1. Introduction
In the past decades, numerous studies have attempted to address the various phenomena that take place simultaneously during bone remodeling. These works are based on Frost’s “mechanostat theory” (Frost Citation1987) and postulate the existence of a feedback mechanism between mechanical loading and the biochemical activity of bone cells in bone remodeling. Hence, in the wake of this seminal work, multiple phenomenological laws of varying complexity have been developed to describe the evolution of bone porosity (Beaupré et al. Citation1990; Huiskes et al. Citation2000), tissue orientation (Huiskes et al. Citation2000; Doblaré and García 2001), biological and chemical levels (Komarova et al. Citation2003; Pivonka et al. Citation2008; Klika et al. Citation2014) and mineralization (Hernandez et al. Citation2000; García-Aznar et al. Citation2005; Rouhi et al. Citation2007; Ganghoffer et al. Citation2016).
Here, we propose a novel macroscopic and comprehensive model of bone remodeling considering the influences and interactions of mechanics and biochemistry at the microscale. The evolution of bone tissue is described through its macroscopic deformation as well as the orientation of its microstructure (to be called micro-orientation for short) and bone tissue composition, this latter being encoded in the fraction of osteoid (newly-formed bone matrix) and mineralized bone matrix in a representative tissue (volume) element. Finally, the evolution law relies on the principles of thermodynamics by calculating the energetic contribution and dissipation of the mechanical, biological and chemical processes involved.
2. Methods
We present here a generalized continuum thermodynamics framework for bone remodeling stemming from earlier theories (Germain Citation1973; DiCarlo and Quiligotti Citation2002; Martin et al. Citation2019). The novelty of this contribution concerns the introduction of the mineralization process in the bone remodeling model. The state variables used to describe bone remodeling are the macroscopic deformation, the micro-orientation and—in order to account for the mineralization process—the volume fractions of osteoid and mineralized bone matrix in the bone tissue. Micro-orientation and bone composition are therefore introduced here as textural variables.
In order to derive the evolution laws, we first define the power of external forces (biochemical and mechanical) acting on the system. Additionally, we derive a constitutive law defining the free energy as the sum of the classical mechanical strain energy and a chemical energy depending on tissue composition. Additionally, in the same vein as the free energy formulation, the intrinsic dissipation of the system is assumed to have a mechanical and a biochemical component. Based on these definitions, the evolution laws of the textural variables arise from the second principle of thermodynamics.
Our framework ensures the cross-talk between mechanics and biochemistry and enables the observation of the influence of biological or chemical parameters. Note that bone cells and chemical species in solution are defined here as parameters of the model that can be tuned as functions of the studied system.
3. Results and discussion
In the following benchmark problem, we choose to focus on biochemistry and set the visible deformation null. To this end, we evaluated the influence of different states of bone cells turnover on the evolution of the volume fractions of the osteoid and mineralized matrix (). The simulation depicted in starts with equal fractions of macro-pores, osteoid and mineralized bone (1/3 each) and a concentration of electrolytes corresponding to healthy conditions. Without any cells (dash-dotted lines), the osteoid fraction decreases towards zero (data not shown) while the fraction of mineralized matrix increases, a process referred to as matrix mineralization.
Figure 1. Evolution of the fractions of osteoid (gray) and mineralized bone (black) for systems without cells (dash-dotted), healthy (dashed) or perturbed (solid).
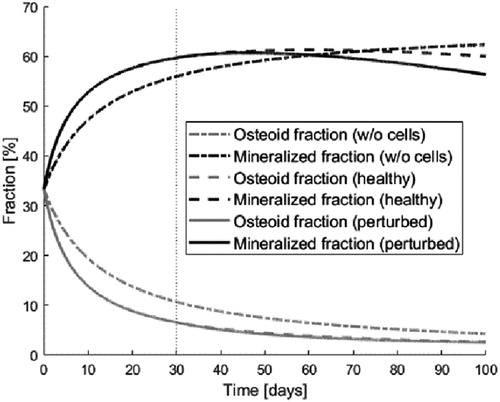
Then, we impose bone cell concentrations and electrolyte concentrations (dashed line) under healthy conditions and increase the cell populations after 30 days by 20% (solid).
We observe here that the increase in remodeling (here, enforced by an augmentation of the turnover) decreases the fraction of mineralized bone. In this illustration, one can clearly observe that the biological activity modulates the chemical evolution.
4. Conclusions
The present work shows the potential of a thermodynamics approach in the context of bone remodeling. Our novel framework includes clinically relevant mechanical and biochemical variables and parameters. Moreover, the wide scope of our model enables the description of the dynamics of bone diseases and their treatment.
While the biochemical contribution of remodeling cells is included in the present study, the latter are only implemented as parameters. However, cells concentrations and their behavior are continuously regulated by their chemical environment. Further work will address this point by following previous numerical studies on mechanobiology and receptor ligand binding regulation in bone remodeling (Pivonka et al. Citation2008).
Additional information
Funding
References
- Beaupré GS, Orr TE, Carter DR. 1990. An approach for time-dependent bone modeling and remodeling-application: A preliminary remodeling simulation. J Orthop Res. 8(5):662–670.
- DiCarlo A, Quiligotti S. 2002. Growth and balance. Mech Res Commun. 29(6):449–456.
- Doblaré M, Garcı́a J.M. 2001. Application of an anisotropic bone-remodelling model based on a damage-repair theory to the analysis of the proximal femur before and after total hip replacement. J Biomech. 34(9):1157–1170.
- Frost HM, 1987. Bone “mass” and the “mechanostat”: A proposal. Anat Rec. 219 2. (1):1–9.
- Ganghoffer JF, Rahouadj R, Boisse J, Forest S. 2016. Phase field approaches of bone remodeling based on TIP. J Non-Equilibrium Thermodyn. 41:49–75.
- García-Aznar JM, Rueberg T, Doblare M. 2005. A bone remodelling model coupling microdamage growth and repair by 3D BMU-activity. Biomech Model Mechanobiol. 4(2-3):147–167.
- Germain P. 1973. La méthode des puissances virtuelles en mécanique des milieux continus. J Mécanique. 12:235–274.
- Hernandez CJ, Beaupré GS, Carter DR. 2000. A model of mechanobiologic and metabolic influences on bone adaptation. J Rehabil Res Dev. 37(2):235–244.
- Huiskes R, Ruimerman R, van Lenthe GH, Janssen JD. 2000. Effects of mechanical forces on maintenance and adaptation of form in trabecular bone. Nature. 405(6787):704–706.
- Klika V, Pérez MA, García-Aznar JM, Maršík F, Doblaré M. 2014. A coupled mechanobiochemical model for bone adaptation. J Math Biol. 69(6-7):1383–1429.
- Komarova SV, Smith RJ, Dixon SJ, Sims SM, Wahl LM, 2003. Mathematical model predicts a critical role for osteoclast autocrine regulation in the control of bone remodeling. Bone. 33 33. (2):206–215.
- Martin M, Pivonka P, Haiat G, Lemaire T, Sansalone V. 2019. Bone orthotropic remodeling as a thermodynamically-driven evolution. J Mech Med Biol. Submitted.
- Pivonka P, Zimak J, Smith DW, Gardiner BS, Dunstan CR, Sims NA, John Martin T, Mundy GR. 2008. Model structure and control of bone remodeling: A theoretical study. Bone. 43(2):249–263.
- Rouhi G, Epstein M, Sudak L, Herzog W. 2007. Modeling bone resorption using mixture theory with chemical reactions. JOMMS. 2(6):1141–1155.