscale values of the bands given by the software were used to calculate the relative protein expression with β-actin as an internal reference. The antibodies used in the study were Rabbit anti-Caspase3 (1:100, ABclonal, Wuhan, China), PARP (1:100, Cell Signaling Technology, Massachusetts, USA) and rabbit anti-β-actin (1:100, bimake, Texas, USA).
2.4. Quantitative real-time PCR (qRT-PCR)
Total RNA was extracted from cells using TRIzol reagent (Invitrogen, California, USA). Synthesis of cDNA was done using the M-MLV Reverse Transcriptase (Promega, Wisconsin, USA) according to the manufacturer’s instructions. Then qRT-PCR was performed using a LightCycler 96 Realtime PCR System (Roche, Basel, Switzerland) with TB Green Premix Ex Taq kit (TaKaRa, Kyoto, Japan). The β-actin was selected as the endogenous control in the assay. Relative levels of the indicated genes were calculated by 2−ΔΔCt method as reported previously (Zhang et al., Citation2021). The primers were designed using Primer Premier 5 software, and the sequences are provided as follows: MALAT1 forward primer: 5’-GAGCTCGCCAGGTTTACAGT-3’ and reverse primer: 5’-AACTACCAGCAATTCCGCCA-3’; β-actin forward primer: 5’-GAGCTCGCCAGGTTTACAGT-3’ and reverse primer: 5’-AACTACCAGCAATTCCGCCA-3’.
2.5. Data analysis
Statistical analysis was processed with GraphPad Prism software (version 8.0). The data were expressed as mean ± SD. The continuous variables were evaluated for normality before comparison for statistical differences. Student’s t-test was used for comparison between the two groups. One-way ANOVA analysis was performed to compare the values among multiple groups. Two-way ANOVA was performed to compare the values categorized by two variables. Significant results of ANOVA were subjected to Tukey’s multiple comparisons test. All differences were two-sided. A p-value less than 0.05 was considered statistically significant.
3. Results
3.1. A High dose of NE-induced HT22 cells apoptosis
The effect of NE on apoptosis of HT22 cells was examined by flow cytometry and Wes assay after intervening with different concentrations of NE (10, 50 and 100 μmol/L) for 48 h. The results of flow cytometry showed that compared with the DMSO group, the rate of apoptotic cells was not obviously changed upon 10 μmol/L of NE treatment, while it was significantly increased upon 50 and 100 μmol/L of NE treatment (, One-way ANOVA, F3,8 = 154.1, p < 0.0001). Wes assay was used to examine the protein levels of key apoptosis mediators. As shown in , the level of cleaved-Caspase3 was upregulated above 50 μmol/L of NE stimulation. Furthermore, the expression of cleaved-Caspase3 (One-way ANOVA, F3,8 = 686.4, p < 0.0001) and cleaved-PARP (One-way ANOVA, F3,8 = 39.05, p < 0.0001) were both observably induced upon 100 μmol/L NE treatment; this suggested that the high level of NE induced apoptosis of HT22 cells.
Figure 1. High dose of NE induced HT22 cells apoptosis. Flow cytometry analysis and Wes assay were used to evaluate the effect of NE on apoptosis of HT22 cells. (A) Representative images of HT22 cells treated with different concentrations of NE were examined by flow cytometry analysis. (B) The apoptosis rate of HT22 cells upon indicated concentration of NE administration (n = 3, One-way ANOVA, F3,8 = 154.1, p < 0.0001). (C) Expressions of cleaved-Caspase3 and cleaved-PARP in HT22 cells treated with DMSO or NE were detected by Wes assay. (D-F) The relative expressions of cleaved-Caspase3 (D, F3,8 = 686.4, p < 0.0001), cleaved-PARP (E, F3,8 = 39.05, p < 0.0001), and PARP (F, F3,8 = 0.2934, p = 0.8292) proteins were normalized to β-actin (n = 3, One-way ANOVA). ** p < 0.01 vs DMSO.
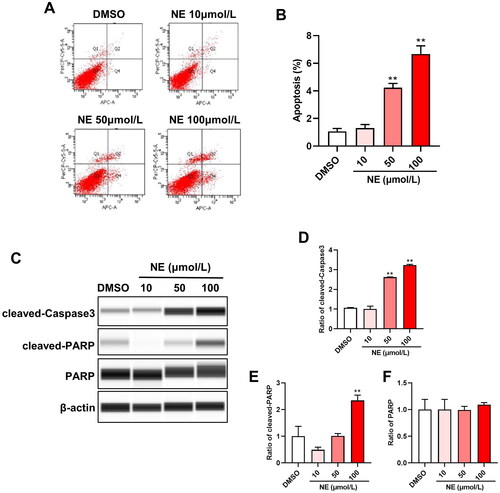
3.2. MALAT1 expression was upregulated in HT22 cells treated with high level of NE
After incubating with different concentrations of NE (10, 50 and 100 μmol/L) for 12, 24 and 48 hours respectively, the expression of MALAT1 in HT22 cells was measured by qRT-PCR. The results showed that the expression of MALAT1 in HT22 cells was significantly upregulated upon 100 μmol/L NE treatment, and the increase was higher at 12 h compared to 24 and 48 h (, One-way ANOVA, A, F3,8 = 7.459, p = 0.0105; B, F3,8 = 10.88, p = 0.0034; C, F3,8 = 10.17, p = 0.0042).
Figure 2. High level of NE-induced MALAT1 expression in HT22 cells. The effect of NE on MALAT expression was quantified by qRT-PCR. (A-C) qRT-PCR assays monitoring expression of MALAT1 in HT22 cells exposed to different concentrations of NE for 12 h (A, F3,8 = 7.459, p = 0.0105), 24 h (B, F3,8 = 10.88, p = 0.0034) and 48 h (C, F3,8 = 10.17, p = 0.0042) (n = 3, One-way ANOVA). * p < 0.05, ** p < 0.01 vs DMSO.
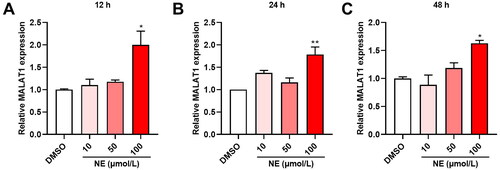
3.3. MALAT1 depletion diminished NE-induced apoptosis of HT22 cells
To explore the significance of MALAT1 in NE-induced cell apoptosis, stable transfectants of HT22 cells expressing shRNA against MALAT1 were established. The knockdown effect was determined by qRT-PCR (, Student’s t-test, t = 8.241, p = 0.0012). Next, flow cytometry and Wes assay were used to evaluate the influence of MALAT1 on cell apoptosis. As shown in , compared with the scramble control, the apoptotic rate was decreased in MALAT1 lower-expressing cells. More intriguingly, the knockdown of MALAT1 abrogated the discrepancy of apoptosis between NE-treated and DMSO-treated HT22 cells (Two-way ANOVA, Interaction: F1,8 = 42.83, p = 0.0002). Consistently, the results of Wes assay showed a reduction in the levels of cleaved-Caspase3 and cleaved-PARP after MALAT1 inhibition. Furthermore, MALAT1 depletion also alleviated the distinct expression levels of cleaved-Caspase3 (Two-way ANOVA, Interaction: F1,8 = 26.44, p = 0.0009) and cleaved-PARP (Two-way ANOVA, Interaction: F1,8 = 9.891, p = 0.0137) between HT22 cells upon NE treatment and DMSO treatment (). Taken together, these results indicated a regulative role of MALAT1 in NE-mediated cell apoptosis.
Figure 3. MALAT1 knockdown alleviated NE-induced cell apoptosis. Lentiviruses were used to establish the cell lines with shMALAT1. The levels of MALAT1 and cells apoptosis were detected in the indicated cells. (A) Knockdown of MALAT1 in HT22-shMALAT cells was validated by qRT-PCR (n = 3, student’s t-test, t = 8.241, p = 0.0012). (B) Representative images of flow cytometry analysis for HT22-shCtrl/shMALAT1 cells upon NE (100 μmol/L) or DMSO treatment. (C) The quantified result of cell apoptosis analyzed by flow cytometry (n = 3, Two-way ANOVA, Interaction: F1,8 = 42.83, p = 0.0002). (D) Expressions of cleaved-Caspase3 and cleaved-PARP in HT22-shCtrl/shMALAT1 cells treated with DMSO or NE (100 μmol/L) were detected by Wes assay. (E-G) The relative expressions of cleaved-Caspase3 (E, Interaction: F1,8 = 26.44, p = 0.0009), cleaved-PARP (F, Interaction: F1,8 = 9.891, p = 0.0137), and PARP (G, Interaction: F1,8 = 5.403, p = 0.0486) proteins were normalized to β-actin (n = 3, Two-way ANOVA). *p < 0.05, **p < 0.01, n.s., no significance.
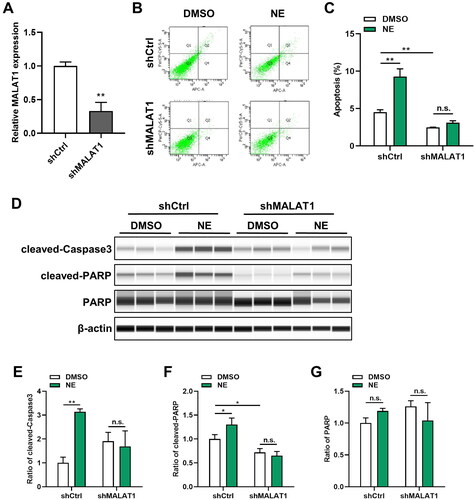
4. Discussion
Stress is a conserved biological and psychological response in all mammals, enabling them to react or adapt to challenging environmental changes (Kass et al., Citation2013). The brain is the central organ for perceiving and adapting to social and physical stressors and is also one of the most vulnerable organs in response to stress (Mariotti, Citation2015). Chronic stress has been recognized as a significant risk factor leading to cognitive impairment and even dementia (Lutz et al., Citation2020; Polis et al., Citation2021), but the underlying mechanism is still unclear. In this study, our results showed that a high level of the stress-related hormone NE promoted hippocampal neuron apoptosis, and the biological effect of NE was attributed to the regulation of MALAT1 expression.
Numerous physiological functions both in fetal tissues and in adult tissues depend on programmed cell death. Necrosis is less common than apoptosis in physiological cell death. Many diseases, including those where cells accumulate (cancer, restenosis) or where cell loss results (stroke, heart failure, dementia, AIDS), are influenced by flaws in the regulation of apoptotic cell death (Ayna et al., Citation2020; Caglayan et al., Citation2022; Kızıl et al., Citation2023). Epidemiological and experimental studies have demonstrated that brain damage, occurs after prolonged periods of stress, (McEwen et al., Citation2015). However, whether chronic stress causes neuron loss or apoptosis remains controversial. Sapolsky et al. reported that chronic stress causes a loss of pyramidal neurons in the hippocampus, accompanied by cognitive deficits in rats (Sapolsky, Citation1985). Some studies also described a reduction of cell numbers in the CA1/3 areas of the hippocampus in stressed rats (Brunson et al., Citation2001; Czeh et al., Citation2005). Nevertheless, there were other studies that did not observe massive neuronal loss or obvious apoptosis following chronic stress in non-human primates or rodents (Pravosudov & Omanska, Citation2005; Vollmann-Honsdorf et al., Citation1997). This discrepancy may be correlated with the intensity of the stressors and the activation degree of their induced neuroendocrinology reaction. Consistently, in this study, we used NE to evaluate the effect of stress on hippocampal neurons and found that the impact of different doses of NE on cell apoptosis varied. The results showed that cell apoptosis was not observed at 10 μmol/L of NE treatment. When the concentration of NE increased to 50 or 100 μmol/L, cell apoptosis was markedly induced, suggesting that the content of stress-induced hormones might be involved in regulating the adaptive or maladaptive consequences of stress.
Chronic stress induces aberrantly persistent activation of the SNS and the hypothalamic-pituitary-adrenal (HPA) axis, leading to the simultaneous elevation of catecholamines and glucocorticoids. The locus coeruleus (LC)-noradrenergic system is the main source of NE in the central nervous system. Stress triggers activation of the LC and interaction with the hippocampus, amygdala and prefrontal cortex, which is involved intensively in modulating stress-related emotional or cognitive disorders (Belujon & Grace, Citation2011; Suarez-Pereira et al., Citation2022). It has been reported that the catecholamine hormone NE can induce apoptosis of cardiomyocytes, endothelial cells and alveolar epithelial cells (Communal et al., Citation1998; Dincer et al., Citation2001; Thakur et al., Citation2015). Mechanistically, NE induced reactive oxygen species (ROS), mitochondrial Ca2+ overload, impaired uncoupled respiration and downregulation of anti-apoptotic protein Bcl-2, finally leading to cell death (Assali et al., Citation2020; de Lima-Seolin et al., Citation2019; Fu et al., Citation2004). Besides, it was reported that NE induced cardiomyocyte apoptosis by upregulating the expression of lncRNA GAS5 and downregulating the level of sema3a protein (Hao et al., Citation2018). In this study, we found that NE promoted the expression of MALAT1 in HT22 cells, while the knockdown of MALAT1 reversed neuronal apoptosis induced by NE. The results provide new experimental data toward a further understanding of the epigenetic mechanisms in the regulation of stress response.
MALAT1, also named Nuclear-enriched autosomal transcript 2 (NEAT2), was first identified in non-small cell lung cancer in 2003 (Ji et al., Citation2003). Due to the widely expressed in many organs, the function of MALAT1 has drawn extensive attention from researchers. Early studies involving MALAT1 mainly focused on tumors, and MALAT1 has been demonstrated to be a key regulator of malignant behaviors such as proliferation, apoptosis, drug resistance and metastasis of tumor cells (Liu et al., Citation2021; Zhang et al., Citation2022). In recent years, increasing evidence showed that MALAT1 plays an important role in the regulation of cognitive function and the progression of neurodegenerative diseases (Zhang and Wang, Citation2019). In Parkinson’s disease (PD) and epilepsy, the level of MALAT1 was found to be elevated, and MALAT1 induced neuronal apoptosis and damage through regulating miR-205-5p/LRRK2 and miR-101/PI3K/AKT axis (Chen et al., Citation2018; Wu and Yi, Citation2018). Here, our data indicated that MALAT1 depletion inhibited neuronal apoptosis and protected cells from NE-induced cell damage. However, the underlying mechanisms still need further exploration.
In summary, we have found that a high level of stress-induced hormone NE promoted hippocampal neuron apoptosis and MALAT1 expression. Besides, the Suppression of MALAT1 relieved neuronal apoptosis caused by NE treatment. Our findings may provide a potential therapeutic target for the development of new strategies to protect people from the detrimental effects of stress on cognitive function.
Disclosure of interest
No potential conflict of interest was reported by the author(s).
Author contributions
LQ and XW designed the study. YW, HH, YW, YZ, FX, and ZS contributed to acquiring the data and performed the statistical analysis. XW and YW drafted the manuscript. LQ and HH performed the revision of the manuscript. XW and HH revised the manuscript. All authors read and approved the final manuscript.
Acknowledgements
The authors would like to thank Shida Wang, Feng Li, Xiaotian Wang in Academy of Military Medicine Sciences for their technical assistance.
Data availability statement
The data and materials in this paper are freely available from the authors upon request.
Additional information
Funding
Notes on contributors
Ying Wang
Ying Wang graduated from Academy of Military Medicine Sciences, Beijing, China, who is currently studying for a master’s degree at Soochow university, Zhejiang, China.
Hui Hu
Hui Hu is a postgraduate student at Academy of Military Medicine Sciences, Beijing, China.
Yuhan Wu
Yuhan Wu is a postgraduate student at Academy of Military Medicine Sciences, Beijing, China.
Yun Zhao
Yun Zhao Ph.D is a professor at Beijing Institute of Basic Medical Sciences, Academy of Military Medicine Sciences, Beijing, China, who is focus on stress-related cognitive impairment.
Fang Xie
Fang Xie Ph.D is a professor at Beijing Institute of Basic Medical Sciences, Academy of Military Medicine Sciences, Beijing, China, who is focus on stress-related metabolic disorder.
Zhaowei Sun
Zhaowei Sun Ph.D is a researcher at Beijing Institute of Basic Medical Sciences, Academy of Military Medicine Sciences, Beijing, China, who is focus on the study of blood-brain barrier.
Xue Wang
Xue Wang Ph.D is a researcher at Beijing Institute of Basic Medical Sciences, Academy of Military Medicine Sciences, Beijing, China, who is focus on the epigenetic regulation of cognition.
Lingjia Qian
Lingjia Qian Ph.D is a principle investigator at Beijing Institute of Basic Medical Sciences, Academy of Military Medicine Sciences, Beijing, China, who is focus on stress-related brain and cardiovascular injury.
References
- Arun, G., Aggarwal, D., & Spector, D. L. (2020). MALAT1 long non-coding rna: functional implications. Non-Coding RNA, 6(2), 22. https://doi.org/10.3390/ncrna6020022
- Assali, E. A., Jones, A. E., Veliova, M., Acín-Pérez, R., Taha, M., Miller, N., Shum, M., Oliveira, M. F., Las, G., Liesa, M., Sekler, I., & Shirihai, O. S. (2020). NCLX prevents cell death during adrenergic activation of the brown adipose tissue. Nature Communications, 11(1), 1. https://doi.org/10.1038/s41467-020-16572-3
- Ayna, A., Ozbolat, S. N., & Darendelioglu, E. (2020). Quercetin, chrysin, caffeic acid and ferulic acid ameliorate cyclophosphamide-induced toxicities in SH-SY5Y cells. Molecular Biology Reports, 47(11), 8535–8. https://doi.org/10.1007/s11033-020-05896-4
- Belujon, P., & Grace, A. A. (2011). Hippocampus, amygdala, and stress: interacting systems that affect susceptibility to addiction. Annals of the New York Academy of Sciences, 1216(1), 114–121. https://doi.org/10.1111/j.1749-6632.2010.05896.x
- Brunson, K. L., Eghbal-Ahmadi, M., Bender, R., Chen, Y., & Baram, T. Z. (2001). Long-term, progressive hippocampal cell loss and dysfunction induced by early-life administration of corticotropin-releasing hormone reproduce the effects of early-life stress. Proceedings of the National Academy of Sciences of the United States of America, 98(15), 8856–8861. https://doi.org/10.1073/pnas.151224898
- Caglayan, C., Kandemir, F. M., Ayna, A., Gür, C., Küçükler, S., & Darendelioğlu, E. (2022). Neuroprotective effects of 18beta-glycyrrhetinic acid against bisphenol A-induced neurotoxicity in rats: involvement of neuronal apoptosis, endoplasmic reticulum stress and JAK1/STAT1 signaling pathway. Metabolic Brain Disease, 37(6), 1931–1940. https://doi.org/10.1007/s11011-022-01027-z
- Cai, L. J., Tu, L., Huang, X. M., Huang, J., Qiu, N., Xie, G. H., Liao, J. X., Du, W., Zhang, Y. Y., & Tian, J. Y. (2020). LncRNA MALAT1 facilitates inflammasome activation via epigenetic suppression of Nrf2 in Parkinson’s disease. Molecular Brain, 13(1), 130. https://doi.org/10.1186/s13041-020-00656-8
- Chen, Q., Huang, X., & Li, R. (2018). lncRNA MALAT1/miR-205-5p axis regulates MPP(+)-induced cell apoptosis in MN9D cells by directly targeting LRRK2. Am J Transl Res, 10, 563–572.
- Communal, C., Singh, K., Pimentel, D. R., & Colucci, W. S. (1998). Norepinephrine stimulates apoptosis in adult rat ventricular myocytes by activation of the beta-adrenergic pathway. Circulation, 98(13), 1329–1334. https://doi.org/10.1161/01.cir.98.13.1329
- Czeh, B., Simon, M., van der Hart, M. G., Schmelting, B., Hesselink, M. B., & Fuchs, E. (2005). Chronic stress decreases the number of parvalbumin-immunoreactive interneurons in the hippocampus: prevention by treatment with a substance P receptor (NK1) antagonist. Neuropsychopharmacology, 30(1), 67–79. https://doi.org/10.1038/sj.npp.1300581
- de Lima-Seolin, B. G., Nemec-Bakk, A., Forsyth, H., Kirk, S., da Rosa Araujo, A. S., Schenkel, P. C., Bello-Klein, A., & Khaper, N. (2019). Bucindolol modulates cardiac remodeling by attenuating oxidative stress in H9c2 cardiac cells exposed to norepinephrine. Oxidative Medicine and Cellular Longevity, 2019, 6325424. https://doi.org/10.1155/2019/6325424
- Dincer, H. E., Gangopadhyay, N., Wang, R., & Uhal, B. D. (2001). Norepinephrine induces alveolar epithelial apoptosis mediated by alpha-, beta-, and angiotensin receptor activation. American Journal of Physiology. Lung Cellular and Molecular Physiology, 281(3), L624–630. https://doi.org/10.1152/ajplung.2001.281.3.L624
- Fu, Y. C., Chi, C. S., Yin, S. C., Hwang, B., Chiu, Y. T., & Hsu, S. L. (2004). Norepinephrine induces apoptosis in neonatal rat endothelial cells via down-regulation of Bcl-2 and activation of beta-adrenergic and caspase-2 pathways. Cardiovascular Research, 61(1), 143–151. https://doi.org/10.1016/j.cardiores.2003.10.014
- Goyal, B., Yadav, S., R. M., Awasthee, N., Gupta, S., Kunnumakkara, A. B., & Gupta, S. C. (2021). Diagnostic, prognostic, and therapeutic significance of long non-coding RNA MALAT1 in cancer. Biochimica et Biophysica Acta. Reviews on Cancer, 1875(2), 188502. https://doi.org/10.1016/j.bbcan.2021.188502
- Griffiths, B. B., & Hunter, R. G. (2014). Neuroepigenetics of stress. Neuroscience, 275, 420–435. https://doi.org/10.1016/j.neuroscience.2014.06.041
- Hao, S., Liu, X., Sui, X., Pei, Y., Liang, Z., & Zhou, N. (2018). Long non-coding RNA GAS5 reduces cardiomyocyte apoptosis induced by MI through sema3a. International Journal of Biological Macromolecules, 120(Pt A), 371–377. https://doi.org/10.1016/j.ijbiomac.2018.08.039
- Ji, P., Diederichs, S., Wang, W., Böing, S., Metzger, R., Schneider, P. M., Tidow, N., Brandt, B., Buerger, H., Bulk, E., Thomas, M., Berdel, W. E., Serve, H., & Müller-Tidow, C. (2003). MALAT-1, a novel noncoding RNA, and thymosin beta4 predict metastasis and survival in early-stage non-small cell lung cancer. Oncogene, 22(39), 8031–8041. https://doi.org/10.1038/sj.onc.1206928
- Jindal, E., & Goswami, S. K. (2011). In cardiac myoblasts, cellular redox regulates FosB and Fra-1 through multiple cis-regulatory modules. Free Radical Biology & Medicine, 51(8), 1512–1521. https://doi.org/10.1016/j.freeradbiomed.2011.07.008
- Kass, M. D., Rosenthal, M. C., Pottackal, J., & McGann, J. P. (2013). Fear learning enhances neural responses to threat-predictive sensory stimuli. Science), 342(6164), 1389–1392. https://doi.org/10.1126/science.1244916
- Kızıl, H. E., Caglayan, C., Darendelioğlu, E., Ayna, A., Gür, C., Kandemir, F. M., & Küçükler, S. (2023). Morin ameliorates methotrexate-induced hepatotoxicity via targeting Nrf2/HO-1 and Bax/Bcl2/Caspase-3 signaling pathways. Molecular Biology Reports, 50(4), 3479–3488. https://doi.org/10.1007/s11033-023-08286-8
- Krizanova, O., Babula, P., & Pacak, K. (2016). Stress, catecholaminergic system and cancer. Stress, 19(4), 419–428. https://doi.org/10.1080/10253890.2016.1203415
- Li, L., Xu, Y., Zhao, M., & Gao, Z. (2020). Neuro-protective roles of long non-coding RNA MALAT1 in Alzheimer’s disease with the involvement of the microRNA-30b/CNR1 network and the following PI3K/AKT activation. Experimental and Molecular Pathology, 117, 104545. https://doi.org/10.1016/j.yexmp.2020.104545
- Liu, S. J., Dang, H. X., Lim, D. A., Feng, F. Y., & Maher, C. A. (2021). Long noncoding RNAs in cancer metastasis. Nature Reviews. Cancer, 21(7), 446–460. https://doi.org/10.1038/s41568-021-00353-1
- Liu, W., Han, J. L., Tomek, J., Bub, G., & Entcheva, E. (2023). Simultaneous widefield voltage and dye-free optical mapping quantifies electromechanical waves in human induced pluripotent stem cell-derived cardiomyocytes. ACS Photonics, 10(4), 1070–1083. https://doi.org/10.1021/acsphotonics.2c01644
- Liu, Y. Z., Wang, Y. X., & Jiang, C. L. (2017). Inflammation: The common pathway of stress-related diseases. Frontiers in Human Neuroscience, 11, 316. https://doi.org/10.3389/fnhum.2017.00316
- Lutz, M. W., Luo, S., Williamson, D. E., & Chiba-Falek, O. (2020). Shared genetic etiology underlying late-onset Alzheimer’s disease and posttraumatic stress syndrome. Alzheimer’s & Dementia, 16(9), 1280–1292. https://doi.org/10.1002/alz.12128
- Ma, J., Wang, R., Chen, Y., Wang, Z., & Dong, Y. (2023). 5-HT attenuates chronic stress-induced cognitive impairment in mice through intestinal flora disruption. Journal of Neuroinflammation, 20(1), 23. https://doi.org/10.1186/s12974-023-02693-1
- Mariotti, A. (2015). The effects of chronic stress on health: new insights into the molecular mechanisms of brain-body communication. Future Science OA, 1(3), FSO23. https://doi.org/10.4155/fso.15.21
- McEwen, B. S., Bowles, N. P., Gray, J. D., Hill, M. N., Hunter, R. G., Karatsoreos, IN., & Nasca, C. (2015). Mechanisms of stress in the brain. Nature Neuroscience, 18(10), 1353–1363. https://doi.org/10.1038/nn.4086
- Polis, B., Karasik, D., & Samson, A. O. (2021). Alzheimer’s disease as a chronic maladaptive polyamine stress response. Aging, 13(7), 10770–10795. https://doi.org/10.18632/aging.202928
- Pravosudov, V. V., & Omanska, A. (2005). Prolonged moderate elevation of corticosterone does not affect hippocampal anatomy or cell proliferation rates in mountain chickadees (Poecile gambeli). Journal of Neurobiology, 62(1), 82–91. https://doi.org/10.1002/neu.20069
- Sapolsky, R. M. (1985). A mechanism for glucocorticoid toxicity in the hippocampus: increased neuronal vulnerability to metabolic insults. The Journal of Neuroscience, 5(5), 1228–1232. https://doi.org/10.1523/JNEUROSCI.05-05-01228.1985
- Suarez-Pereira, I., Llorca-Torralba, M., Bravo, L., Camarena-Delgado, C., Soriano-Mas, C., & Berrocoso, E. (2022). The role of the locus coeruleus in pain and associated stress-related disorders. Biological Psychiatry, 91(9), 786–797. https://doi.org/10.1016/j.biopsych.2021.11.023
- Thakur, A., Alam, M. J., Ajayakumar, M. R., Ghaskadbi, S., Sharma, M., & Goswami, S. K. (2015). Norepinephrine-induced apoptotic and hypertrophic responses in H9c2 cardiac myoblasts are characterized by different repertoire of reactive oxygen species generation. Redox Biology, 5, 243–252. https://doi.org/10.1016/j.redox.2015.05.005
- Vollmann-Honsdorf, G. K., Flugge, G., & Fuchs, E. (1997). Chronic psychosocial stress does not affect the number of pyramidal neurons in tree shrew hippocampus. Neuroscience Letters, 233(2-3), 121–124. https://doi.org/10.1016/s0304-3940(97)00647-2
- Wang, S. D., Wang, X., Zhao, Y., Xue, B. H., Wang, X. T., Chen, Y. X., Zhang, Z. Q., Tian, Y. R., Xie, F., & Qian, L. J. (2022a). Homocysteine-induced disturbances in DNA methylation contribute to development of stress-associated cognitive decline in rats. Neuroscience Bulletin, 38(8), 887–900. https://doi.org/10.1007/s12264-022-00852-7
- Wang, X., Wang, X., Xie, F., Sun, Z., Guo, B., Li, F., Wang, S., Wang, Y., Tian, Y., Zhao, Y., & Qian, L. (2022b). Leucine mediates cognitive dysfunction in early life stress-induced mental disorders by activating autophagy. Frontiers in Cellular Neuroscience, 16, 1060712. https://doi.org/10.3389/fncel.2022.1060712
- Wang, X., Wang, Y., Xie, F., Song, Z. T., Zhang, Z. Q., Zhao, Y., Wang, S. D., Hu, H., Zhang, Y. S., & Qian, L. J. (2022c). Norepinephrine promotes glioma cell migration through up-regulating the expression of Twist1. BMC Cancer, 22(1), 213. https://doi.org/10.1186/s12885-022-09330-9
- Wu, Q., & Yi, X. (2018). Down-regulation of long noncoding RNA MALAT1 protects hippocampal neurons against excessive autophagy and apoptosis via the PI3K/Akt signaling pathway in rats with epilepsy. Journal of Molecular Neuroscience, 65(2), 234–245. https://doi.org/10.1007/s12031-018-1093-3
- Xie, F., Zhao, Y., Ma, J., Gong, J. B., Wang, S. D., Zhang, L., Gao, X. J., & Qian, L. J. (2016). The involvement of homocysteine in stress-induced Abeta precursor protein misprocessing and related cognitive decline in rats. Cell Stress & Chaperones, 21(5), 915–926. https://doi.org/10.1007/s12192-016-0718-0
- Zhang, L., & Wang, H. (2019). Long non-coding RNA in CNS Injuries: A new target for therapeutic intervention. Molecular Therapy. Nucleic Acids, 17, 754–766. https://doi.org/10.1016/j.omtn.2019.07.013
- Zhang, Y., Xiao, Y., Li, G. C., Gong, F. Y., Zhang, X. N., & Hou, K. (2022). Long non-coding RNAs as epigenetic mediator and predictor of glioma progression, invasiveness, and prognosis. Seminars in Cancer Biology, 83, 536–542. https://doi.org/10.1016/j.semcancer.2020.08.016
- Zhang, Z. Q., Wang, X., Xue, B. H., Zhao, Y., Xie, F., Wang, S. D., Xue, C., Wang, Y., Zhang, Y. S., & Qian, L. J. (2021). Chronic stress promotes glioma cell proliferation via the PI3K/Akt signaling pathway. Oncology Reports, 46(3), 202. https://doi.org/10.3892/or.2021.8153