Abstract
One of the possible solutions for the current antibiotic resistance crisis may be found in (often bacteriophage-derived) peptidoglycan hydrolases. The first clinical trials of these natural enzymes, coined here as first-generation lysins, are currently ongoing. Moving beyond natural endolysins with protein engineering established the second generation of lysins. In second-generation lysins, the focus lies on improving antibacterial and biochemical properties such as antimicrobial activity and stability, as well as expanding their activities towards Gram-negative pathogens. However, solutions to particular key challenges regarding clinical applications are only beginning to emerge in the third generation of lysins, in which protein and biochemical engineering efforts focus on improving properties relevant under clinical conditions. In addition, increasingly advanced formulation strategies are developed to increase the bioavailability, antibacterial activity, and half-life, and to reduce pro-inflammatory responses. This review focuses on third-generation and advanced formulation strategies that are developed to treat infections, ranging from topical to systemic applications. Together, these efforts may fully unlock the potential of lysin therapy and will propel it as a true antibiotic alternative or supplement.
Introduction
As was anticipated by sir Alexander Fleming after he discovered penicillin, it did not take long for bacteria to develop resistance against antibiotics (Fleming Citation1945). The excessive use and misuse of antibiotics in both livestock and medical fields have accelerated this process, giving rise to so-called superbugs (WHO Citation2015; O’Neill Citation2016). It is estimated that 700,000 deaths worldwide are attributed to infections by antibiotic-resistant pathogens each year (O’Neill Citation2016). As a consequence, substantial economic losses are suffered (O’Neill Citation2016; Cassini et al. Citation2019; Centers for Disease Prevention and Control Citation2019). We have entered a post-antibiotic era where certain bacterial pathogens are difficult to combat (Centers for Disease Prevention and Control Citation2019).
The economic and financial risks associated with developing new antibacterials have hamstrung the interest of the pharmaceutical sector. Almost all antibiotics used today were discovered between 1940 and 1960, or are derivatives of those compounds (Lewis Citation2013). However, due to the alarming reports concerning antibacterial resistance and a broad societal need, interest in developing antibacterials is re-emerging, primarily in academia and in small and medium-sized companies (WHO Citation2015; O’Neill Citation2016; WHO Citation2017; Theuretzbacher et al. Citation2020). Currently, antibacterials in the clinical pipeline mainly comprise derivatives or combinations of previously existing classes of antibiotics. However, innovative strategies have entered the (pre)clinical pipeline, holding the promise for entirely new approaches to overcome the current challenges (Theuretzbacher et al. Citation2019, Citation2020). These approaches target virulence and involve antibodies or vaccines, but there is also interest in bacteriophages and their components (Theuretzbacher et al. Citation2020).
Bacteriophages, phages, are viruses that infect bacteria. These viruses encode peptidoglycan degrading enzymes, essential for their infection cycle. First, phages carry so-called virion-associated peptidoglycan hydrolases (VAPGHs) as part of their viral particles. VAPGHs locally hydrolyze the bacterial cell wall, allowing the phage to inject its genetic material (Moak and Molineux Citation2004). Secondly, phages require endolysins, expressed inside the bacterial host, at the end of their infection cycle. Membrane permeabilizing holins create access for these endolysins that consequently break down the host peptidoglycan, leading to cell lysis and release of the progeny phage particles (De Smet et al. Citation2017).
At the beginning of this millennium, the first generation of lysins, natural lysins, were explored as antibacterials (). These peptidoglycan degrading enzymes were found to have strong antibacterial activity against Gram-positive pathogens when applied extracellularly (Nelson et al. Citation2001; Jado et al. Citation2003; Loeffler et al. Citation2003). These findings marked lysins as a promising alternative class of new antibacterials (Czaplewski et al. Citation2016). Indeed, some of these first-generation lysins are currently being tested in clinical trials. Particularly, CF-301 is the first lysin to have entered a phase III clinical trial, targeting Staphylococcus aureus in patients with bloodstream infections and/or right-sided infectious endocarditis (ClinicalTrials.gov, NCT04160468). In the case of Gram-negative bacteria, the peptidoglycan layer is shielded by an outer membrane (OM). Nonetheless, some natural lysins were reported to have limited antibacterial activity when applied extracellularly (Morita et al. Citation2001; Lai et al. Citation2011; Wu et al. Citation2019; Kim et al. Citation2020). Orito and co-workers hypothesized this effect is related to the presence of an amphipathic C-terminal region that is responsible for a local OM permeabilization, granting access to the peptidoglycan layer (Orito et al. Citation2004).
Figure 1. Overview of recent advances in engineering and formulation strategies in the lysin field. First-generation lysins constitute native, wild type lysins such as CF-301 and Cpl-1, of which the first one is currently being validated in a phase three clinical trial. Initial lysin engineering studies focused on improving antibacterial and biochemical properties such as an increased bactericidal activity, a better stability, or activity towards Gram-negative pathogens (second generation). A third generation of lysins aims to improve pharmacokinetics and/or pharmacodynamics for optimal implementation of pharmaceutical applications.
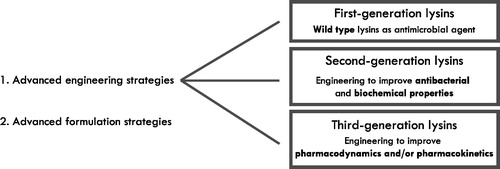
Insights in endolysins structural biology revealed many endolysins have a modular design, comprising at least one enzymatically active domain (EAD) as well as one cell wall binding domain (CBD) (Gerstmans et al. Citation2018). This modular structure served as a critical basis for the second generation of lysins, defined by the implementation of protein engineering techniques, moving beyond natural endolysins (). These lysins were engineered to improve intrinsic antibacterial and biochemical parameters including bactericidal activity, specificity, protein stability, and solubility. Numerous efforts have been undertaken, including site-directed mutagenesis, truncation of lysins, domain shuffling approaches, and fusions with peptides to enhance the above-mentioned parameters, as reviewed recently by Gerstmans and colleagues (Gerstmans et al. Citation2018). One of the main drawbacks of natural (first-generation) lysins was their limited activity towards Gram-negative pathogens. To overcome this barrier, outer membrane permeabilizing peptides were fused to endolysins, as first demonstrated in so-called Artilysins®. These fusion proteins proved highly effective against different Gram-negative bacteria including Pseudomonas aeruginosa and Acinetobacter baumannii, both in vitro, ex vivo, in vivo and against metabolically inactive cells such as persisters (Briers, Walmagh, Grymonprez, et al. Citation2014; Briers, Walmagh, Van Puyenbroeck, et al. Citation2014; Defraine et al. Citation2016). Yet another approach to overcome the OM is inspired by bacteriocins. The fusion of the T4 lysozyme with the region of the toxin pesticin exploiting a TonB-dependent translocation resulted in the specific killing of pathogenic strains of Escherichia coli and Yersinia species (Lukacik et al. Citation2012). A similar strategy resulted in the development of so-called lysocins. In this strategy, colicin-like bacteriocins were modified to comprise a lysin-derived lytic domain to cross the OM exploiting TolC and TolB translocation pathways. This approach was shown to be effective against Pseudomonas aeruginosa both in vitro and in vivo (Heselpoth et al. Citation2019).
These protein engineering efforts often relied on traditional restriction-ligation cloning methods, which are after all cumbersome procedures, limiting the number of engineered variants that can be studied, within a rational design framework. The largest libraries obtained with lysins are in the range of hundreds of variants (Briers, Walmagh, Van Puyenbroeck, et al. Citation2014; Verbree et al. Citation2017; Yang et al. Citation2017). Recent advances now allow us to efficiently assemble tens to hundreds of thousands of engineered proteins using efficient assembly. A recently introduced technique dedicated to the modular assembly of large lysin libraries is the VersaTile technique. As proof of concept, a lysin was engineered and selected from a library of 9576 variants to kill clinically relevant A. baumannii strains in serum conditions (Gerstmans et al. Citation2020). Implementing such approaches can take lysin engineering to the next level with libraries of increasing complexity. By screening these variants for the desired traits, insights in structure-activity relationships can be gained to inspire the construction of more refined libraries in an iterative manner.
Whereas the initial engineering efforts were mainly focussed on antibacterial and biochemical properties of lysins that can be easily evaluated in vitro (second-generation lysins), an increasing number of lysins are being developed to improve pharmacokinetic and/or pharmacodynamic properties relevant under clinical conditions. This branch of lysin engineering is only in its infancy and includes engineering efforts to reduce unwanted effects upon clinical administration. Examples of unwanted effects may include immune responses to the lysin upon (systemic) administration, a limited half-life, and proteolysis on the site of infection due to the presence of inflammatory proteases (Agren et al. Citation2000; Jado et al. Citation2003; Harhala et al. Citation2018). These engineering approaches include both protein engineering and biochemical modifications through covalent attachment of specific groups. Efforts focussing on tackling these hurdles form a basis for what we can consider a third generation of lysins ().
Apart from this first trend of increasingly advanced engineering efforts, a second trend in the field is the increasing attention for the advanced formulation of lysins to increase the bioavailability, antibacterial activity, and half-life, and to reduce pro-inflammatory responses. Depending on the location of the infection in the human body, specific challenges have to be overcome. Within that context, this review will discuss recent advances and efforts made in the field of third-generation lysins and advanced formulation strategies for clinical applications, outlining external and topical uses and the path towards internal and systemic applications. A general overview of the engineering and formulation approaches can be found in and for the main body sites of infection.
Figure 2. Overview of third-generation engineering approaches and advanced formulation strategies. Increasingly advanced engineering efforts are undertaken and advanced formulation strategies are investigated to push the development of lysins as antibacterials. This third generation of lysins aims to improve pharmacokinetics and/or pharmacodynamics with the bulk of the efforts focused on topical, systemic, pulmonary and gastro-intestinal applications. In addition, lysins are also investigated to coat catheter and implant surfaces.
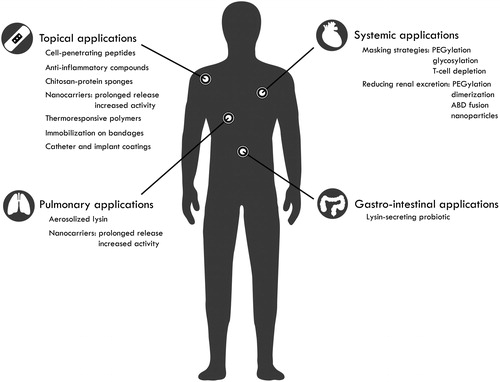
Table 1. Overview of the third-generation engineering and advanced formulation strategies discussed in this review.
Topical applications
The skin is the first protective barrier shielding the body from the external environment. Keratinocytes, the main cell type in the epidermal layer of the skin, are closely joined together by tight junctions to avoid passage of micro-organisms such as bacteria, viruses, or parasites (Heath and Carbone Citation2013). Furthermore, these keratinocytes also produce antimicrobial peptides (AMPs) such as β-defensins to avoid pathogens from entering the human body via the skin (Gallo and Hooper Citation2012). Highly specialized secretory structures such as eccrine glands and sebocytes extend the spectrum of AMPs but also interact with the adaptive immune system making the skin a highly efficient first defence line against pathogens (Gallo and Hooper Citation2012; Heath and Carbone Citation2013). However, injuries or hypersensitivity reactions will disrupt this barrier, providing (opportunistic) pathogens access to the human body.
Skin and soft tissue infections are highly prevalent affecting more than 14 million people in the United States, and are more prevalent than the number of urinary tract infections or pneumonia (Hersh et al. Citation2008; Miller et al. Citation2015). Furthermore, it is estimated that at least 60% of all severe burn wounds become infected by opportunistic pathogens (Diederen et al. Citation2015). In many skin-related infections, prominent opportunistic pathogens are Staphylococci, known for their drug resistance (Otto Citation2009; Diederen et al. Citation2015; Park et al. Citation2017; Shahbazian et al. Citation2017). In general, infections with methicillin-resistant S. aureus (MRSA) yearly causes 10,600 deaths in the United States and is therefore prioritized by both the WHO and the CDC as a pathogen with high priority to develop new drugs (WHO Citation2017; Centers for Disease Prevention and Control Citation2019). Gram-negative bacteria including P. aeruginosa, A. baumannii, and Enterobacteriaceae also play an important role in the morbidity of (burn) wound infections (Park et al. Citation2017; Backes et al. Citation2018; Jabbour et al. Citation2020). These opportunistic pathogens are known for their emerging multi-drug resistance (WHO Citation2017; Centers for Disease Prevention and Control Citation2019; Jabbour et al. Citation2020). As a result, there is an urgent need to develop tailored approaches to combat skin infections. Third-generation engineering or formulation strategies targeting S. aureus have been extensively applied and will be discussed here. Although other (Gram-negative) pathogens frequently occur as well in these types of infections, to our knowledge no third-generation engineering or formulation strategies have been made public to date.
Proof-of-concept studies of third-generation lysins targeting intracellular bacteria
S. aureus can invade eukaryotic cells such as keratinocytes, where they persist and proliferate. Since these cells have reduced metabolic activity, they are more tolerant to antibiotics, rendering standard of care antibiotics ineffective (Soong et al. Citation2015; Leimer et al. Citation2016; Peyrusson et al. Citation2020). Yet, lysins have shown to be effective against persister cells, since their mode-of-action does not require an active metabolism (Briers, Walmagh, Grymonprez, et al. Citation2014; Gutiérrez et al. Citation2014; Defraine et al. Citation2016). In a first attempt to target intracellular S. aureus with lysins, Becker and colleagues fused lysostaphin, LysK, and triple-acting chimeric lysins (K-L and L-K) to eleven different cell-penetrating peptides (CPPs) (Becker et al. Citation2016). Lysostaphin is a peptidoglycan-degrading enzyme secreted by Staphylococus simulans, whereas the chimeric lysins K-L and L-K consist of the lytic domains of LysK and the N-terminal enzymatic domain of lysostaphin, but in opposite orientation (Becker et al. Citation2016). Some fusion proteins of CPPs to lysostaphin cause a significant reduction in intracellular cell counts when tested in a bovine mammary epithelial cell line (MAC-T) even though the enzymatic activities were reduced upon fusion. On the contrary, the addition of a CPP to LysK or the chimeric lysin L-K did not result in enhanced intracellular killing. Surprisingly, the chimeric lysin K-L already had the capacity to kill intracellular S. aureus without a CPP. In a next step, Becker and colleagues tested the efficacy of their approach in a murine mastitis model. In contrast to what was observed in cultured mammary cells, lysostaphin alone already resulted in a significant decrease of the bacterial load, which was not further enhanced by a CPP, whereas the chimeric lysin K-L lost its intracellular killing activity, which was restored by fusing to a CPP, pinpointing the complexity of CPP-mediated uptake in eukaryotic cells (Wang et al. Citation2018).
Wang and colleagues also exploited the transducing activity of CPPs (Wang et al. Citation2018). Three different CPPs were fused to the N- or C-terminus of JDlys, an endolysin originating from phage JD007 infecting S. aureus. Only the N-terminal fusions were observed to retain antibacterial activity, with fusion to the trans-activating transcription factor of HIV type 1 (CPPTat-JDlys) being the most active combination. Using HaCaT keratinocytes, a significantly increased antibacterial effect against intracellular MRSA USA300 was observed. The potential of this fusion approach was further evaluated in a mouse model of cutaneous abscesses. Treatment with CPPTat-JDlys had a better clinical outcome than JDlys, which was also active in vivo. The abscess size was substantially smaller and the bacterial load was approximately 10-fold lower when comparing CPPTat-JDlys and JDlys. The pro-inflammatory cytokine levels (IL-6, TNF-α) are significantly decreased when treating with CPPTat-JDlys compared to the untreated group.
This approach was further refined in a study by Röhrig et al. (Röhrig et al. Citation2020) by selecting first the most active lysins under intracellular and lysosomal conditions from a library comprising 322 native, recombinantly engineered or truncated lysins. Then, N-terminal fusions of the seven most active lysins to six different CPPs were prepared. Whereas all JDlys-CPP fusions were inactive in the study of Wang, lysin-CPP combinations retained antibacterial activity in the work of Röhrig and colleagues (Wang et al. Citation2018; Röhrig et al. Citation2020). They also observed that the trans-activating transcription factor of HIV type 1 (Tat) has the least detrimental effect on the in vitro antibacterial activity. Then, uptake and antibacterial activity of selected Tat-fusion proteins were confirmed in three different cell lines originating from lung epithelia (A549), mice-derived fibroblasts (3T3-L1) and bone fibroblasts (MG-63). All tested proteins were found to be noncytotoxic. As the last step, a murine subcutaneous abscess model was used to successfully confirm the potential of selected Tat-engineered lysins. Moreover, by using a cocktail of Tat-engineered enzymes targeting different peptidoglycan bonds, a synergistic effect was observed in vivo, indicating an optimal strategy to target intracellular S. aureus (Röhrig et al. Citation2020).
In this study, promising results are thus obtained by selecting the right (engineered) lysin, peptide, and cocktail. Nevertheless, these studies also show the difficulties of rational engineering, exemplified by the diverse effects when fusing a peptide to an enzyme and the discrepancy between in vitro and in vivo data. The eventual outcome of fusions is therefore difficult to predict, partially due to the fact that exact mechanisms in which proteins can overcome the eukaryotic membrane are still not completely understood. In addition, some lysins have the capacity of crossing eukaryotic membranes without a fused peptide, such as the chimeric lysin K-L described above (Becker et al. Citation2016) and lysin PlyC active against Streptococcus pyogenes (Shen et al. Citation2016).
Advanced formulation strategies: towards integrated topical delivery strategies of lysins
Besides engineering efforts, several advanced formulation strategies have been explored to improve the topical effect of lysins. These strategies have been mainly applied to first-generation lysins and the bacteriocin lysostaphin. Formulation strategies take other aspects related to infection into account such as inflammation or aim to increase the antibacterial effect of the lysin. To address the first issue, one may co-formulate lysins with anti-inflammatory compounds. For example, a native lysin, LysGH15 was incorporated in an Aquaphor® gel together with apigenin, a plant-derived, anti-inflammatory flavonoid (Duthie and Crozier Citation2000; Byun et al. Citation2013; Lopez-Jornet et al. Citation2014; Li et al. Citation2015; Cheng et al. Citation2018). The efficacy of this treatment was assessed in a murine skin infection model. Gels containing the lysin showed an accelerated wound healing. When apigenin was included, levels of pro-inflammatory cytokines (TNF-α, IL-1β, and IFN-γ) were reduced. Yet, the latter observation did not result in increased wound healing. After wound healing, serum was collected and revealed the presence of antibodies against LysGH15. The development of antibodies did not significantly affect the antibacterial activity of the lysin (Cheng et al. Citation2018). Another strategy uses chitosan-protein sponges loaded with lysostaphin as a wound dressing material. These sponges gradually release the lysin over a 90-min time frame. Depending on the amount of cross-linking agent used, the level of lysin release varied between 30 and 90% of the total amount of lysin applied. This approach was characterized by a 6 months shelf life at 4 °C (Szweda et al. Citation2014). Another study applied a hydrogel consisting of polyethylene glycol (PEG) as a polymer, loaded with lysostaphin. This formulation strategy is not only applicable to topical applications but for more invasive applications as well (Johnson et al. Citation2018). One may also consider thermoresponsive polymers. Upon infection, skin temperature increases, as was observed in chronic leg ulcers (Fierheller and Sibbald Citation2010). A Poly(N-isopropylacrylamide) (PNIPAM) gel was loaded with a cocktail comprising the cysteine, histidine-dependent aminohydrolase/peptidase (CHAP) domain of the endolysin of phage K and lysostaphin. A temperature increase successfully triggered their release (Hathaway et al. Citation2017). These gels may also contain preservatives such as benzalkonium chloride to prolong shelf-life as was patented recently (Vladimirovna et al. Citation2020).
Strategies to increase the antibacterial effect by formulation include the loading of a lytic enzyme in an antibacterial matrix and a system for prolonged lysin delivery. To address the first concept, lysostaphin was formulated in an antimicrobial chitosan gel. In this case, no cytotoxicity was observed against Drosophila melanogaster nor against the macrophage RAW 264.7 cell line (Nithya et al. Citation2018). A way to extend the lysin delivery at the site of infection involves formulation in nano-emulgels. These thermodynamically stable particles show good adhesion to the skin, which in turn leads to an increased bioavailability (Tayeb and Sainsbury Citation2018). The bacteriocin lysostaphin has already been formulated in such nano-emulgels, resulting in a prolonged-release up to 8 h. A treatment regimen including a topical application thrice a day was sufficient to cure 48 h old murine MRSA 300 infected skin abscesses, initially inoculated with 3 × 107 CFU (Nour El-Din et al. Citation2020). Notwithstanding the fact that lysostaphin is a bacteriocin, similar applications could be envisioned using phage-derived lysins or engineered lysins. Yet another interesting nanocarrier is cellulose. This relatively cheap material is an abundant alternative to nano-emulsions (Habibi et al. Citation2010). A proof-of-concept was provided by immobilizing T4 lysozyme and hen egg-white lysozyme on these nanocarriers. These cellulose nanoparticles expanded the range of pathogens that could be targeted and increased the antibacterial effect of the lysin (Abouhmad et al. Citation2017). Moreover, cellulose nanocarriers were shown to be non-cytotoxic, confirming their potential as a safe administration strategy (Jackson et al. Citation2011). Other than using nanoparticles, lytic enzymes may also be used to coat liposomes containing standard of care antibiotics, as has been shown for the combination of lysostaphin and vancomycin that resulted in a powerful synergistic treatment against MRSA (Hajiahmadi et al. Citation2019). Conventional formulation techniques such as incorporation in gels, creams or sprays may also be explored but may impact the enzymatic activity of the (engineered) lysin, and also change some critical physicochemical characteristics such as pH and viscosity as previously observed for such formulations of proteases (Yudanova and Reshetov Citation2006). An alternative approach relies on engineering of the lysin. Since most wound dressing materials contain cellulose, T4 lysozyme was anchored to cellulose using a cellulose-binding module. This module originates from bacterial and fungal cellulases to efficiently bind cellulose (Shoseyov et al. Citation2006). The fusion of a cellulose-binding module from Cellulomonas fimi to the T4 lysozyme resulted in immobilization of 5.5 µg protein/mg of bandage without being leached out by washing. Although the enzymatic activity of this lysin was lowered by the fusion, E. coli counts were reduced by 1.59 log units (Abouhmad et al. Citation2016). Besides using a cellulose-binding domain, also linkage chemistry can be used to functionalize cellulose fibres with lysins. Miao and colleagues linked lysostaphin to three different types of cellulose fibres: a pure cellulose fibre, a cellulose-chitosan fibre, and a cellulose-poly(metylmethacrylate) fibre (Miao et al. Citation2011). Out of these three, the functionalized cellulose fibre performed best, and resulted in the complete contact-mediated killing of a 106 CFU/mL S. aureus suspension. Additionally, no significant cytotoxicity of these fibres was observed in a HaCaT monolayer model (Miao et al. Citation2011). This approach could be straightforwardly adapted towards lysins active against other clinically relevant wound colonizers such as S. aureus and P. aeruginosa. Furthermore, as is the case for other fusion concepts described above, high-throughput engineering would enable screening for the best variant from a library containing various cellulose binding domains, lytic domains or other domains of interest.
On a critical note, all of these concepts were evaluated in a murine in vivo model of skin infection. However, murine skin structure significantly differs from that of human skin. Firstly, mice have a thinner epidermis and dermis compared to humans. In addition, murine skin is covered with hair that is being renewed every three weeks, whereas the hair cycle in humans lasts up to several years (Wong et al. Citation2011). Wound closure also progresses in a different manner (Zomer and Trentin Citation2018), which limits the translational potential of the results obtained from this model. From all animal skin types, porcine skin resembles human skin the most, making them most suitable for skin and wound research (Abdullahi et al. Citation2014). However, only ex vivo pig skin models have been implemented so far in the lysin field (Nithya et al. Citation2018; Gerstmans et al. Citation2020). Practical and financial considerations often explain the choice for a murine model (Zomer and Trentin Citation2018).
Lysin coatings for implanted devices and catheters
Notwithstanding the fact that the introduction of non-living materials in the human body for medical applications is successful in the majority of the cases, there is an imminent risk of bacterial colonization (Busscher et al. Citation2012). As a result, strategies to avoid colonization on these surfaces have been developed, some of which involve lysins. The first step in this field was taken by immobilizing lysostaphin successfully on two different polymers frequently used for catheters: polystyrene and FEP (Shah et al. Citation2004; Yeroslavsky et al. Citation2015). The same antibacterial enzyme was covalently linked to a titanium plate, which was analyzed in a murine implant-associated bone infection model. Seven days after infection, the lysostaphin-coated implant contained a significantly reduced bacterial load. After 28 days, complete fracture healing was observed for this group (Windolf et al. Citation2014). Besides lysostaphin, PlySs2 and SAL-1 were immobilized on self-assembling spider silk proteins, resulting in an effective antistaphylococcal fibre (Nilebäck et al. Citation2019; Seijsing et al. Citation2020). In addition, one can target biofilm formation specifically combining lysins with matrix-degrading enzymes such as Dispersin B (Seijsing et al. Citation2020).
Mucosal applications
Several sites of the human body that are exposed to the external environment are covered with the mucosal layer as is the case for both the respiratory and the urogenital tract, intestinal and ocular surfaces. These sites are hotspots for bacterial colonization and infection. No specific engineering efforts have been undertaken to improve the treatment of mucosa-related infections, but advances in formulation strategies for pulmonary and gastro-intestinal applications have been made.
Formulation strategies delivering lysins to pulmonary surfaces
Lysins have been evaluated to treat lung infections. A prominent pathogen in these cases is Streptococcus pneumoniae (Mandell Citation2015). One of the first lysins described targeting S. pneumoniae is Cpl-1 (Loeffler et al. Citation2003). This enzyme was aerosolized to deliver the natural lysin deep into the lungs of mice suffering from pneumonia. In this way, the infection was cleared, and potentially systemic bacteraemia could be prevented. However, treatment with aerosolized Cpl-1 resulted in a significant increase in pro-inflammatory cytokines IL-1β and IL-6 (Doehn et al. Citation2013). Another approach includes the loading of Cpl-1 on antibacterial chitosan nanoparticles (Gondil et al. Citation2020). This approach displayed low cytotoxicity towards A549 lung epithelial cells. However, an increased IgG immune response was observed upon intranasal administration compared to the response upon the unloaded nanoparticles or the lysin alone. The advantage of this delivery strategy is a gradual release of the lysin over 20 h, resulting in a longer bioavailability at the site of infection (Gondil et al. Citation2020).
Designer probiotics encoding lytic enzymes
Probiotics can be engineered to target specific pathogens in the gut by secreting lysins. In this application, the narrow activity of lysins is of great interest. Selecting the appropriate lysin results in targeting the desired pathogens, while not affecting the microbiota. A proof-of-concept was shown in chicken. The probiotic strain Lactobacillus johnsonii was engineered to secrete the CP25L endolysin, targeting Clostridium perfringens (Gervasi et al. Citation2014). The latter is a Gram-positive pathogen, able to secrete toxins resulting in food poisoning (Lindström et al. Citation2011). Similarly, a patent was filed claiming the use of probiotic strains to secrete peptidoglycan hydrolases to treat Clostridium difficile-associated diarrhoea (Graf Citation2019). The latter species is the leading cause of antibiotic-associated diarrhoea in the Western world (Karen and John Citation2011). A different patent application describes the use of the Enterococcal SagA antigen with lytic activity recombinantly produced and secreted from probiotic Lactobacilli (Hang et al. Citation2016). Indeed, SagA was observed to protect Caenorhabditis elegans against Salmonella pathogenesis (Rangan et al. Citation2016).
Besides applications in the gut, the use of a probiotic strain has also been investigated to prevent genital tract infections. A proof-of-concept was established by Liu and colleagues by successfully integrating a gene coding for lysostaphin together with a signal sequence and a pH-responsive promotor into the genome of Lactobacillus plantarum WCFS1, a common colonizer of the vaginal tract (Liu et al. Citation2011). Indeed, during menstruation, the pH rises facilitating S. aureus proliferation in the vaginal tract (Beller and Schweppe Citation1979). Together with elevated protein, oxygen, and carbon dioxide levels, S. aureus will produce toxins resulting in toxic shock syndrome (Mccormick et al. Citation2001). Using this engineered probiotic, S. aureus growth could be limited, lowering the risk of developing toxic shock syndrome.
Although the concept of equipping probiotics with lysins is promising, engineered living therapeutics should encode biocontainment mechanisms to prevent the spread of recombinant DNA in the environment. To date, only a limited number of such mechanisms have been constructed for probiotic applications. An interesting concept is the introduction of a temperature-dependent kill switch: when leaving the body, the probiotic is no longer able to proliferate (Pedrolli et al. Citation2019). Notwithstanding synthetic biologists have been expanding the toolbox to engineer living therapeutics over the past few years, important tools are still missing resulting in challenges to engineering these strains (Pedrolli et al. Citation2019). Lysins should also be carefully selected to exclude toxicity against the producing probiotic bacterium.
Systemic applications
Compared to topical and mucosa-related infections, the treatment of systemic infections faces additional hurdles. Upon systemic administration of a proteinaceous therapeutic such as lysins, the development of an immune response is imminent, potentially affecting the clinical outcome. Indeed, a diminished activity due to the development of antibodies, or worse, a hypersensitivity reaction resulting in anaphylaxis are critical points of attention. summarises the lysins evaluated in murine bacteraemia models, including the immune response when reported ().
Table 2. Overview of lysins evaluated in a murine model of bacteraemia.
Biochemical modifications and engineering to reduce the immune response
Some studies indicate that this immune response not necessarily leads to diminished antibacterial activity of the lysin in acute infections (Loeffler et al. Citation2003; Schuch et al. Citation2014; Zhang et al. Citation2016; Cheng et al. Citation2017). However, to avoid the risk of provoking a hypersensitivity response or to avoid inhibition by antibodies, a number of so-called “masking” strategies have been developed. These include PEGylation, glycosylation, and T-cell epitope depletion and may be generally exploited to develop third-generation lysins.
One of the first masking strategies implemented with lysins is PEGylation. Briefly, polyethylene glycol (PEG) molecules are covalently linked to certain amino acid residues such as cysteine or lysine (Turecek et al. Citation2016). In 2003, lysostaphin was the first lytic enzyme to be PEGylated resulting in a tenfold reduction in binding affinity for anti-lysostaphin antibodies. However, in vitro antibacterial activity was observed to be reduced as well (Walsh et al. Citation2003). Nonetheless, a longer half-life could compensate for this diminished activity in vivo. PEGylation can also lead to complete inhibition of antibacterial activity, as was observed with Cpl-1 (Resch et al. Citation2011b). This study used cysteine-specific PEGylation, but a different way of PEGylation such as lysin-specific PEGylation with PEG tresylate or other commercially available PEG reagents may affect antibacterial activity to a lesser extent (Turecek et al. Citation2016). After all, some patients develop anti-PEG antibodies as well. Recently, a gene locus was identified to be associated with the development of IgM antibodies against PEG (Chang et al. Citation2017). This finding allows the prediction of a potentially adverse outcome upon treatment with PEGylated therapeutics enabling a patient-specific approach.
Glycosylation of the therapeutic protein could also be used for masking and avoiding an immune response. Human proteins are glycosylated, a posttranslational modification in which carbohydrate molecules are covalently bound to the polypeptide chain on specific locations. More specifically, N-glycosylation always occurs on an Asn residue in an Asn-X-Ser/Thr sequence, whereas O-glycosylation primarily targets Ser-, Tyr- or Thr-residues (Héver et al. Citation2019). The lack of glycosylation leads to a shorter half-life, partially due to proteolysis, as was observed with therapeutic erythropoietin and IFN-α (Ceaglio et al. Citation2010; Kiss et al. Citation2010). Epitopes generated in this proteolysis process can be presented in MHC-complexes leading to T-cell activation, finally leading to the production of specific antibodies by mature B cells (Blum et al. Citation2013). In this regard, it is useful to consider eukaryotic systems for lysin production such as Saccharomyces cerevisiae. In contrast to E. coli, S. cerevisiae is classified as a GRAS organism (generally recognized as safe) by the FDA for recombinant expression of therapeutic proteins. This obviates an endotoxin removal protocol. Although S. cerevisiae offers only a basic set of glycosylation resulting in a more simplified glycosylation pattern compared to humans, this limited glycosylation could result in limited proteolysis and hence a longer half-life (Doering et al. Citation2017). Other eukaryotic systems such as Chinese hamster ovary (CHO) cells could be used to obtain glycosylated therapeutics (Solá and Griebenow Citation2010; Clausen et al. Citation2017). However, there is no guarantee for success: an attempt to glycosylate lysostaphin rendered the bacteriocin ineffective (Huang et al. Citation2013). The authors suggested an N-glycosylated Asn residue situated in the catalytic site to be responsible for the loss of the enzymatic activity. Therefore, insights in lysin structure and in silico prediction of glycosylation sites could potentially give an idea of the potential of glycosylation for a lysin of interest.
A third masking approach focuses on avoiding or reducing the presentability of proteolytic peptides, so-called epitopes, of lysins in MHC complexes (depletion of T-cell epitopes). Epitopes presented in MHC complexes are about 10 amino acids in length (Blum et al. Citation2013). Furthermore, algorithms, data transformations, and machine learning approaches revealed MHC peptide-binding domains, resulting in the development of T-cell epitope mapping tools (El-Manzalawy et al. Citation2011; Paul et al. Citation2015). As a result, it is possible to digest a peptide (i.e. the lysin of interest) in silico and screen it for potential immunogenicity using mapping tools. Upon detection of potential immunogenic regions, amino acids can be changed to reduce immunogenicity with minimal changes in protein conformation. Both aspects were incorporated into a single protein design tool, called EpiSweep (Parker et al. Citation2013). A deimmunization proof-of-concept was achieved for lysostaphin (Blazanovic et al. Citation2015; Zhao et al. Citation2015). This approach generated variants that were less immunogenic compared to wild type lysostaphin. However, these variants also showed reduced antibacterial activity. Two lead variants were selected: Opt4 and Lib5, the first being less immunogenic, but also losing 99.75% of its antibacterial activity. In contrast, Lib5 was only slightly more immunogenic than Opt4, but retained 50% of its antibacterial activity. Using two different murine models, Zhao and colleagues showed the deimmunization approach to be specific for the human MHC complex, responsible for antibody generation (Zhao et al. Citation2015). One murine model expressed an endogenous murine MHC complex, whereas the other group expressed a chimeric human MHC complex. In mice expressing the human MHC complex, the deimmunized lead Lib5 elicited a reduced immune response, which was not the case in mice expressing the murine MHC complex. In addition, they showed that for a recurring bacteraemia model in which mice are challenged and treated again on a weekly basis, the antibody titre against both proteins increased after each treatment. Since Lib5 is a less immunogenic variant, the titre was substantially reduced compared to titre observed in animals treated with wild type lysostaphin. As a result, wild type lysostaphin treatment was unable to save any mice in a second infection. In contrast, Lib5 treatment could rescue two out of three animals, which also survived a third infection and treatment cycle. It should be noted that the one that did not survive the second challenge, developed antibodies in the same extent as wild type lysostaphin (Zhao et al. Citation2015). With this experiment, the potential of deimmunized variants for treatment in recurring infections is clear. This approach has not yet been described for other lysins but a patent describing deimmunized variants of PlySs2 (CF-301), the lead variant of a US-based company, was filed (Schuch et al. 2018).
Third-generation lysins to increase the lysin half-life by reducing the renal filtration rate
Upon systemic administration, lytic enzymes appear to be characterized by a short half-life. Indeed, individual cases of recorded half-life to date were shown to be 20.5, 22.5, and 60 min, respectively (Loeffler et al. Citation2003; Walsh et al. Citation2003; Jun et al. Citation2017). The kidney plays an important role in this regard: glomerular filtration filters proteins smaller than 40–50 kDa into the excretion fluid (Kontermann Citation2011). Since lysins are often smaller than this cut-off value, a significant portion of therapeutic protein is likely to be cleared from the body in this way. Third-generation engineering approaches can address this hurdle.
The first strategy to reduce the influence of renal filtration relied on increasing the hydrodynamic volume. By increasing the capacity to bind water molecules, particle size will increase as well. As a result, renal filtration is hampered. Hydrodynamic volume increase can be achieved by conjugating the lysin with water-binding polymers such as PEG (Turecek et al. Citation2016). This strategy was applied to lysostaphin leading to an increase in half-life up to 24 h, whereas 95% of the unPEGylated enzyme was cleared after 1 h (Walsh et al. Citation2003). An in-depth analysis of the effects of PEGylation on Hen egg-white lysozyme has been published, revealing that PEGylation results in a two- to fourfold increase in Stokes or hydrodynamic radius depending on the type of PEG polymer that was used (Gokarn et al. Citation2012). As such, PEGylation could increase the half-life of lysins by acting on different concepts: evading the immune system as was described above and reduced glomerular filtration by increasing the hydrodynamic volume of the particle. However, it should be noted that this approach could result in an inactive lysin and will impact the production efficiency and cost of such products (Resch et al. Citation2011b).
A more straightforward approach to reduce glomerular filtration involves dimerization of the lysin. This strategy was applied to the pneumococcal lysin Cpl-1 by introducing cysteine-residues at the C-terminus of the lysin (Resch et al. Citation2011a). In this manner, stable dimers of 74 kDa in size could be obtained, now larger than the renal filtration cut-off (40–50 kDa), and correspondingly resulting in a tenfold increased half-life. Interestingly, applying also doubled the enzymatic activity. This could be explained by the presence of two catalytic sites. It should be noted that the latter was observed in vitro only; whether this also contributes to higher anti-pneumococcal activity in vivo should be examined. Another approach of dimerization was followed for the lytic bacteriocin lysostaphin. There, an α-helical dimerization domain was used to obtain dimeric lysostaphin. This approach resulted in a doubled half-life, albeit a reduction in in vitro antibacterial activity, reflected by a 32-fold increase in minimum inhibitory concentration (MIC), was observed as well (Grishin, Lavrova, et al. Citation2019). An in vivo experiment in which systemically infected mice are treated with the two variants of the bacteriocin could reveal whether the prolonged residence time of the dimeric lysin compensates for the reduced in vitro activity.
An alternative approach was followed by Seijsing and colleagues, relying on a fusion to an albumin-binding domain (ABD) (Seijsing et al. Citation2018). This 5 kDa-domain binds albumin with high affinity, and engineering efforts increased its binding affinity (Jonsson et al. Citation2008). Interestingly, albumin is protected from catabolic turnover by exploiting the FcRn pathway, resulting in a longer half-life of the protein (Chaudhury et al. Citation2003). After fusion to the ABD, the lysin will bind albumin and be recycled by the same complex pathway, thus increasing its half-life. Moreover, due to association with human serum albumin, a protein of 67 kDa, a large hydrodynamic volume is created, resulting in reduced glomerular filtration (Chaudhury et al. Citation2003; Kontermann Citation2011). Seijsing et al. focussed on LysK, a lysin derived from S. aureus phage K comprising an N-terminal CHAP domain, a central amidase-2 domain, and a C-terminal SH3b. They incorporated the ABD at different sites of the lysin. More specifically, they not only made N-terminal and C-terminal fusions but also inserted the ABD in between the CHAP and amidase-2 domain and in between the amidase-2 and the SH3b domain. Furthermore, they also removed the amidase-2 domain, as this domain contributed little to the overall enzymatic activity of the lysin (Becker et al. Citation2009). In vitro, the engineered variant lacking the amidase-2 domain and comprising the ABD in between the CHAP and SH3b domains showed the highest muralytic activity of all variants. This engineered variant could reduce S. aureus numbers by three log units within an hour in a time-kill assay, while a control lacking the ABD resulted in a four-log unit reduction of the bacterial count. However, an in vivo experiment revealed the added value of the ABD. Whereas the half-life of the CHAP-SH3b construct was 23 h, the engineered variant had a half-life of 34 h (Seijsing et al. Citation2018). Subsequently, the enzyme can exert its (slightly lower) antibacterial activity for a longer period of time, potentially enhancing its antibacterial effect. The same approach was used to successfully extend the half-life of lysostaphin (Grishin, Shestak, et al. Citation2019).
The effects of these interesting engineering efforts prolonging the half-life are tested in a murine model, but differences may exist between different test organisms. As an example, lysin SAL-200 showed a rather long half-life in monkeys (up to 9 h) whereas the half-life in humans was short (maximal 0.38 h) (Jun et al. Citation2016, Citation2017). Nonetheless, the potential of these approaches is clear despite this translational hurdle.
Conclusion & future perspectives
Over the past decade, the potential of lysins in fighting emerging antibiotic resistance has become clear. Due to their rapid action, anti-persister activity, recalcitrance towards resistance development and progress in clinical trials, lysins have been proposed as a highly promising alternative class of new antibacterials (Briers, Walmagh, Grymonprez, et al. Citation2014; Gutiérrez et al. Citation2014; Czaplewski et al. Citation2016; Defraine et al. Citation2016). Furthermore, these new antibacterials also show synergies with a currently used standard of care antibiotics, enabling the possibility of combination therapies (Channabasappa et al. Citation2018; Kim et al. Citation2018; Watson et al. Citation2019). First- and second-generation lysins may encounter specific hurdles or may perform suboptimally under clinical settings, giving room for further optimizations. These opportunities are increasingly explored by advanced engineering techniques, defined here as third-generation lysins, and formulation strategies. This potential for easy engineering sets lysins apart from all existing classes of small-molecule antibiotics.
This review discussed approaches to tackle clinical hurdles. Briefly, one can expand the activity of a lysin by fusion to cell-penetrating peptides to target intracellular pathogens, co-formulate the lysin with anti-inflammatory compounds, or with polymers having beneficial properties for topical applications. In addition, the half-life can be increased by reducing the risk of an immune response using so-called masking-strategies or by avoiding renal filtration increasing the hydrodynamic volume. Several of these engineering efforts resulted in reduced antibacterial activity in vitro, however, the added value became clear when tested in vivo. For example, by increasing their half-life by deimmunization, third-generation lysins performed worse in vitro but were shown to be more effective to treat recurrent infections in vivo (Zhao et al. Citation2015). Another example is the fusion with cell-penetrating peptides (CPPs). Here, the reduction in antibacterial activity was compensated by targeting intracellular S. aureus thereby eradicating a major cause of recurrent, chronic infections (Wang et al. Citation2018; Röhrig et al. Citation2020).
All efforts discussed in this review involved enzymes targeting Gram-positive pathogens. Indeed, it is even more challenging to come up with an efficient lysin-based approach to combat Gram-negative pathogens as the peptidoglycan layer is shielded by an outer membrane. Nonetheless, several second-generation lysins have (recently) shown to be promising against Gram-negative bacteria in vitro and ex vivo. A few engineered lysins have shown efficacy in C. elegans and mice (Briers, Walmagh, Van Puyenbroeck, et al. Citation2014; Heselpoth et al. Citation2019). Furthermore, two case studies with Art-175 in dogs have been reported (Briers and Lavigne Citation2015). Third-generation engineering approaches should be considered in the further development of these lysins targeting Gram-negative pathogens as well.
Generation of a lysin possessing superior properties might be facilitated using libraries with increased complexity combined with a screening approach under relevant conditions. This was illustrated by Röhrig and colleagues who performed a preceding screening of enzyme variants under intracellular and lysosomal conditions before different fusions with CPPs were attempted (Röhrig et al. Citation2020). Whereas the authors could rely on an available library, the VersaTile approach (Gerstmans et al. Citation2020) could further leverage this process by increasing library complexity by incorporating more CPPs, more CBDs, EADs, or other elements of interest. It is clear that many concepts that are highlighted in this review can benefit from such libraries of increased complexity, but following the first law of directed evolution (“you get what you screen for”), the screening assays must be carefully selected and/or combined given the observed discrepancies between different in vitro and in vivo assays (Schmidt-Dannert and Arnold Citation1999; Peisajovich and Tawfik Citation2007).
Several third-generation engineering strategies or formulation strategies discussed in this review (, ) can be expanded to combat infections in other body sites. As mentioned before, a limited amount of third-generation approaches or formulation strategies have been reported for some mucosal applications. As an example, one might also consider the use of nanoparticles to increase the release time of lysin to fight urinary tract, vaginal, or other mucosa-related infections. Indeed, both liposomal and niosome nanoparticle formulation strategies with lysins may have the potential to combat these types of infections (Bai et al. Citation2019; Sadeghi et al. Citation2019; Portilla et al. Citation2020). In general, literature describing the treatment of these infections with lysins is rather limited notwithstanding there is a high societal need for alternatives to the current small-molecule antibiotics (Cheng et al. Citation2005; Li et al. Citation2018).
As lysins are moving closer towards actual introduction in the clinic, limited protein yield in standard industrial expression systems might represent an additional hurdle for translation to practice. Therefore, some suggested third-generation approaches might be less favourable. As an example, one could opt to glycosylate the therapeutic protein to reduce proteolysis in vivo (Doering et al. Citation2017). However, eukaryotic expression systems are cost-intensive, and apart from yeast-based expression systems less scalable.
Two decades of research of lysins as antibacterials have resulted in substantial progress, with a growing global community of researchers from an increasing number of disciplines involved. It is expected that interdisciplinary approaches will further leverage lysins as a class of novel antibiotics. In addition, lysins can be easily modified and fused with function enhancing domains due to their proteinaceous and modular nature, giving rise to a distinct engineering potential.
Acknowledgements
The authors thank Dominique Holtappels for sharing his expertise in mining the patent landscape.
Disclosure statement
RL and YB are co-inventors on a patent application related to VersaTile (WO2018114980).
Additional information
Funding
References
- Abdullahi A, Amini-Nik S, Jeschke MG. 2014. Animal models in burn research. Cell Mol Life Sci. 71(17):3241–3255.
- Abouhmad A, Dishisha T, Amin MA, Hatti-Kaul R. 2017. Immobilization to positively charged cellulose nanocrystals enhances the antibacterial activity and stability of hen egg white and T4 lysozyme. Biomacromolecules. 18(5):1600–1608.
- Abouhmad A, Mamo G, Dishisha T, Amin MA, Hatti-Kaul R. 2016. T4 lysozyme fused with cellulose-binding module for antimicrobial cellulosic wound dressing materials. J Appl Microbiol. 121(1):115–125.
- Agren MS, Eaglstein WH, Ferguson MW, Harding KG, Moore K, Saarialho-Kere UK, Schultz G. 2000. Causes and effects of the chronic inflammation in venous leg ulcers. Acta Dermato-Venereologica. 80(210):3–17.
- Backes M, Spijkerman IJ, de Muinck-Keizer RJO, Goslings JC, Schepers T. 2018. Determination of pathogens in postoperative wound infection after surgically reduced calcaneal fractures and implications for prophylaxis and treatment. J Foot Ankle Surg. 57(1):100–103.
- Bai J, Yang E, Chang PS, Ryu S. 2019. Preparation and characterization of endolysin-containing liposomes and evaluation of their antimicrobial activities against Gram-negative bacteria. Enzyme Microb Technol. 128:40–48.
- Becker SC, Dong S, Baker JR, Foster-Frey J, Pritchard DG, Donovan DM. 2009. LysK CHAP endopeptidase domain is required for lysis of live staphylococcal cells. FEMS Microbiol Lett. 294(1):52–60.
- Becker SC, Roach DR, Chauhan VS, Shen Y, Foster-Frey J, Powell AM, Bauchan G, Lease RA, Mohammadi H, Harty WJ, et al. 2016. Triple-acting lytic enzyme treatment of drug-resistant and intracellular Staphylococcus aureus. Sci Rep. 6:25010–25063.
- Beller FK, Schweppe KW. 1979. Review on the biology of menstrual blood. In Beller FK and Schumacher GGB, editors. The biology of the fluids of the female genital tract. New York (NY): Elsevier; p. 231–245.
- Blazanovic K, Zhao H, Choi Y, Li W, Salvat RS, Osipovitch DC, Fields J, Moise L, Berwin BL, Fiering SN. 2015. Structure-based redesign of lysostaphin yields potent antistaphylococcal enzymes that evade immune cell surveillance. Mol Ther - Meth Clin Dev. 2:1–10.
- Blum JS, Wearsch PA, Cresswell P. 2013. Pathways of antigen processing. Annu Rev Immunol. 31:443–473.
- Briers Y, Lavigne R. 2015. Breaking barriers: expansion of the use of endolysins as novel antibacterials against Gram-negative bacteria. Future Microbiol. 10(3):377–390.
- Briers Y, Walmagh M, Grymonprez B, Biebl M, Pirnay J-P, Defraine V, Michiels J, Cenens W, Aertsen A, Miller S, et al. 2014. Art-175 is a highly efficient antibacterial against multidrug-resistant strains and persisters of Pseudomonas aeruginosa. Antimicrob Agents Chemother. 58(7):3774–3784.
- Briers Y, Walmagh M, Van Puyenbroeck V, Cornelissen A, Cenens W, Aertsen A, Oliveira H, Azeredo J, Verween G, Pirnay J-P, et al. 2014. Engineered endolysin-based “Artilysins” to combat multidrug resistant Gram-negative pathogens. mBio. 5(4):1–10.
- Busscher HJ, van der Mei HC, Subbiahdoss G, Jutte PC, van den Dungen JJAM, Zaat SAJ, Schultz MJ, Grainger DW. 2012. Biomaterial-associated infection: locating the finish line in the race for the surface. Sci Transl Med. 4(153):153rv10–153rv10.
- Byun S, Park J, Lee E, Lim S, Yu JG, Lee SJ, Chen H, Dong Z, Lee KW, Lee HJ. 2013. Src kinase is a direct target of apigenin against UVB-induced skin inflammation. Carcinogenesis. 34(2):397–405.
- Cassini A, Högberg LD, Plachouras D, Quattrocchi A, Hoxha A, Simonsen GS, Colomb-Cotinat M, Kretzschmar ME, Devleesschauwer B, Cecchini M, et al. 2019. Attributable deaths and disability-adjusted life-years caused by infections with antibiotic-resistant bacteria in the EU and the European Economic Area in 2015: a population-level modelling analysis. The Lancet Infectious Diseases. 19(1):56–66.
- Centers for Disease Prevention and Control. 2013. Antibiotic resistance threats in the United States, 2013. Current. 114. https://www.cdc.gov/drugresistance/biggest-threats.html.
- Centers for Disease Prevention and Control. 2019. Antibiotic resistance threats in the United States, 2019. Atlanta (GA): U.S. Department of Health and Human Services, CDC. https://www.cdc.gov/drugresistance/biggest-threats.html.
- Ceaglio N, Etcheverrigaray M, Conradt HS, Grammel N, Kratje R, Oggero M. 2010. Highly glycosylated human alpha interferon: an insight into a new therapeutic candidate. J Biotechnol. 146(1-2):74–83.
- Chang C-J, Chen C-H, Chen B-M, Su Y-C, Chen Y-T, Hershfield MS, Lee M-TM, Cheng T-L, Chen Y-T, Roffler SR, et al. 2017. A genome-wide association study identifies a novel susceptibility locus for the immunogenicity of polyethylene glycol. Nat Commun. 8(1):1–8.
- Channabasappa S, Chikkamadaiah R, Durgaiah M, Kumar S, Ramesh K, Sreekanthan A, Sriram B. 2018. Efficacy of chimeric ectolysin P128 in drug-resistant Staphylococcus aureus bacteraemia in mice. J Antimicrob Chemother. 73(12):3398–3404.
- Chaudhury C, Mehnaz S, Robinson JM, Hayton WL, Pearl DK, Roopenian DC, Anderson CL. 2003. The major histocompatibility complex-related Fc receptor for IgG (FcRn) binds albumin and prolongs its lifespan. J Exp Med. 197(3):315–322.
- Cheng M, Zhang L, Zhang H, Li X, Wang Y, Xia F, Wang B, Cai R, Guo Z, Zhang Y, et al. 2018. An ointment consisting of the phage lysin LysGH15 and apigenin for decolonization of methicillin-resistant Staphylococcus aureus from skin wounds. Viruses. 10(5):215–244.
- Cheng M, Zhang Y, Li X, Liang J, Hu L, Gong P, Zhang L, Cai R, Zhang H, Ge J, et al. 2017. Endolysin LysEF-P10 shows potential as an alternative treatment strategy for multidrug-resistant Enterococcus faecalis infections. Sci Rep. 7(1):1–15.
- Cheng Q, Nelson D, Zhu S, Fischetti VA. 2005. Removal of group B streptococci colonizing the vagina and oropharynx of mice with a bacteriophage lytic enzyme. Antimicrob Agents Chemother. 49(1):111–117.
- Clausen H, Wandall HH, Steentoft C. 2017. Glycosylation engineering. In: Varki A, Cummings RD, Esko JD, editors. Essentials of glycobiology. 3rd ed. Cold Spring Harbor (NY): Cold Spring Harbor Laboratory Press; p. 2015–2017.
- Czaplewski L, Bax R, Clokie M, Dawson M, Fairhead H, Fischetti VA, Foster S, Gilmore BF, Hancock REW, Harper D, et al. 2016. Alternatives to antibiotics-a pipeline portfolio review. Lancet Infect Dis. 16(2):239–251.
- De Smet J, Hendrix H, Blasdel BG, Danis-Wlodarczyk K, Lavigne R. 2017. Pseudomonas predators: understanding and exploiting phage-host interactions. Nat Rev Microbiol. 15(9):517–530.
- Defraine V, Schuermans J, Grymonprez B, Govers SK, Aertsen A, Fauvart M, Michiels J, Lavigne R, Briers Y. 2016. Efficacy of artilysin Art-175 against resistant and persistent Acinetobacter baumannii. Antimicrob Agents Chemother. 60(6):3480–3488.
- Diederen BMW, Wardle CLW, Krijnen P, Tuinebreijer WE, Breederveld RS. 2015. Epidemiology of clinically relevant bacterial pathogens in a burn center in the Netherlands between 2005 and 2011. J Burn Care Res. 36(3):446–453.
- Doehn JM, Fischer K, Reppe K, Gutbier B, Tschernig T, Hocke AC, Fischetti VA, Löffler J, Suttorp N, Hippenstiel S, et al. 2013. Delivery of the endolysin Cpl-1 by inhalation rescues mice with fatal pneumococcal pneumonia. J Antimicrob Chemother. 68(9):2111–2117.
- Doering TL, Cummings RD, Aebi M. 2017. Fungi. In: Varki A, Cummings RD, Esko JD, editors. Essentials of glycobiology. 3rd ed. Cold Spring Harbor (NY): Cold Spring Harbor Laboratory Press; p. 2015–2017.
- Duthie G, Crozier A. 2000. Plant-derived phenolic antioxidants. Curr Opin Clin Nutr Metab Care. 3(6):447–451.
- El-Manzalawy Y, Dobbs D, Honavar V. 2011. Predicting MHC-II binding affinity using multiple instance regression. IEEE/ACM Trans Comput Biol Bioinform. 8(4):1067–1079.
- Fierheller M, Sibbald RG. 2010. A clinical investigation into the relationship between increased periwound skin temperature and local wound infection in patients with chronic leg ulcers. Adv Skin Wound Care. 23(8):369–379.
- Fleming AP. 1945. Nobel lecture. Available from https://www.nobelprize.org/prizes/medicine/1945/fleming/lecture/
- Gallo RL, Hooper LV. 2012. Epithelial antimicrobial defence of the skin and intestine. Nat Rev Immunol. 12(7):503–516.
- Gerstmans H, Criel B, Briers Y. 2018. Synthetic biology of modular endolysins. Biotechnol Adv. 36(3):624–640.
- Gerstmans H, Grimon D, Gutiérrez D, Lood C, Rodríguez A, van Noort V, Lammertyn J, Lavigne R, Briers Y. 2020. A VersaTile-driven platform for rapid hit-to-lead development of engineered lysins. Sci Adv. 6(23):eaaz1136.
- Gervasi T, Horn N, Wegmann U, Dugo G, Narbad A, Mayer MJ. 2014. Expression and delivery of an endolysin to combat Clostridium perfringens. Appl Microbiol Biotechnol. 98(6):2495–2505.
- Gilmer DB, Schmitz JE, Euler CW, Fischetti VA. 2013. Novel bacteriophage lysin with broad lytic activity protects against mixed infection by Streptococcus pyogenes and methicillin-resistant Staphylococcus aureus. Antimicrob Agents Chemother. 57(6):2743–2750.
- Gokarn YR, McLean M, Laue TM. 2012. Effect of PEGylation on protein hydrodynamics. Mol Pharm. 9(4):762–773.
- Gondil VS, Dube T, Panda JJ, Yennamalli RM, Harjai K, Chhibber S. 2020. Comprehensive evaluation of chitosan nanoparticle based phage lysin delivery system; a novel approach to counter S. pneumoniae infections. Int J Pharm. 573:118850.
- Graf F. 2019 November 07. Probiotic bacterial strains producing antimicrobial proteins and compositions comprising these for use in the treatment of diarrheal and other microbial diseases. WO2019211382A1.
- Grishin AV, Lavrova NV, Lyashchuk AM, Strukova NV, Generalova MS, Ryazanova AV, Shestak NV, Boksha IS, Polyakov NB, Galushkina ZM, et al. 2019. The influence of dimerization on the pharmacokinetics and activity of an antibacterial enzyme lysostaphin. Molecules. 24(10):1813–1879.
- Grishin AV, Shestak NV, Lavrova NV, Lyashchuk AM, Popova LI, Strukova NV, Generalova MS, Ryazanova AV, Polyakov NB, Galushkina ZM, et al. 2019. Fusion of lysostaphin to an albumin binding domain prolongs its half-life and bactericidal activity in the systemic circulation. Molecules. 24(16):2814–2892.
- Gutiérrez D, Ruas-Madiedo P, Martínez B, Rodríguez A, García P. 2014. Effective removal of staphylococcal biofilms by the endolysin LysH5. PLoS One. 9(9):e107307.
- Habibi Y, Lucia LA, Rojas OJ. 2010. Cellulose nanocrystals: chemistry, self-assembly, and applications. Chem Rev. 110(6):3479–3500.
- Hajiahmadi F, Alikhani MY, Shariatifar H, Arabestani MR, Ahmadvand D. 2019. The bactericidal effect of lysostaphin coupled with liposomal vancomycin as a dual combating system applied directly on methicillin-resistant Staphylococcus aureus infected skin wounds in mice. Int J Nanomed. 14:5943–5955.
- Hang H, Rangan KJ, Mucida D, Pedicord V, inventors. 2016 October 27. Modified microorganisms expressing SagA as anti-infective agents, probiotics and food components. WO2016172476A1.
- Harhala M, Nelson D, Miernikiewicz P, Heselpoth R, Brzezicka B, Majewska J, Linden S, Shang X, Szymczak A, Lecion D, et al. 2018. Safety studies of pneumococcal endolysins Cpl-1 and Pal. Viruses. 10(11):638.
- Hathaway H, Ajuebor J, Stephens L, Coffey A, Potter U, Sutton JM, Jenkins ATA. 2017. Thermally triggered release of the bacteriophage endolysin CHAPK and the bacteriocin lysostaphin for the control of methicillin resistant Staphylococcus aureus (MRSA). J Control Release. 245:108–115.
- Heath WR, Carbone FR. 2013. The skin-resident and migratory immune system in steady state and memory: innate lymphocytes, dendritic cells and T cells. Nat Immunol. 14(10):978–985.
- Hersh AL, Chambers HF, Maselli JH, Gonzales R. 2008. National trends in ambulatory visits and antibiotic prescribing for skin and soft-tissue infections. Arch Intern Med. 168(14):1585–1591.
- Heselpoth RD, Euler CW, Schuch R, Fischetti VA. 2019. Lysocins: bioengineered antimicrobials that deliver lysins across the outer membrane of Gram-negative bacteria. Antimicrob Agents Chemother. 63(6):1–14.
- Héver H, Darula Z, Medzihradszky KF. 2019. Characterisation of site-specific N-glycosylation. In: Kannicht C, editor. Post-translational modification of proteins. New York (NY): Humana Press; p. 93–125.
- Huang CY, Hsu JT, Chung PH, Cheng WTK, Jiang YN, Ju YT. 2013. Site-specific N-glycosylation of caprine lysostaphin restricts its bacteriolytic activity toward Staphylococcus aureus. Anim Biotechnol. 24(2):129–147.
- Huang L, Luo D, Gondil VS, Gong Y, Jia M, Yan D, He J, Hu S, Yang H, Wei H, et al. 2020. Construction and characterization of a chimeric lysin ClyV with improved bactericidal activity against Streptococcus agalactiae in vitro and in vivo. Appl Microbiol Biotechnol. 104(4):1609–1619.
- Jabbour JF, Sharara SL, Kanj SS. 2020. Treatment of multidrug-resistant Gram-negative skin and soft tissue infections. Curr Opin Infect Dis. 33(2):146–154.
- Jackson JK, Letchford K, Wasserman BZ, Ye L, Hamad WY, Burt HM. 2011. The use of nanocrystalline cellulose for the binding and controlled release of drugs. Int J Nanomed. 6:321–330.
- Jado I, López R, García E, Fenoll A, Casal J, García P. 2003. Phage lytic enzymes as therapy for antibiotic-resistant Streptococcus pneumoniae infection in a murine sepsis model. J Antimicrob Chemother. 52(6):967–973.
- Johnson CT, Wroe JA, Agarwal R, Martin KE, Guldberg RE, Donlan RM, Westblade LF, García AJ. 2018. Hydrogel delivery of lysostaphin eliminates orthopedic implant infection by Staphylococcus aureus and supports fracture healing. Proc Natl Acad Sci USA. 115(22):E4960.
- Jonsson A, Dogan J, Herne N, Abrahmsén L, Nygren PÅ. 2008. Engineering of a femtomolar affinity binding protein to human serum albumin. Protein Eng Des Sel. 21(8):515–527.
- Jun SY, Jang IJ, Yoon S, Jang K, Yu K-S, Cho JY, Seong M-W, Jung GM, Yoon SJ, Kang SH. 2017. Pharmacokinetics and Tolerance of the Phage endolysin-based candidate drug SAL200 after a single intravenous administration among healthy volunteers. Antimicrob Agents Chemother. 61(6):1–11.
- Jun SY, Jung GM, Yoon SJ, Youm SY, Han H-Y, Lee J-H, Kang SH. 2016. Pharmacokinetics of the phage endolysin-based candidate drug SAL200 in monkeys and its appropriate intravenous dosing period. Clin Exp Pharmacol Physiol. 43(10):1013–1016.
- Karen CC, John GB. 2011. Biology of Clostridium difficile: implications for epidemiology and diagnosis. Annu Rev Microbiol. 65(1):501–521.
- Kim N-H, Park WB, Cho JE, Choi YJ, Choi SJ, Jun SY, Kang CK, Song K-H, Choe PG, Bang J-H, et al. 2018. Effects of phage endolysin SAL200 combined with antibiotics on Staphylococcus aureus infection. Antimicrob Agents Chemother. 62(10):e00731.
- Kim S, Lee D-W, Jin J-S, Kim J. 2020. Antimicrobial activity of LysSS, a novel phage endolysin, against Acinetobacter baumannii and Pseudomonas aeruginosa. J Global Antimicrobial Resist. 22:39.
- Kiss Z, Elliott S, Jedynasty K, Tesar V, Szegedi J. 2010. Discovery and basic pharmacology of erythropoiesis-stimulating agents (ESAs), including the hyperglycosylated ESA, darbepoetin alfa: an update of the rationale and clinical impact . Eur J Clin Pharmacol. 66(4):331–340.
- Kontermann RE. 2011. Strategies for extended serum half-life of protein therapeutics. Curr Opin Biotechnol. 22(6):868–876.
- Lai M-J, Lin N-T, Hu A, Soo P-C, Chen L-K, Chen L-H, Chang K-C. 2011. Antibacterial activity of Acinetobacter baumannii phage ΦaB2 endolysin (LysAB2) against both Gram-positive and Gram-negative bacteria. Appl Microbiol Biotechnol. 90(2):529–539.
- Leimer N, Rachmühl C, Palheiros Marques M, Bahlmann AS, Furrer A, Eichenseher F, Seidl K, Matt U, Loessner MJ, Schuepbach RA, et al. 2016. Nonstable Staphylococcus aureus small-colony variants are induced by low pH and sensitized to antimicrobial therapy by phagolysosomal alkalinization. J Infect Dis. 213(2):305–313.
- Lewis K. 2013. Platforms for antibiotic discovery. Nat Rev Drug Discov. 12(5):371–387.
- Li KC, Ho YL, Hsieh WT, Huang SS, Chang YS, Huang GJ. 2015. Apigenin-7-glycoside prevents LPS-induced acute lung injury via downregulation of oxidative enzyme expression and protein activation through inhibition of MAPK phosphorylation. Int J Mol Sci. 16(1):1736–1754.
- Li W, Yang H, Gong Y, Wang S, Li Y, Wei H. 2018. Effects of a chimeric lysin against planktonic and sessile Enterococcus faecalis hint at potential application in endodontic therapy. Viruses. 10(6):213–290.
- Lindström M, Heikinheimo A, Lahti P, Korkeala H. 2011. Novel insights into the epidemiology of Clostridium perfringens type A food poisoning. Food Microbiol. 28(2):192–198.
- Liu H, Gao Y, Yu LR, Jones RC, Elkins CA, Hart ME. 2011. Inhibition of Staphylococcus aureus by lysostaphin-expressing Lactobacillus plantarum WCFS1 in a modified genital tract secretion medium. Appl Environ Microbiol. 77(24):8500–8508.
- Loeffler JM, Djurkovic S, Fischetti VA. 2003. Phage lytic enzyme Cpl-1 as a novel antimicrobial for pneumococcal bacteremia. Infect Immunol. 71(11):6199–6204.
- Lopez-Jornet P, Camacho-Alonso F, Gómez-Garcia F, Molina Miñano F, Cañas X, Serafín A, Castillo J, Vicente-Ortega V. 2014. Effects of potassium apigenin and verbena extract on the wound healing process of SKH-1 mouse skin. Int Wound J. 11(5):489–495.
- Lukacik P, Barnard TJ, Keller PW, Chaturvedi KS, Seddiki N, Fairman JW, Noinaj N, Kirby TL, Henderson JP, Steven AC, et al. 2012. Structural engineering of a phage lysin that targets Gram-negative pathogens. Proc Natl Acad Sci USA. 109(25):9857–9862.
- Mandell LA. 2015. Community-acquired pneumonia: an overview. Postgrad Med. 127(6):607–615.
- Mccormick JK, Yarwood JM, Schlievert PM. 2001. Toxic shock syndrome and bacterial superantigens: an update. Annu Rev Microbiol. 55:77–104.
- Miao J, Pangule RC, Paskaleva EE, Hwang EE, Kane RS, Linhardt RJ, Dordick JS. 2011. Lysostaphin-functionalized cellulose fibers with antistaphylococcal activity for wound healing applications. Biomaterials. 32(36):9557–9567.
- Miller LG, Eisenberg DF, Liu H, Chang C-L, Wang Y, Luthra R, Wallace A, Fang C, Singer J, Suaya JA. 2015. Incidence of skin and soft tissue infections in ambulatory and inpatient settings, 2005-2010. BMC Infect Dis. 15(1):1–8.
- Moak M, Molineux IJ. 2004. Peptidoglycan hydrolytic activities associated with bacteriophage virions. Mol Microbiol. 51(4):1169–1183.
- Morita M, Tanji Y, Mizoguchi K, Soejima A, Orito Y, Unno H. 2001. Antibacterial activity of Bacillus amyloliquefaciens phage endolysin without holin conjugation. J Biosci Bioeng. 91(5):469–473.
- Nelson D, Loomis L, Fischetti VA. 2001. Prevention and elimination of upper respiratory colonization of mice by group A streptococci by using a bacteriophage lytic enzyme. PNAS. 98(7):4107–4112.
- Nilebäck L, Widhe M, Seijsing J, Bysell H, Sharma PK, Hedhammar M. 2019. Bioactive silk coatings reduce the adhesion of Staphylococcus aureus while supporting growth of osteoblast-like cells. ACS Appl Mater Interfaces. 11(28):24999–25007.
- Nithya S, Nimal TR, Baranwal G, Suresh MK, Anju CP, Anil Kumar V, Gopi Mohan C, Jayakumar R, Biswas R. 2018. Preparation, characterization and efficacy of lysostaphin-chitosan gel against Staphylococcus aureus. Int J Biol Macromol. 110:157–166.
- Nour El-Din HT, Elhosseiny NM, El-Gendy MA, Mahmoud AA, Hussein MMM, Attia AS. 2020. A rapid lysostaphin production approach and a convenient novel lysostaphin loaded nano-emulgel as a sustainable low-cost methicillin-resistant Staphylococcus aureus combating platform. Biomolecules. 10(3):415–435.
- O’Neill J. 2016. Tackling drug-resistant infections globally: final report and recommendations. The Review on Antimicrobial Resistance. London (UK): HM Government.
- Orito Y, Morita M, Hori K, Unno H, Tanji Y. 2004. Bacillus amyloliquefaciens phage endolysin can enhance permeability of Pseudomonas aeruginosa outer membrane and induce cell lysis. Appl Microbiol Biotechnol. 65(1):105–109.
- Otto M. 2009. Staphylococcus epidermidis-the ‘accidental’ pathogen. Nat Rev Microbiol. 7(8):555–567.
- Park HS, Pham C, Paul E, Padiglione A, Lo C, Cleland H. 2017. Early pathogenic colonisers of acute burn wounds: a retrospective review. Burns. 43(8):1757–1765.
- Parker AS, Choi Y, Griswold KE, Bailey-Kellogg C. 2013. Structure-guided deimmunization of therapeutic proteins. J Comput Biol. 20(2):152–165.
- Pastagia M, Euler C, Chahales P, Fuentes-Duculan J, Krueger JG, Fischetti VA. 2011. A novel chimeric lysin shows superiority to mupirocin for skin decolonization of methicillin-resistant and -sensitive Staphylococcus aureus strains. Antimicrob Agents Chemother. 55(2):738–744.
- Paul S, Lindestam Arlehamn CS, Scriba TJ, Dillon MBC, Oseroff C, Hinz D, McKinney DM, Carrasco Pro S, Sidney J, Peters B, et al. 2015. Development and validation of a broad scheme for prediction of HLA class II restricted T cell epitopes. J Immunol Methods. 422:28–34.
- Pedrolli DB, Ribeiro NV, Squizato PN, de Jesus VN, Cozetto DA. 2019. Engineering microbial living therapeutics: the synthetic biology toolbox. Trends Biotechnol. 37(1):100–115.
- Peisajovich SG, Tawfik DS. 2007. Protein engineers turned evolutionists. Nat Methods. 4(12):991–994.
- Peyrusson F, Varet H, Nguyen TK, Legendre R, Sismeiro O, Coppée J-Y, Wolz C, Tenson T, Van Bambeke F. 2020. Intracellular Staphylococcus aureus persisters upon antibiotic exposure. Nat Commun. 11(1):2200–2214.
- Portilla S, Fernández L, Gutiérrez D, Rodríguez A, García P. 2020. Encapsulation of the antistaphylococcal endolysin Lysrodi in pH-sensitive liposomes. Antibiotics. 9(5):242–248.
- Rangan KJ, Pedicord VA, Wang Y-C, Kim B, Lu Y, Shaham S, Mucida D, Hang HC. 2016. A secreted bacterial peptidoglycan hydrolase enhances tolerance to enteric pathogens. Science. 353(6306):1434–1437.
- Rashel M, Uchiyama J, Ujihara T, Uehara Y, Kuramoto S, Sugihara S, Yagyu K-I, Muraoka A, Sugai M, Hiramatsu K, et al. 2007. Efficient elimination of multidrug-resistant Staphylococcus aureus by cloned lysin derived from bacteriophage phi MR11. J Infect Dis. 196(8):1237–1247.
- Resch G, Moreillon P, Fischetti VA. 2011a. A stable phage lysin (Cpl-1) dimer with increased antipneumococcal activity and decreased plasma clearance. Int J Antimicrob Agents. 38(6):516–521.
- Resch G, Moreillon P, Fischetti VA. 2011b. PEGylating a bacteriophage endolysin inhibits its bactericidal activity. AMB Express. 1(29):1–5. DOI:10.1186/2191-0855-1-29.
- Röhrig C, Huemer M, Lorgé D, Luterbacher S, Phothaworn P, Schefer C, Sobieraj AM, Zinsli LV, Mairpady Shambat S, Leimer N, et al. 2020. Targeting hidden pathogens: cell-penetrating enzybiotics eradicate intracellular drug-resistant Staphyloccus aureus. mBio. 11(2):1–19.
- Sadeghi S, Bakhshandeh H, Cohan RA, Peirovi A, Ehsani P, Norouzian D. 2019. Synergistic anti-staphylococcal activity of niosomal recombinant lysostaphin-LL-37. Int J Nanomed. 14:9777–9792.
- Schmidt-Dannert C, Arnold FH. 1999. Directed evolution of industrial enzymes. Trends Biotechnol. 17(4):135–136.
- Schuch R, Indiani C, Wittekind M, inventor. 2019 August 29. Modified plySs2 lysins and uses thereof. WO2019165454A1.
- Schuch R, Lee HM, Schneider BC, Sauve KL, Law C, Khan BK, Rotolo JA, Horiuchi Y, Couto DE, Raz A, et al. 2014. Combination therapy with lysin CF-301 and antibiotic is superior to antibiotic alone for treating methicillin-resistant Staphylococcus aureus-induced murine bacteremia. J Infectious Dis. 209(9):1469–1478.
- Seijsing F, Nilebäck L, Öhman O, Pasupuleti R, Ståhl C, Seijsing J, Hedhammar M. 2020. Recombinant spider silk coatings functionalized with enzymes targeting bacteria and biofilms. MicrobiologyOpen. 9(4):1–10.
- Seijsing J, Sobieraj AM, Keller N, Shen Y, Zinkernagel AS, Loessner MJ, Schmelcher M. 2018. Improved biodistribution and extended serum half-life of a bacteriophage endolysin by albumin binding domain fusion. Front Microbiol. 9:2927–2929.
- Shah A, Mond J, Walsh S. 2004. Lysostaphin-coated catheters eradicate Staphylococccus aureus challenge and block surface colonization. Antimicrob Agents Chemother. 48(7):2704–2707.
- Shahbazian JH, Hahn PD, Ludwig S, Ferguson J, Baron P, Christ A, Spicer K, Tolomeo P, Torrie AM, Bilker WB, et al. 2017. Multidrug and mupirocin resistance in environmental methicillin-resistant Staphylococcus aureus (MRSA) isolates from homes of people diagnosed with community-onset MRSA infection. Appl Environ Microbiol. 83(22):1–14.
- Shen Y, Barros M, Vennemann T, Gallagher DT, Yin Y, Linden SB, Heselpoth RD, Spencer DJ, Donovan DM, Moult J, et al. 2016. A bacteriophage endolysin that eliminates intracellular streptococci. eLife. 5:1–26.
- Shoseyov O, Shani Z, Levy I. 2006. Carbohydrate binding modules: biochemical properties and novel applications. Microbiol Mol Biol Rev. 70(2):283–295.
- Solá RJ, Griebenow K. 2010. Glycosylation of therapeutic proteins: an effective strategy to optimize efficacy. BioDrugs. 24(1):9–21.
- Soong G, Paulino F, Wachtel S, Parker D, Wickersham M, Zhang D, Brown A, Lauren C, Dowd M, West E, et al. 2015. Methicillin-resistant Staphylococcus aureus adaptation to human keratinocytes. mBio. 6(2):1–14.
- Szweda P, Gorczyca G, Tylingo R, Kurlenda J, Kwiecinski J, Milewski S. 2014. Chitosan-protein scaffolds loaded with lysostaphin as potential antistaphylococcal wound dressing materials. J Appl Microbiol. 117(3):634–642.
- Tayeb HH, Sainsbury F. 2018. Nanoemulsions in drug delivery: formulation to medical application. Nanomed. 13(19):2507–2525.
- Theuretzbacher U, Gottwalt S, Beyer P, Butler M, Czaplewski L, Lienhardt C, Moja L, Paul M, Paulin S, Rex JH, et al. 2019. Analysis of the clinical antibacterial and antituberculosis pipeline. The Lancet Infectious Diseases. 19(2):e40–e50.
- Theuretzbacher U, Outterson K, Engel A, Karlén A. 2020. The global preclinical antibacterial pipeline. Nat Rev Microbiol. 18(5):275–285.
- Turecek PL, Bossard MJ, Schoetens F, Ivens IA. 2016. PEGylation of biopharmaceuticals: a review of chemistry and nonclinical safety information of approved drugs. J Pharm Sci. 105(2):460–475.
- Verbree CT, Dätwyler SM, Meile S, Eichenseher F, Donovan DM, Loessner MJ, Schmelcher M. 2017. Identification of peptidoglycan hydrolase constructs with synergistic staphylolytic activity in cow’s milk. Appl Environ Microbiol. 84(1):1–15.
- Vladimirovna BN, Olegovna KA, Dimitrievich R, Vasilevna M, Viktorovich R, Vladimirovna SA, inventors. 2020 March 02. Pharmaceutical composition for treating eye infections caused by methicillin-resistant strains of Staphylococcus aureus, which includes as active ingredient N-terminal CHAP-domain of endolysin of bacteriophage K Staphylococcus aureus. RU2715694C1.
- Walsh S, Shah A, Mond J. 2003. Improved pharmacokinetics and reduced antibody reactivity of lysostaphin conjugated to polyethylene glycol. Antimicrob Agents Chemother. 47(2):554–558.
- Wang ZF, Kong L, Liu Y, Fu Q, Cui ZL, Wang J, Ma J, Wang H, Yan Y, Sun JH. 2018. A phage lysin fused to a cell-penetrating peptide kills intracellular methicillin-resistant Staphylococcus aureus in keratinocytes and has potential as a treatment for skin infections in mice. Appl Environ Microbiol. 84(12):1–13.
- Watson A, Sauve K, Cassino C, Schuch R. 2019. Exebacase demonstrates in vitro synergy with a broad range of antibiotics against both methicillin-resistant and methicillin-susceptible Staphylococcus aureus. Antimicrob Agents Chemother. 64(2):e01885.
- WHO. 2015. Global action plan on antimicrobial resistance. Geneva (Switzerland): WHO Press.
- WHO. 2017. Prioritization of pathogens to guide discorvery, research and development of new antibiotics for drug-resistant bacterial infections, including tuberculosis. Geneva (Switzerland): World Health Organization.
- Windolf CD, Lögters T, Scholz M, Windolf J, Flohé S, Schuch R. 2014. Lysostaphin-coated titan-implants preventing localized osteitis by Staphylococcus aureus in a mouse model. PLoS One. 9(12):e115940.
- Wong VW, Sorkin M, Glotzbach JP, Longaker MT, Gurtner GC. 2011. Surgical approaches to create murine models of human wound healing. J Biomed Biotechnol. 2011:969618–969618.
- Wu M, Hu K, Xie Y, Liu Y, Mu D, Guo H, Zhang Z, Zhang Y, Chang D, Shi Y. 2019. A novel phage PD-6A3, and its endolysin Ply6A3, with extended lytic activity against Acinetobacter baumannii. Front Microbiol. 9:1–12.
- Yang H, Zhang H, Wang J, Yu J, Wei H. 2017. A novel chimeric lysin with robust antibacterial activity against planktonic and biofilm methicillin-resistant Staphylococcus aureus. Sci Rep. 7:40182–40113.
- Yeroslavsky G, Girshevitz O, Foster-Frey J, Donovan DM, Rahimipour S. 2015. Antibacterial and antibiofilm surfaces through polydopamine-assisted immobilization of lysostaphin as an antibacterial enzyme. Langmuir. 31(3):1064–1073.
- Yudanova TN, Reshetov IV. 2006. Modern wound dressings: making and properties. II. Wound dressings containing immobilized proteolytic enzymes (a review). Pharm Chem J. 40(8):430–434.
- Zhang L, Li D, Li X, Hu L, Cheng M, Xia F, Gong P, Wang B, Ge J, Zhang H, et al. 2016. LysGH15 kills Staphylococcus aureus without being affected by the humoral immune response or inducing inflammation. Sci Rep. 6:29344–29349.
- Zhao H, Verma D, Li W, Choi Y, Ndong C, Fiering SN, Bailey-Kellogg C, Griswold KE. 2015. Depletion of T cell epitopes in lysostaphin mitigates anti-drug antibody response and enhances antibacterial efficacy in vivo. Chem Biol. 22(5):629–639.
- Zomer HD, Trentin AG. 2018. Skin wound healing in humans and mice: challenges in translational research. J Dermatol Sci. 90(1):3–12.