Abstract
Nitric oxide (NO) has been involved in many pathophysiological brain processes. Recently, we showed that neuronal nitric oxide synthase (nNOS)-mediated decrease in NO production is involved in memory impairment induced by chronic mild stress (CMS) in BALB/c mice. Two genetically different inbred murine strains, C57BL/6 and BALB/c, show distinct behavioral responses, neurodevelopmental and neurochemical parameters. Here, we perform a comparative study on CMS effects upon learning and memory in both strains, analyzing the role of NO production and its regulation by protein kinase C (PKC). Stressed BALB/c, but not C57Bl/6 mice, showed a poor learning performance in both the open field and passive avoidance inhibitory tasks. Also, CMS induced a diminished NO production by nNOS, associated with an increment in γ and ζ PKC isoenzymes in BALB/c mice. In C57BL/6 mice, CMS had no effect on NO production, but increased δ and decreased βI PKC isoforms. In vivo administration of a NOS inhibitor induced behavioral alterations in both strains. These results suggest a differential effect of stress, with BALB/c being more vulnerable to stress than C57BL/6 mice. This effect could be related to a differential regulation of NOS and PKC isoenzymes, pointing to an important role of NO in learning and memory.
Introduction
Stress may be defined as a state in which physiological or psychological homeostasis is perturbed. While response to stress is a necessary survival mechanism, prolonged stress can have several repercussions, ranging from impairments in learning and memory to enhanced neuronal cell death (McEwen Citation1999; Palumbo et al. Citation2007). The hippocampus, a limbic area involved in learning and memory, is particularly sensitive to the effects of stress (Kim and Yoon Citation1998). Despite important progress in the study of the biochemical and molecular consequences of stress (Alfonso et al. Citation2006), this complex topic is incompletely understood.
Nitric oxide (NO) has been implicated in many physiological and pathological brain processes including hippocampal responses to stress (Reagan et al. Citation1999). NO is an important neurotransmitter in the central nervous system (Garthwaite et al. Citation1998; Jurado et al. Citation2004). It is synthesized from l-arginine by nitric oxide synthase (NOS). At least three distinct isoforms of NOS have been cloned and located: endothelial NOS (eNOS) and neuronal NOS (nNOS) have calcium-dependent activity and are constitutively expressed in endothelial cells and in neurons, respectively, and a calcium independent subtype known as inducible NOS (iNOS) (Bredt and Snyder Citation1990). NOS-containing neurons are largely distributed in the brain, including the hippocampus (Brown et al. Citation1999).
NOS can be phosphorylated by different kinases, including protein kinase C (PKC), cyclic AMP-dependent protein kinase A, calcium-calmodulin-dependent protein kinase and cyclic GMP-dependent protein kinase (Nakane et al. Citation1991; Bredt et al. Citation1992; Dinerman et al. Citation1994). Thus, PKC phosphorylation has been shown to either stimulate (Nakane et al. Citation1991) or inhibit (Bredt et al. Citation1992; Dinerman et al. Citation1994) NOS activity in vitro. PKC represents a family of serine/threonine kinases that play a central role in signal transduction, as it is involved in the control of numerous cellular processes. In mammals, the PKC enzyme family comprises at least 10 isoforms divided into three subclasses: the conventional or classical PKCs (α, βI, βII, and γ), which are Ca2 + -dependent and activated by diacyl-glycerol and phorbol esters; the novel PKCs (δ, ε, η, and θ), which can be activated by diacyl-glycerol and phorbol esters but are Ca2 + -independent; and the atypical isoforms (ζ and τ/λ) which are unresponsive to both Ca2 + and diacyl-glycerol or phorbol esters (Newton and Johnson Citation1998).
Multiple lines of research implicate PKC in activity-dependent neuronal plasticity and memory formation (Alvarez-Jaimes et al. Citation2005). In addition, other reports suggest that alterations in PKC function contribute to age-related memory deficits (Colombo et al. Citation1997; Colombo and Gallagher Citation2002). Although PKC has been implicated in learning and memory in studies of spatial learning (Paylor et al. Citation1991; Colombo et al. Citation1997), conditioned taste aversion (Yasoshima and Yamamoto Citation1997) and conditioned avoidance (Jerusalinsky et al. Citation1994) in mammalian models the specific contributions of PKC subtypes to learning and memory are not well known.
In a previous report (Palumbo et al. Citation2007), we showed that BALB/c mice exposed to chronic stress had a poor learning performance in both the open field and passive avoidance inhibitory tasks with respect to control mice, which was correlated with a diminished NO production by nNOS and increased γ and ζ PKC isoenzymes. The magnitude of oxidative stress was increased in the hippocampus of chronic mild stress (CMS) mice. Moreover, treatment of control mice with a NOS inhibitor increased oxidative stress and memory impairments, suggesting a novel protective role for nNOS.
In addition, it was observed that C57BL/6 and BALB/c, two genetically different inbred strains of mice, differ from each other in several behavioral responses and in neurodevelopmental and neurochemical parameters. Thus, BALB/c is considered an emotive and anxiogenic strain with low basal locomotor activity and increased stress reactivity, whereas C57BL/6 is considered a non-emotive, non-anxiogenic and active strain in different experimental situations, such as the open-field test or the elevated plus-maze test (Tassin et al. Citation1980; Marona-Lewicka and Vetulani Citation1989; Lepicard et al. Citation2000; Belzung and Griebel Citation2001; Tang et al. Citation2002, Dulawa et al. Citation2004). Concerning neurochemical studies, BALB/c mice show approximately twofold lower levels of serotonin in the forebrain than the C57BL/6 strain (Zhang et al. Citation2004). The concentration of 17-OH-pregnenolone, a neurosteroid associated with behavior such as aggression, adaptation to stress or learning in mice, in whole brain in C57BL/6 was significantly greater than in BALB/c mice (Tagawa et al. Citation2006). Moreover, significantly less maternal care and elevated stress-induced corticosterone level was observed in BALB/c compared to C57BL/6J mice (Priebe et al. Citation2006).
The aim of the present study was to compare the effect of chronic stress in the C57Bl/6 and BALB/c strains of mice. Specifically, we studied the behavioral and learning alterations induced by chronic stress and correlation with changes in both activity and isoform expression of NOS and PKC isoenzymes.
Materials and methods
Animals
Inbred female BALB/c and C57Bl/6 mice were purchased from Facultad de Veterinaria de la Universidad de Buenos Aires. Sixty-day-old mice weighing between 29 and 31 g at the beginning of the experiments were used. Mice were singly housed and maintained on a 12 h light/dark cycle (lights on at 08.00 h, off at 20.00 h), with room controlled temperature (18–22°C). Except as mentioned below, food and water were freely available.
Four experimental groups (28 mice each) of both murine strains were used throughout. Mice were distributed into two groups (n = 14), one group was housed in normal conditions (control) and the other group was subjected throughout the experiment to chronic unpredictable mild stress (CMS-exposed mice).
Chronic mild stress model
The stress scheme was slightly modified from that previously used in rats by Willner et al. (Citation1992) and in mice by Monleon et al. (Citation1995), and consisted of 16 h of water deprivation; two periods of continuous overnight illumination; two periods (7 and 17 h) of 45° cage tilt; one 17 h period in a soiled cage (100 ml water in sawdust bedding); one period (8 h) of food deprivation; one 17 h period of paired housing (mice were always housed in the same pairs, but the location alternated between the home cages of each member of the pair). All the individual stressors used had been classified as “mild” according to the Animals (Scientific Procedures) Act of 1986 (UK legislation). The stressors were scheduled throughout the week, in a similar manner to that previously described (Willner et al. Citation1992; Monleon et al. Citation1995) for 6 weeks. Experimental protocols were approved by the Internal Ethics Committee of the School of Medicine of the University of Buenos Aires, i.e., Comité Institucional para Cuidado y Uso de los Animales de Laboratorio (CICUAL).
Twenty-two mice of each strain and for each condition (control and CMS-mice) were used for behavioral studies and the other for neurochemical studies. Mice were left undisturbed in their home cages 24 h prior to killing by decapitation, without anesthetic, at 10:00 am.
Another experimental group of mice of each strain (n = 48) was distributed between two subgroups (n = 24), one subgroup received the broad-spectrum NOS inhibitor L-NAME (500 mg/l) and the other nothing in the drinking water, as previously described (Palumbo et al. Citation2007).
Open field habituation
Open-field habituation was performed using a rectangular chamber (42 cm × 35 cm × 15 cm) made of gray polyvinylchloride (PVC) (Frisch et al. Citation2005). The floor of the open field was uniformly divided into 30 squares of 7 × 7 cm. Two observers, unaware of the group to which each mouse belonged, recorded the parameters for 5 min. A low level loudspeaker provided a broad spectrum masking noise. After each trial, the apparatus was cleaned with water containing 0.1% acetic acid. The behavioral parameters registered during 5 min sessions were: (1) rearing (vertical activity): the number of times a mouse stood on its hind legs with forelegs in the air or against the wall; (2) locomotion (horizontal activity): the number of horizontal lines an animal crossed; and (3) corner time: the time an animal spent in the four corner squares of the apparatus. Mice were re-exposed to the open field 1 and 24 h after the initial trial. The open field test was performed between 5:00 and 7:00 pm. Because mice demonstrate less exploratory activity in a familiar environment, this simple test assesses the ability of the mouse to learn and remember the open-field chamber. Habituation was estimated as the relative decrease in activity between the first and re-exposures to the open field (Frisch et al. Citation2005).
Passive avoidance
The step-through passive avoidance response was examined between 10.00 am and 14.00 pm each day. The apparatus consisted of two compartments; one was illuminated with a 60 W lamp and the other was dark. The compartments were separated by a guillotine door (20 × 20 mm). Before the first trial, each mouse was allowed to explore both the illuminated and dark compartments freely for 180 s, and the time spent in the dark and light compartment was measured (light–dark distribution test). On the first day, mice were placed in the illuminated compartment. When all four paws were on the grid of the dark compartment, the mouse received a foot-shock of 0.5 mA for 3 s. Mice could escape from the shock only by stepping back into the safe illuminated compartment. Mice were then returned to their home cages. One and 24 h after training mice were tested and the response latency to enter the dark compartment was measured. The latency for mice that did not move into the dark compartment within more than 5 min was taken as 300 s.
NOS and PKC measurements
Measurements were made on hippocampus (left and right sides) dissected from brains removed after decapitation.
Materials
[U-14C] arginine (300 mCi/mmol) was provided by Amersham Biosciences (Piscataway, NJ, USA) and [32P]-γATP (3000 Ci/mmol) by Perkin Elmer, Inc., (Waltham, MA, USA). Dowex AG 50WX-8 was purchased from Bio Rad (Hercules, CA, USA) and DE-52 from Whatman (Brentford, UK) and Co. L-NAME (Ng-nitro-l-arginine methyl ester), GF 109203X (bisindolylmaleimide) and other chemicals were purchased from Sigma Chemical Co. (St Louis, MO, USA).
NOS Activity
NOS activity was determined by measuring conversion of [U-14C] arginine to [U-14C] citrulline as described by Bredt and Snyder (Citation1990). In brief, hippocampi were pre-incubated in 50 mM HEPES buffer, pH 7.4 pre-warmed and equilibrated with 5% CO2 in O2. Then tissues were homogenized by sonication in 1 ml of medium containing 20 mM HEPES (pH 7.4), 1 mM dithiothreytol (DTT), 1 μM leupeptin, 0.45 mM CaCl2, and 0.2 mM phenylmethanesulphonyl fluoride (PMSF) at 37°C for 30 min 5% CO2 in O2 in the presence of [U-14C] arginine (0.5 μCi). In addition, the same buffer without CaCl2 and containing 1 mM EGTA was added to another aliquot of homogenate for iNOS measurement. The reaction was stopped by rapid cooling on ice and the samples were centrifuged at 20,000 g for 10 min at 4°C. Supernatants were passed through 2 ml Dowex AG 50 WX-8 (sodium form) columns. [U-14C] citrulline was eluted with 2 ml of water and quantified by liquid scintillation counting. Calcium dependent activity was estimated as the difference between the activity in the presence and absence of EGTA. As expected, the presence of a broad-spectrum NOS inhibitor L-NAME, completely inhibit NOS activity in all groups of mice (data not shown). Results are expressed as pmoles of [14C] citrulline produced per g of tissue weight in 30 min. In order to study the influence of PKC in the regulation of NOS activity, the effects of in vitro pre-incubation of hippocampus with the PKC inhibitor GF 109203X (10 μM) were analyzed.
PKC activity
Soluble (cytosolic) and particulate (membrane) fractions were obtained from the hippocampus as previously described by Genaro and Bosca (Citation1993). PKC enzyme was purified by filtration through a DE 52 column (3.5 × 0.5 cm). The enzyme was eluted in a buffer containing 120 mM NaCl, 10 mM β-mercaptoethanol, 0.5 mM EGTA, 10 mM HEPES (pH 7.4). PKC activity was assayed in both cytosolic and membrane preparations by measuring the incorporation of 32P from [32P]-γ ATP into histone 1 (H1). Incubations were performed for 30 min at 30°C in a final volume of 85 μl. Final concentrations of the assay mixture were 25 μM ATP (0.4 mCi), 10 mM Mg2 acetate, 5 mM β-mercaptoethanol, 50 μg of H1, 20 mM HEPES (pH 7.4), and unless indicated, 0.2 mM CaCl2 and 10 μg/ml of phosphatidylserine vesicles. The incorporation of 32P into H1 was linear for at least 30 min. The reaction was stopped by the addition of 2 ml of ice-cold 5% trichloroacetic acid, 10 mM H3PO4. The radioactivity retained on GF/C glass-fiber filters after filtration was determined by counting the filters in 2 ml of scintillation fluid. PKC activity was determined after subtracting the incorporation of 32P into H1 in the absence of calcium and phospholipids. Results are expressed as pmoles of phosphate incorporated into substrate per minute by mg of tissue weight.
Western blot analysis
PKC immunoblot analysis from homogenates of hippocampus was performed. A pool of hippocampi from three mice was used to perform each experiment to test all PKC and NOS isoforms. Samples were supplemented with 3 × sodium dodecyl sulfate (SDS) sample buffer [2% SDS, 10% (v/v) glycerol, 62.5 mM Tris–HCl, pH 6.8, 0.2% bromophenol blue, 10 mM 2-mercaptoethanol]. Proteins were separated by SDS–PAGE on 10% polyacrylamide gels and transferred to nitrocellulose membranes. Non-specific binding sites were blocked with blocking buffer (5% non-fat dried milk, containing 0.1% Tween-20 in 100 mM Tris–HCl, pH 7.5 and 0.9% NaCl) for 1 h. Membranes were subsequently incubated with protein G-purified anti-peptide antibodies to the PKC or NOS isoforms (Santa Cruz Biotechnology, Santa Cruz, CA, USA) for 18 h. Antibodies to PKC α, βI, βII, γ, δ, ε, θ, and ζ were used to evaluate the PKC isoenzyme profile. Antibodies to eNOS, nNOS, and iNOS were used to evaluate levels of NOS isoforms. Anti-β-actin antibody (Santa Cruz Biotechnology) was used as a control for equal loading and transfer efficiency. Negative controls were incubated in the presence of immunogenic peptides (2 μg/ml of antibody to 1 μg/ml of peptide). Then membranes were incubated with a monoclonal anti-rabbit or anti-mouse IgG alkaline phosphatase conjugate antibody (Sigma) for 1 h. Immunoreactive bands were visualized using nitroblue tetrazolium and 5-bromo-4-chloro-3-indolyl-phosphate. Full Range Rainbow (Amersham Pharmacia, Amersham Biosciences Argentina, Buenos Aires, Argentina) was used as molecular weight markers. The densitometric analysis was performed using Image J (version 1.33u, National Institutes of Health, Bethesda, MD, USA). NOS and PKC densitometry values were normalized with respect to the actin value corresponding to the same sample and results are depicted as relative intensity (RI) values.
Statistical analysis
Student's t-test for unpaired values was used to determine the level of significance for normally distributed data. Group differences were tested by one-way analysis of variance (ANOVA). When multiple comparisons were necessary after ANOVA, the Student–Newman–Keuls test was applied. Habituation data were analyzed by repeated measures ANOVA. Passive avoidance data were not normally distributed, hence the non-parametric Mann–Whitney U-test was performed. Differences between means were considered significant if p < 0.05.
Results
Differential effect of chronic stress on learning and memory in BALB/c and C57BL/6 mice
Open field
As expected from our previous report, control BALB/c mice displayed habituation to the environment after repeated exposure, as indicated by a decrease in horizontal activity 51% (t = 5.923, p < 0.001; ) and in rearing activity (62%, t = 6.124, p < 0.001), and by the increase in the time that mice stayed in the corner squares 62%, t = 5.898, p < 0.001). The CMS mice showed an increase in the horizontal (t = 3.556, p < 0.01) and vertical (t = 3.005, p < 0.05) activity and a parallel decrease in the corner time during the training (t = 4.523, p < 0.01). In addition, CMS exposure reduced habituation to the open field (). Non-significant differences were observed in locomotion (t = 1.591, NS) and in the corner time (t = 2.035, NS) after repeated exposure. Moreover, a lower decrease in rearing activity was observed (t = 2.703, p < 0.01). By contrast, both control and CMS C57BL/6 mice showed habituation capacity 24 h post-training as indicated by the decrease in the number of line crossings by 57% (t = 3.189, p < 0.01) in control and 58% (t = 5.783, p < 0.001) in CMS C57BL/6 mice; decreases in the number of rearings by 54% (t = 2.974, p < 0.05) in control and 60% (t = 4.56, p < 0.001) in CMS C57BL/6 mice; and by the increase in the time spent in the corner of the cage of 94% (t = 4.89, p < 0.001) in control and 144% (t = 5.336, p < 0.001) in CMS C57BL/6 mice.
Figure 1 Mice behavior in the open field. Locomotion (panel A), corner time (panel B) and rearing (panel C) were measured in BALB/c (clear bars) and C57BL/6 (dark bars) in control (N) (plain bars) and CMS (crossed bars) mice. Results represent the mean ± SEM of 12 mice for each group. +p < 0.05, ++p < 0.01 versus the corresponding control; *p < 0.05, **p < 0.01, ***p < 0.001 versus training.
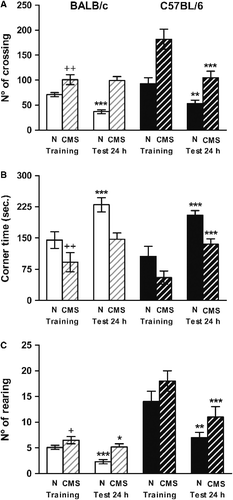
Passive avoidance
In the passive avoidance task, both BALB/c and C57BL/6 mice showed a similar latency to enter the dark compartment during the training phase (data not shown). Twenty-four hours after the foot shock of 0.5 mA the latency to escape to the dark compartment was measured (). The percentage of CMS BALB/c mice that showed the maximal latency to escape to the dark compartment was significantly lower than the control BALB/c mice (20% and 77%, U = 21, p < 0.05). By contrast, the percentage of CMS C57BL/6 mice that showed the lowest latency to escape to the dark compartment was not different from the control C57BL/6 mice (40% and 66.7%, U = 44, NS) ().
Figure 2 Passive avoidance behavior. Escape latency was determined for BALB/c (white) and C57BL/6 (black), control (N) (circle), and CMS (rhombus) mice, 24 h post-training. Each point represents one animal (nine mice per group). Dotted line indicates mean value for each group. *p < 0.05 versus corresponding control.
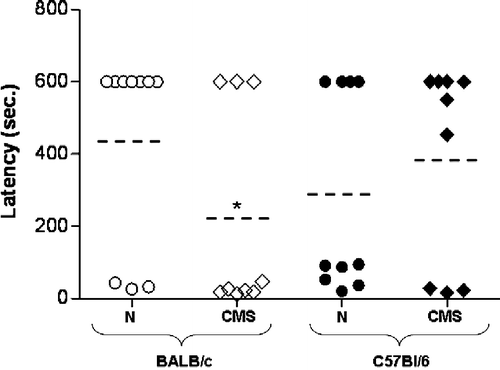
Changes in NOS activity and expression in the hippocampus of BALB/c and C57BL/6 mice induced by chronic stress
Total NOS activity was significantly less in hippocampal homogenates of CMS than in control BALB/c mice (pmol/g tisssue/30 min; t = 11.833, p < 0.0001; ). Control C57BL/6 mice showed a higher basal NOS activity than control BALB/c mice (t = 7.751, p < 0.0001). In CMS C57BL/6 mice, a non-significant difference in NOS activity was observed with respect to control (pmol/g tisssue/30 min, t = 1.176, NS).
Figure 3 Hippocampal NOS activity. Total NOS activity was determined in homogenates of hippocampus from BALB/c (clear bars) and C57BL/6 (dark bars) in control (N) (plain bars) and CMS (crossed bars) mice. Each bar represents the mean ± SEM for eight measurements; each measurement was on the hippocampus (right + left) from a different mouse, n = 8 mice per group. ***p < 0.0001 versus the corresponding control. +++p < 0.0001 respect to control BALB/c mice.
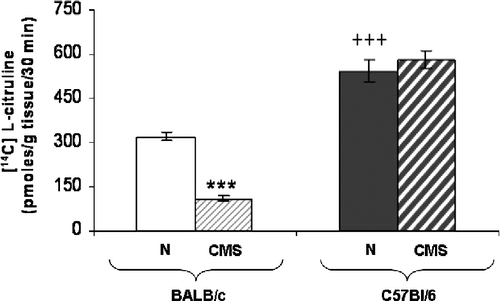
A decrease in hippocampal nNOS protein expression (t = 7.324, p < 0.001) and a smaller increase in hippocampal eNOS expression (t = 2.589, p < 0.05) was detected in CMS BALB/c mice by western blot analysis (). On the other hand, in C57BL/6 mice exposed to CMS there was a smaller decrease in hippocampal nNOS level (t = 2.451, p < 0.05) but no significant difference in eNOS expression (t = 0.008, NS) with respect to control (). It is important to note that according to our previous results (Palumbo et al. Citation2007) both activity and protein expression of iNOS was negligible in all groups tested (data not shown).
Figure 4 Hippocampal nNOS and eNOS expression. Panel A shows a representative western blot of nNOS and eNOS from BALB/c and C57BL/6, control (N) and CMS hippocampus. Panel B shows the densitometric analysis of nNOS and eNOS. Results represent the mean ± SEM of five independent experiments performed with a pool of three hippocampi per experiment, n = 15 mice per group. *p < 0.05, ***p < 0.001 versus control.
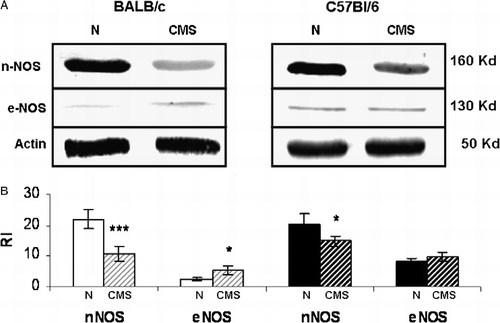
Changes in PKC activity and PKC profile in hippocampus of BALB/c and C57BL/6 mice induced by chronic stress
The total PKC activity in hippocampus of control C57Bl/6 mice was significantly greater than in control BALB/c mice (t = 4.836, p < 0.001; ). Moreover, no significant increase in PKC activity was observed in the hippocampus in CMS BALB/c mice with respect to control BALB/c mice (t = 0.853, NS). In contrast, a significant decrease was found in hippocampal PKC activity of CMS C57BL/6 mice with respect to control C57BL/6 mice (t = 1.799, p < 0.05, one-tailed) (). It is important to note that no significant differences were found in the sub-cellular distribution of PKC between all groups tested (F(3,31) = 1.518, NS).
Figure 5 Hippocampal PKC activity. Total PKC activity was determined in homogenates of hippocampus from BALB/c (left) and C57BL/6 (right) in control (N) and CMS (cross-hatched bars) mice. Results represent the mean ± SEM for eight measurements; each measurement was on the hippocampus (right + left) from a different mouse, n = 8 mice per group. *p < 0.05 versus the corresponding control. +++p < 0.0001 versus control BALB/c mice. Table shows the cytosol and membrane PKC activities (mean % total+SEM) in control (N) and CMS hippocampus.
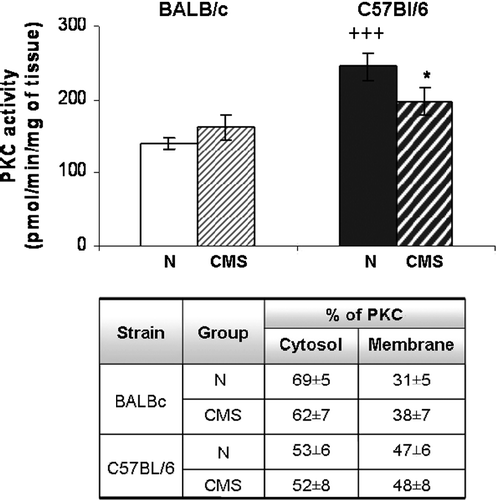
In order to study the influence of PKC in regulating NOS activity the effects of in vitro pre-incubation of hippocampus from control and CMS mice with the PKC inhibitor GF 109203X (bisindolylmaleimide, 10 μmol/l) were analyzed. The data show that calcium-dependent NOS activity in homogenates of control BALB/c mice hippocampus decreased significantly in the presence of PKC inhibitor (t = 3.897, p < 0.01; ) but increased in CMS mice (t = 3.577, p < 0.01). However, in hippocampus of both control and CMS C57Bl/6 mice the PKC inhibitor significantly decreased NOS activity (t = 4.866, p < 0.001 and t = 4.759, p < 0.001, respectively).
Figure 6 Hippocampal PKC regulation of NOS activity. Results show the influence of the PKC inhibitor GF109203X (G) on NOS activity in control (N) and CMS hippocampus from BALB/c and C57BL/6 mice. Results represent the mean ± SEM of eight measurements; each measurement was on the hippocampus (right + left) from a different mouse, n = 8 mice per group. **p < 0.01, ***p < 0.001 respect to basal (B).
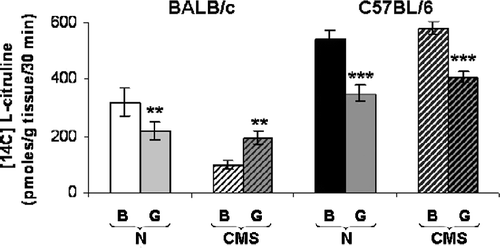
The western blot analysis of PKC isoforms in the hippocampus () revealed that CMS exposure increased ζ (49%, t = 3.626, p < 0.01) and γ (55%, t = 3.111, p < 0.01) PKC isoform expression in BALB/c mice. However, CMS C57BL/6 mice showed an increase in δ (29.61%, t = 2.065, p < 0.05) and a decrease in β1 (29.33%, t = 2.375, p < 0.05) PKC isoforms. No differences were found for the other isoforms tested in both mice strains (data not shown).
Figure 7 Hippocampal PKC isoform expression. Western blot analysis using antibodies for different PKC isoforms was performed on hippocampus from BALB/c and C57BL/6, control (N) and CMS mice. The bands showed are representative of five independent experiments performed with a pool of three hippocampi per experiment, n = 15 mice per group. The histograms show the mean ± SEM of the RI for each isoform. *p < 0.05, **p < 0.01 respect to control.
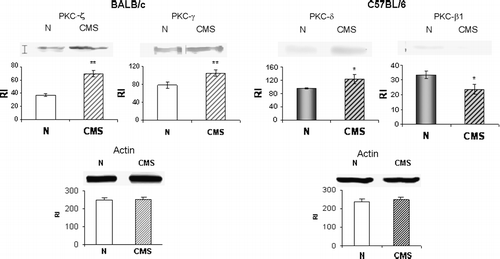
Effects of in vivo treatment with L-NAME in BALB/c and C57BL/6 mice
According to our previous results, NOS inhibition is involved in the behavioral alterations observed in stressed BALB/c mice (Palumbo et al. Citation2007). To investigate if NOS inhibition is able to modify the behavior of C57BL/6 mice, the effect of oral administration of L-NAME to mice for 4 weeks was studied. As expected the administration of the NOS inhibitor in normal BALB/c mice increased locomotor activity [crossing F(3,23) = 19.883, p < 0.0001; rearing F(3,23) = 9.104, p < 0.001; and corner time F(3,23) = 28.978, p < 0.0001; ]. After 1 week of L-NAME administration, BALB/c mice showed an increase in horizontal (t = 6.155, p < 0.001) and vertical (t = 4.637, p < 0.001) activity, and a decrease in the time spent in the corner (t = 6.524, p < 0.001) was found. Moreover, poor memory retention was observed in L-NAME-treated BALB/c mice after 2 weeks. Thus, control mice showed a decrease in locomotor activity when re-exposed to open field 24 h after the initial trial (crossing t = 6.737, p < 0.0001; rearing t = 7.672, p < 0.001; corner time t = 7.082, p < 0.001). However, a significant decrease was only observed in rearing for mice treated with L-NAME for 2 weeks (crossing t = 0.802, NS; rearing t = 2.051, p < 0.05; corner time t = 1.200, NS). Similar results were observed after 4 weeks of treatment (data not shown).
Figure 8 Mouse behavior in the open field after chronic NOS inhibitor treatment (L-NAME). Line crossing, corner time and rearing were measured in the first and second week of L-NAME treatment (L; part-shaded bars) in BALB/c and C57BL/6 mice, and in normal untreated (B) BALB/c (clear bars) and C57BL/6 (dark bars) mice. Results represent the mean ± SEM of six mice for each group. ++p < 0.01,+++p < 0.001 versus corresponding control; *p < 0.05, **p < 0.01, ***p < 0.001 versus training.
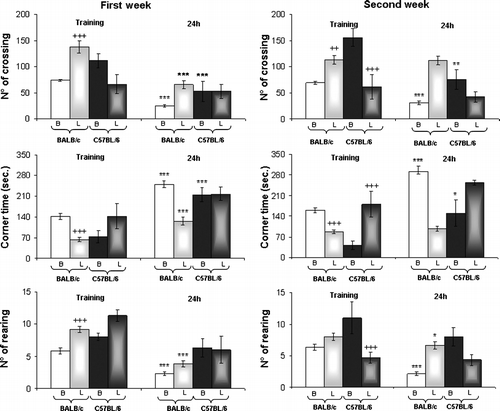
In normal C57BL/6 mice, the inhibition of NOS by L-NAME induced changes in locomotor activity [crossing F(3, 23) = 12.558, p < 0.0001; rearing F(3,23) = 13.892, p < 0.0001 and corner time F(3,23) = 9.361, p < 0.001; ]. Thus, after 2 weeks of treatment a significant decrease was observed in locomotor horizontal activity (t = 5.355, p < 0.001), and in the number of rearings (t = 5.909, p < 0.001) and an increase in the corner time (t = 4.746, p < 0.001). Moreover, control C57BL/6 mice showed a decrease in locomotor activity when re-exposed to the open field 24 h after the initial trial (crossing t = 13.467, p < 0.0001; rearing t = 1.420 NS; corner time t = 10.324, p < 0.0001). No significant changes were observed for C57BL/6 mice treated with L-NAME after 1 week of treatment.
Discussion
The effect of stress on learning and memory has been well documented in a diverse array of species from fish to human (Kim and Yoon Citation1998). A growing body of work suggests that stress could act in many ways to affect the processes that underlie learning and memory.
Memory researchers have identified the hippocampus as a crucial brain structure involved in key aspects of memory formation. Previously, we demonstrated that exposure to chronic stress was able to induce a deficit in learning and memory in BALB/c mice related to a decrease in NO production by nNOS in the hippocampus. Moreover, the results suggested a role for PKC in regulating NOS activity (Palumbo et al. Citation2007). In order to know if the crucial role of NO in learning and memory is confined to BALB/c mice, here we studied the effect of chronic stress and the relation between memory performance and NO production in C57Bl/6 mice. BALB/c and C57Bl/6 mice are two genetically different inbred strains of mice differing from one to another in several behavioral responses and in neurodevelopmental and neurochemical parameters.
Here we exposed animals to the chronic stress model described by Willner et al. (Citation1992) in rats and Monleon et al. (Citation1995) in mice, as previously detailed (Palumbo et al. Citation2007). As expected, the exposure of BALB/c mice to the CMS model increased vertical and horizontal activity in the open field (Silberman et al. Citation2004). Also, according with our previous results (Palumbo et al. Citation2007) CMS BALB/c mice showed a reduced habituation to the open field compared with controls and poor memory retention in a passive avoidance inhibitory task. In contrast, the basal locomotor activity in C57BL/6 mice was increased with respect to BALB/c mice. These results are in accordance with previous findings reported by other authors (Tassin et al. Citation1980; Marona-Lewicka and Vetulani Citation1989). Moreover, we observed that chronic stress did not induce alterations in learning and memory in C57BL/6 mice. Thus, stressed C57Bl/6 mice show a performance similar to control C57Bl/6 mice in both the habituation test and the passive avoidance inhibitory task.
To analyze if memory performance was correlated with NO production we used two approaches: the estimation of in vitro NO production in the hippocampus—a crucial area involved in the processes of learning and memory (Daumas et al. Citation2005) and the behavioral effect of in vivo NOS inhibition. Results showed that C57BL/6 had an approximately twofold greater basal NOS activity in the hippocampus than BALB/c mice. However, both strains showed similar hippocampal NOS protein levels. However, in BALB/c mice stress exposure induced a marked decrease in NO production due to a similar decrease in NOS activity that corresponds with changes in NOS protein levels (Palumbo et al. Citation2007). However, in C57Bl/6 mice stress exposure did not alter significantly NOS activity but decreased hippocampal NOS protein levels. Together these results indicate that there is a different regulation of NOS activity in the two strains. Studies in vitro or in transfected cells have clearly documented that phosphorylation of constitutive NOS by different kinases, including PKC, inhibits the catalytic activity of the enzyme (Dinerman et al. Citation1994; Brown et al. Citation1999). Other authors confirmed a similar effect in intact neurons (Riccio et al. Citation1996; Kim et al. Citation2003). However, NOS activation by PKC has been reported in neuronal cultures (Marin et al. Citation1992) and in vivo studies (Hammer et al. Citation1993). Moreover, Okada (Citation1995) demonstrated that PKC is able to enhance NOS activity at low free calcium concentrations corresponding to levels in resting cells, hence PKC regulates calcium sensitivity of NOS.
In order to study the role of PKC and its interdependency with NO changes, the activity of this enzyme was evaluated. Results showed a higher PKC activity in hippocampus of normal C57Bl/6 mice than in BALB/c mice. However, a similar PKC activity in hippocampus of normal and stressed BALB/c mice (Palumbo et al. Citation2007) and a decrease in PKC activity in CMS C57BL/6 mice with respect to C57BL/6 control mice were observed. Moreover, in BALB/c mice the inhibition of PKC activity led to the inhibition of NOS activity in control, but to an increase in NOS activity in CMS mice (Palumbo et al. Citation2007). In contrast, in C57Bl/6 mice the inhibition of PKC activity led to a decrease in NOS activity in both controls and CMS-exposed. So, it would appear that PKC could be regulating NOS activity differently depending on the mouse strain and the stress exposure. PKC represents a family of at least 10 isoforms that play a central role in signal transduction, being involved in the control of numerous cellular processes. The existence of such a large family of PKC isoenzymes that exhibit different tissue distribution, subcellular localization and biochemical properties, suggests that individual PKC isoforms may play specialized roles in cellular functions. In many cases, PKC isoforms exhibit distinct and even opposing cellular effects (Sossin Citation2007). It is possible that different patterns of PKC isoform expression could be regulated differentially by stress exposure in each strain. In order to analyze this possibility, western-blot analyses using the more important isoforms described in brain (α, βI, βII, γ, δ, ϵ, ζ, θ) were performed. Results showed a significant increase of PKC ζ and PKC γ in hippocampus of CMS BALB/c mice (Palumbo et al. Citation2007) and an increase in delta, but a decrease in βI in CMS C57BL/6 mice. Although a role for the PKC enzyme family is broadly established for various forms of mammalian learning, the specific contributions of PKC subtypes to learning and memory are not well known. Some studies using pharmacological or genetic approaches have investigated the role of specific PKC isoforms operating in learning and memory. Van der Zee et al. (Citation1992) demonstrated that spatial learning induced changes in murine hippocampal PKCγ. Moreover, PKCγ mutant mice deficient in this isoform, exhibited deficit in long term potentation (LTP) in the CA1 area of hippocampus, which correlated with mild deficits observed in spatial and contextual learning (Abeliovich et al. Citation1993). However, Colombo et al. (Citation1997) showed an age-related increase of PKCγ in the particulate fraction of hippocampus suggesting an association with spatial memory impairment among individual aged rats (Colombo et al. Citation1997). Also, increased PKCγ expression was observed in the rat hippocampus following either chronic (8 days) stress (Krugers et al. Citation1997) or chronic dexamethasone treatment (Dwivedi and Pandey Citation1999). However, several authors have provided evidence that βII and ζ PKC isozymes play a role in promoting neuronal cell death in rat hippocampus (McNamara et al. Citation1999; Koponen et al. Citation2003; Tang et al. Citation2004; Bloch-Shilderman et al. Citation2005). Paratcha et al. (Citation2000) showed that hippocampal PKC βI is required in the early phase of memory formation of an inhibitory avoidance learning task. However, other authors (Weeber et al. Citation2000) show that PKC β-deficient C57BL/6 mice do not exhibit deficits in hippocampal synaptic transmission or LTP. There have been no reported effects of changes in synaptic plasticity in PKC δ knockout mice. It is may be that the increase in PKCγ, y, ζ induced by stress exposure in BALB/c mice could contribute to the memory deficit. In contrast, PKC isoform regulation during stress exposure, with an important decrease in PKCβI, may protect C57Bl/6 mice from deleterious effects of stress.
Concerning the effect of NOS inhibitor administration on memory performance, a cognitive deficit in BALB/c mice has been observed after the second week of treatment (Palumbo et al. Citation2007). Similarly, C57BL/6 mice showed poor memory retention after 1 week of NOS inhibitor treatment. These results could be indicating that memory performance in C57BL/6 mice is not affected by stress because NO production is not altered by stress. However, when NO production was inhibited, an impaired memory performance was also observed in C57Bl/6 as in BALB/c mice.
The present data reinforce the important role of NO in learning and memory. Several reports support the idea that a deficiency in NO production is related to impairment in learning and memory and to neurodegeneration. NOS containing neurons are evidently resistant to neurodegeneration in Huntington's disease (HD) and reductions in nNOS mRNA have been reported in this disease (Norris et al. Citation1996). Behavioral studies using the transgenic HD mouse model also suggest that NO may be important to the rate of HD disease progression (Deckel et al. Citation2001). Moreover, Garcia-Arenas et al. (Citation2004) suggest that impairment of memory by lead intoxication in adult animals might be related to a region-specific inhibitory effect on constitutive NOS activity in the hippocampus. Baratti et al. (Citation2008) demonstrated that the administration of L-NAME after the first retention test (memory reactivation) of the inhibitory avoidance response impairs retention performance over six consecutive days. These authors suggest an action of L-NAME on memory reactivation-induced processes that are different from memory extinction of the original learning, extending the biological significance of NO in memory.
The present findings point to an important role for NO in stress related cognitive deficit. The finding that NOS inhibition in normal mice results in memory impairment strengthens this idea. It can be concluded that chronic NOS inhibition by either the administration of NOS inhibitors or CMS exposure would lead to a poor memory performance. It is important to note that there are other signal transduction mechanisms operating in learning and memory. These include the PKC family. However, the possibility that NO production might be regulated differentially by different PKC isoenzymes in brain has not been analyzed either using pharmacological norgenetic deletion approaches. The present findings strongly suggest that altered regulation of PKC activity results in different effects of NOS activity depending on the strain studied and the exposure to stress. It is possible that a particular PKC isoform could increase or decrease NOS activity. Indeed, PKCα plays an important role in LPS-induced NO formation in cardiovascular tissues (Li et al. Citation1998). Similarly, PKCζ isoform expression is associated with NOS activity regulation in a lymphoma cell line (Gorelik et al. Citation2002; Barreiro Arcos et al. Citation2006).
Finally, there are no studies analyzing the effect of regulation of PKC isoform expression on NOS activity and the consequences of this interaction on learning and memory. The present results strongly suggest that PKC-NOS interaction may represent an important and interesting line of future investigation for the development of cognitive therapeutics.
Acknowledgements
The authors thank Maria Rosa Gonzalez Murano for her technical assistance, Daniel Gonzalez for his invaluable help in the animal stress model and Mrs. Ana I. Casella for the secretarial assistance. This work was supported by PIP 6049 from CONICET.
Declaration of interest: The authors report no conflicts of interest. The authors alone are responsible for the content and writing of the paper.
References
- Abeliovich A, Paylor R, Chen C, Kim JJ, Wehner JM, Tonegawa S. PKC gamma mutant mice exhibit mild deficits in spatial and contextual learning. Cell 1993; 75: 1263–1271
- Alfonso J, Frick LR, Silberman DM, Palumbo ML, Genaro AM, Frasch AC. Regulation of hippocampal gene expression is conserved in two species subjected to different stressors and antidepressant treatments. Biol Psychiatry 2006; 59: 244–251
- Alvarez-Jaimes L, Feliciano-Rivera M, Centeno-Gonzalez M, Maldonado-Vlaar S. Contributions of the mitogen-activated protein kinase and protein kinase C cascades in spatial learning and memory mediated by the nucleus accumbens. J Pharmacol Exp Ther 2005; 314: 1144–1157
- Baratti CM, Boccia MM, Blake MG, Acosta GB. Reactivated memory of an inhibitory avoidance response in mice is sensitive to a nitric oxide synthase inhibitor. Neurobiol Learn Mem 2008, Doi: 101016/j.nlm.2007.11.001
- Barreiro Arcos ML, Gorelik G, Klecha A, Genaro AM, Cremaschi GA. Thyroid hormones increase inducible nitric oxide synthase gene expression downstream from PKC-zeta in murine tumor T lymphocytes. Am J Physiol Cell Physiol 2006; 291: C327–C336
- Belzung C, Griebel G. Measuring normal and pathological anxiety-like behaviour in mice: A review. Behav Brain Res 2001; 125: 141–149
- Bloch-Shilderman E, Kadar T, Levy A, Sahar R, Rabinovitz I, Gilat E. Subcellular alterations of protein kinase C isozymes in the rat brain after organophosphate poisoning. J Pharmacol Exp Ther 2005; 313: 1082–1089
- Bredt DS, Snyder SH. Isolation of nitric oxide synthetase, a calmodulin-requiring enzyme. Proc Natl Acad Sci USA 1990; 87: 682–685
- Bredt DS, Ferris CD, Snyder SH. Nitric oxide synthase regulatory sites. Phosphorylation by cyclic AMP-dependent protein kinase, protein kinase C, and calcium/calmodulin protein kinase; identification of flavin and calmodulin binding sites. J Biol Chem 1992; 267: 10976–10981
- Brown LA, Key BJ, Lovick TA. Bio-imaging of nitric oxide-producing neurones in slices of rat brain using 4,5-diaminofluorescein. J Neurosci Methods 1999; 15: 101–110
- Colombo PJ, Gallagher M. Individual differences in spatial memory among aged rats are related to hippocampal PKC gamma immunoreactivity. Hippocampus 2002; 12: 285–289
- Colombo PJ, Wetsel WC, Gallagher M. Spatial memory is related to hippocampal subcellular concentrations of calcium-dependent protein kinase C isoforms in young and aged rats. Proc Natl Acad Sci USA 1997; 94: 14195–14199
- Daumas S, Halley H, Frances B, Lassalle JM. Encoding, consolidation, and retrieval of contextual memory: Differential involvement of dorsal CA3 and CA1 hippocampal subregions. Learn Mem 2005; 12: 375–382
- Deckel AW, Gordinier A, Nuttal D, Tang V, Kuwada C, Freitas R, Gary KA. Reduced activity and protein expression of NOS in R6/2 HD transgenic mice: Effects of L-NAME on symptom progression. Brain Res 2001; 919: 70–81
- Dinerman JL, Steiner JP, Dawson TM, Dawson V, Snyder SH. Cyclic nucleotide dependent phosphorylation of neuronal nitric oxide synthase inhibits catalytic activity. Neuropharmacology 1994; 33: 1245–1251
- Dulawa SC, Holick KA, Gundersen B, Hen R. Effects of chronic fluoxetine in animal models of anxiety and depression. Neuropsychopharmacology 2004; 29: 1321–1330
- Dwivedi Y, Pandey GN. Administration of dexamethasone up-regulates protein kinase C activity and the expression of gamma and epsilon protein kinase C isozymes in the rat brain. J Neurochem 1999; 72: 380–397
- Frisch C, De Souza-Silva M, Söhl G, Güldenagel M, Willecke K, Huston JP, Dere E. Stimulus complexity dependent memory impairment and changes in motor performance after deletion of the neuronal gap junction protein connexin36 in mice. Behav Brain Res 2005; 157: 177–185
- Garcia-Arenas G, Ramirez-Amaya, Balderas I, Sandoval K, Escobar ML, Rios C, Bermudez-Rattoni F. Cognitive deficits in adult rats by lead intoxication are related with regional specific inhibition of cNOS. Behav Brain Res 2004; 149: 49–59
- Garthwaite J, Charles SL, Chess-Williams R. Endothelium-derived relaxing factor release on activation of NMDA receptors suggests role as intercellular messenger in the brain. Nature 1998; 24: 385–388
- Genaro AM, Bosca L. Early signals in alloantigen induced B-cell proliferation. Comparison between B-cell triggering by intact allogeneic cells and solubilized alloantigen. J Immunol 1993; 151: 1832–1843
- Gorelik G, Barreiro Arcos ML, Klecha AJ, Cremaschi GA. Differential expression of protein kinase C isoenzymes related to high nitric oxide synthase activity in a T lymphoma cell line. Biochim Biophys Acta 2002; 1588: 179–188
- Hammer B, Parker WD, Jr, Bennett JP, Jr. NMDA receptors increase OH radicals in vivo by using nitric oxide synthase and protein kinase C. Neuroreport 1993; 5: 72–74
- Jerusalinsky D, Quillfeldt JA, Walz R, Da Silva RC, Medina JH, Izquierdo I. Post-training intrahippocampal infusion of protein kinase C inhibitors causes amnesia in rats. Behav Neural Biol 1994; 61: 107–109
- Jurado S, Sanchez-Prieto J, Torres M. Elements of the nitric oxide/cGMP pathway expressed in cerebellar granule cells: Biochemical and functional characterisation. Neurochem Int 2004; 45: 833–843
- Kim JJ, Yoon KS. Stress: Metaplastic effects in the hippocampus. Trends Neurosci 1998; 21: 505–509
- Kim H, Sasaki T, Maeda K, Koya D, Kashiwagi A, Yasuda H. Protein kinase C beta selective inhibitor LY333531 attenuates diabetic hyperalgesia through ameliorating cGMP level of dorsal root ganglion neurons. Diabetes 2003; 52: 2102–2109
- Koponen S, Kurkinen K, Akerman KE, Mochly-Rosen D, Chan PH, Koistinaho J. Prevention of NMDA-induced death of cortical neurons by inhibition of protein kinase C zeta. J Neurochem 2003; 86: 442–450
- Krugers HJ, Douma BR, Andringa G, Bohus B, Korf J, Luiten PG. Exposure to chronic psychosocial stress and corticosterone in the rat: Effects on spatial discrimination learning and hippocampal protein kinase C gamma immunoreactivity. Hippocampus 1997; 7: 427–436
- Lepicard EM, Joubert C Hagneau I, Perez-Diaz F, Chapouthier G. Differences in anxiety-related behavior and response to diazepam in BALB/cByJ and C57BL/6J strains of mice. Pharmacol Biochem Behav 2000; 67: 739–748
- Li S, Huang FL, Feng Q, Liu J, Fan SX, McKenna TM. Overexpression of protein kinase C alpha enhances lipopolysaccharide-induced nitric oxide formation in vascular smooth muscle cells. J Cell Physiol 1998; 176: 402–411
- Marin P, Lafon-Cazal M, Bockaert J. A nitric oxide synthase activity selectively stimulated by NMDA receptors depends on protein kinase C activation in mouse striatal neurons. Eur J Neurosci 1992; 4: 425–432
- Marona-Lewicka D, Vetulani J. Neurochemical correlates of differences in responses to psychotropic drugs. I. Apomorphine and morphine effects on locomotor activity of C57/BL and Balb/C mice. Pol J Pharmacol Pharm 1989; 41: 431–438
- McEwen BS. Stress and hippocampal plasticity. Annu Rev Neurosci 1999; 22: 105–122
- McNamara RK, Wees EA, Lenox RH. Differential subcellular redistribution of protein kinase C isozymes in the rat hippocampus induced by kainic acid. J Neurochem 1999; 72: 1735–1743
- Monleon S, D'Aquila P, Parra A, Simon VM, Brain PF, Willner P. Attenuation of sucrose consumption in mice by chronic mild stress and its restoration by imipramine. Psychopharmacology 1995; 117: 453–457
- Nakane M, Mitchell J, Forstermann U, Murad F. Phosphorylation by calcium calmodulin-dependent protein kinase II and protein kinase C modulates the activity of nitric oxide synthase. Biochem Biophys Res Commun 1991; 14: 1396–1402
- Newton AC, Johnson JE. Protein kinase C: A paradigm for regulation of protein function by two membrane targeting modules. Biochim Biophys Acta 1998; 1376: 155–172
- Norris PJ, Waldvogel HJ, Faull RL, Love DR, Emson PC. Decreased neuronal nitric oxide synthase messenger RNA and somatostatin messenger RNA in the striatum of Huntington's disease. Neuroscience 1996; 72: 1037–1047
- Okada D. Protein kinase C modulates calcium sensitivity of nitric oxide synthase in cerebellar slices. J Neurochem 1995; 64: 1298–1304
- Palumbo ML, Fosser NS, Rios H, Zorrilla Zubilete MA, Guelman LR, Cremaschi GA, Genaro AM. Loss of hippocampal neuronal nitric oxide synthase contributes to the stress-related deficit in learning and memory. J Neurochem 2007; 102: 261–274
- Paratcha G, Furman M, Bevilaqua L, Cammarota M, Vianna M, de Stein ML, Izquierdo I, Medina JH. Involvement of hippocampal PKCbetaI isoform in the early phase of memory formation of an inhibitory avoidance learning. Brain Res 2000; 855: 199–205
- Paylor R, Rudy JW, Wehner JM. Acute phorbol ester treatment improves spatial learning performance in rats. Behav Brain Res 1991; 45: 189–193
- Priebe K, Romeo RD, Francis DD, Sisti HM, Mueller A, McEwen BS, Brake WG. Maternal influences on adult stress and anxiety-like behavior in C57BL/6J and BALB/cJ mice: A cross-fostering study. Dev Psychobiol 2006; 48(1)95–96
- Reagan LP, McKittrick CR, McEwen BS. Corticosterone and phenytoin reduce neuronal nitric oxide synthase messenger RNA expression in rat hippocampus. Neuroscience 1999; 91: 211–219
- Riccio A, Esposito E, Eboli ML. Modulation by protein kinase C of nitric oxide and cyclic GMP poffation in cultured cerebellar granule cells. Brain Res 1996; 718: 159–164
- Silberman DM, Ayelli-Edgar V, Zorrilla-Zubilete M, Zieher LM, Genaro AM. Impaired T-cell dependent humoral response and its relationship with T lymphocyte sensitivity to stress hormones in a chronic mild stress model of depression. Brain Behav Immun 2004; 18: 81–90
- Sossin WS. Isoform specificity of protein kinase Cs in synaptic plasticity. Learn Mem 2007; 14: 236–246
- Tagawa N, Sugimoto Y, Yamada J, Kobayashi Y. Strain differences of neurosteroid levels in mouse brain. Steroids 2006; 71: 776–784
- Tang X, Orchard SM, Sanford LD. Home cage activity and behavioral performance in inbred and hybrid mice. Behav Brain Res 2002; 136: 555–569
- Tang FR, Lee WL, Gao H, Chen Y, Loh YT, Chia SC. Expression of different isoforms of protein kinase C in the rat hippocampus after pilocarpine-induced status epilepticus with special reference to CA1 area and the dentate gyrus. Hippocampus 2004; 14: 87–98
- Tassin JP, Herve D, Blanc G, Glowinski J. Differential effects of a two-minute open-field session on dopamine utilization in the frontal cortices of BALB/C and C57 BL/6 mice. Neurosci Lett 1980, 1-2: 67–71
- Van der Zee EA, Compaan JC, de Boer M, Luiten PG. Changes in PKC gamma immunoreactivity in mouse hippocampus induced by spatial discrimination learning. J Neurosci 1992; 12: 4808–4815
- Weeber EJ, Atkins CM, Selcher JC, Varga AW, Mirnikjoo B, Paylor R, Leitges M, Sweatt JD. A role for the beta isoform of protein kinase C in fear conditioning. J Neurosci 2000; 20: 5906–5914
- Willner P, Muscat R, Papp M. Chronic mild stress-induced anhedonia: A realistic animal model of depression. Neurosci Biobehav Rev 1992; 16: 525–534
- Yasoshima Y, Yamamoto T. Rat gustatory memory requires protein kinase C activity in the amygdala and cortical gustatory area. Neuroreport 1997; 8: 1363–1367
- Zhang X, Beaulieu JM, Sotnikova TD, Gainetdinov RR, Caron MG. Tryptophan hydroxylase-2 controls brain serotonin synthesis. Science 2004; 305: 217