Abstract
The formation of nanostructures from a thin Au film deposited on a polystyrene and polymethylmethacrylate substrate under keV monatomic P, Ta and molecular PF4 ion irradiation is studied. The formation of nanoparticles on both kinds of polymers is shown. Heavy Ta ions are most effective in altering the Au layer, whereas its transformation by light P or molecular PF4 ions is less pronounced. Irradiation with PF4 ions has a much smaller effect on the PS and PMMA substrate bulk compared to that with monatomic P ions. The crucial role of collision cascade density in the process of thin metallic film coagulation on polymer substrate is revealed. During ion bombardment, transmitted IR light intensity increases proportionally to the area covered by the metal film, which makes it possible to use FTIR as a rapid technique to estimate the state of the nanoparticle formation process.
1. Introduction
Nanocomposite materials are of widespread use in various fields from catalysis to sensing and more. The incorporation of nanoparticles into a bulk matrix could increase the hardness, wear resistance and other mechanical properties of a composite material. One can also tailor electrical conductivity, optical transmission and reflection, antimicrobial properties, temperature response and many other characteristics. Noble metal nanoparticles exhibit tunable plasmon resonances over visible to near-infrared (VIS-NIR) spectral range. In particular, gold nanoparticles have attracted attention, primarily due to their unique electronic, optical, thermal, chemical and biological properties, and, accordingly, promising potential applications in various fields, including biology and medicine, chemistry, photonics, materials science and other interdisciplinary areas (Citation1–7).
One of the dry low-temperature techniques of gold nanoparticle synthesis is the heating of a few nanometer-thick gold layers (Citation4), or its irradiation with accelerated ions (Citation5). This layer can be either deposited on a substrate or buried ten to hundred nanometer deep. Moreover, metal ion implantation has been used to fabricate (Citation5) and control the size, shape and distribution (Citation7–9) of metal nanoclusters in various matrices. In particular, carbon-based polymer matrices are of interest due to the possible biocompatibility of a composite. Bombardment with small metallic clusters also can be successfully utilized to produce pinned or buried nanoparticles (Citation10, Citation11). Besides a lot of effort, there are still several unanswered questions. The influence of the substrate material on the process of particle formation can play a decisive role in the formation of nanoparticles. The local density of collision cascades formed by a stopping ion also affects radiation-related phenomena (Citation9). Overlapping of collision sub-cascades formed by atomic constituents of a molecular ion increases the density of the cumulative cascade formed by the molecular ion. This fact lets us study the effect of collision cascade density on the formation of gold nanoparticles. This work is devoted to studying changes in a thin gold layer deposited on a polymer film of two kinds: polymethylmethacrylate (PMMA) and polystyrene (PS) under accelerated molecular (PF4) and monatomic (P, Ta) ion bombardment.
2. Materials and methods
2.1. Sample fabrication
Monocrystalline (100) n-type silicon with a native oxide layer was used as the sample substrate. It was cleaned in hydrochloric acid, followed by thorough washing with distilled water and acetone. The polymer film was applied by the drop-casting technique. 0.0104 g of polymethyl methacrylate or 0.0121 g of polystyrene was dissolved in 3.75 g of dichloroethane at 24°C. After this, 1 drop of the solution was applied onto a 1 × 1 cm2 square Si substrate. The solution layer was leveled by centrifugation for 30 s at 500 rps. The samples were dried for 24 h in air at room temperature. The thickness of the resulting polymer films was about 70 nm, which was measured by atomic force microscopy over a scratch made by an iron needle. The gold layer was deposited by thermal evaporation at a pressure of 10−5 Torr using Oxford Instruments set-up. The substrate was kept at room temperature during Au deposition. The thickness of the deposited gold layer was ∼3 nm as measured by the 0.7 MeV He++ Rutherford backscattering on a test (without polymethyl methacrylate) piece of silicon (see (Citation9) for more detail).
2.2. Irradiation and characterization
Samples were irradiated at room temperature with P, Ta and PF4 ions using a 500 kV HVEE implanter. Ion irradiation parameters are presented in Table . The irradiation fluences, used to compare the effect of irradiation, were selected in a way to keep a constant number of displacements of gold atoms (displacements per atom) after bombardment with monatomic and molecular ions (Citation9). DPAAu values were calculated using TRIM software package (Citation12) simulation data as DPAAu = nv × D/nat, where nv is the average number of displacements produced by a single ion per unit length (cm−1); nat is the target atomic concentration (nat = 5.9·1022 cm−3 for gold layer); D is the incident ion fluence (cm−2). nv was determined by the TRIM code simulation with the default threshold atom displacement energies. Ion energy in all irradiations was kept at 0.65 keV/amu which gives 20, 70 and 120 keV for P, PF4 and Ta ions, respectively. The number of displacements generated by a molecular ion was calculated as a sum of displacements produced by one P and four F monatomic ions, as follows: DPAPF4 = DPAP + 4×DPAF.
Table 1. List of irradiation doses.
Most of the works related to ion-beam-induced formation of gold nanostructures by keV ion bombardment were carried out on crystal substrates (Citation6–9), or SiO2. The starting point for choosing the irradiation doses was the data from (Citation5), where the results of Ta and PF4 ion-beam – induced modification of a gold film on a silicon substrate are reported. The values of the irradiation doses used in this study and corresponding fluences are shown in Table .
After each irradiation, the surface topology was studied by atomic force microscopy (AFM) and scanning electron microscopy (SEM). The effect of irradiation on the internal polymer structure was studied using Fourier transform infrared spectroscopy (FTIR).
The structure of metal nanoparticles on the surface of the sample was studied using a Supra 40 electron microscope operating at 10 keV beam energy. The surface topography was studied using a Nano-DST machine (Pacific Nanotechnology). The AFM images were taken in semi-contact mode, which made it possible to minimize the effect of the probe on the sample surface. An NSG01 silicon tip by TipsNano with a radius of 10 nm with a stiffness coefficient of 1.45–15.1 N/m was used. The Gwyddion software package was used for AFM image processing. The Image-pro software package was used for SEM image processing. Measurements of the polymer structure were carried out in 400–4000 cm−1 spectral range using a VERTEX 80v device. FTIR spectra were processed using the OPUS software package.
3. Results and discussion
Figure shows SEM images of PMMA (Figure (a)) PS (Figure (e)) samples after gold layer deposition. Non-uniform polymer surface coverage with nanoisland formation could be seen in both these images. This is in contrast to the case of pure silicon, where a homogeneous Au layer is obtained at a given film thickness (Citation9). The variable shape of the gold islands is also visible. From the data obtained, it is not possible to identify any special pattern describing the shape of these islands. To characterize the structures formed, contour plots of bright features were drawn and surface coverage with gold was determined. As revealed by SEM, gold coverage over PS is slightly higher than that over PMMA. Nanoislands that appeared on PMMA on average have a larger size than those on PS.
Figure 1. SEM images of the surface of PMMA (A, B, C, D) and PS (E, F, G, H)-based samples (virgin (A and E) and irradiated to a dose of 0.054 DPA with P (B and F), PF4 (C and G), Ta (D and H) ions). The scale bar is 200 nm.
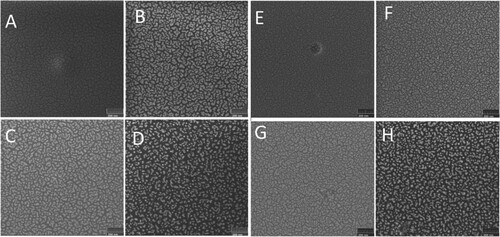
Ion irradiation alters the surface with some notable trends. Figure (b–d) presents SEM images of PMMA-based samples irradiated with P, Ta and PF4 ions, respectively to a dose of DPAAu = 0.054. Similarly, in Figure (f–h) SEM images of PS-based samples irradiated with P, Ta and PF4 ions to the same dose are shown. The gold layer rearranges under the beam as it follows from these figures. Indeed, inter-island distances increase, whereas islands themselves become smaller with the dose increase in both cases, i.e. the area occupied by gold decreases under ion irradiation. This effect is further illustrated by Figure , where atomic force microscopy images of the samples before and after irradiation with 120 keV Ta ions on both polymers are shown. Nanoparticle formation of the surface is clear in Figure (b,d), which is more pronounced on PMMA, exactly as shown in Figure (d,h). Initial roughness of ∼4 nm raises to ∼18 nm after irradiation. Indeed, structures seen in AFM images somehow differ from the structures in the images obtained by SEM (Figure (d,h)). This is because the AFM image shows the result of surface profile convolution with the geometric function of the tip. SEM contrast here is due to different secondary electron emissions from Au and polymer structures. Gold atoms could also diffuse into the polymer layer during deposition and ion irradiation which makes smaller Au features visible in SEM images. Indeed, it is also possible that the polymer degrades under ion irradiation and gases released from nanobubbles under the surface, which are observed in AFM images. Polymer degradation will be further discussed below.
Figure 2. AFM images of the surface of PMMA (A and B) and PS (C and D) samples (virgin (A and C) and irradiated Ta (B and C) up to DPA = 0.054).
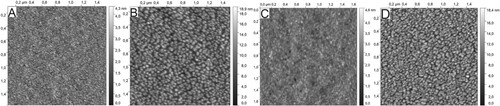
The effect of ion species is also seen in Figure . It follows from the images that the most separated nanostructures are obtained after Ta ion bombardment. Light P or molecular PF4 ions form almost the same features at this dose and both are less effective in altering the Au layer than Ta ions.
Transformation of the gold layer with the ion dose increase is demonstrated in Figure , where Au coated fraction of the surface area is shown. Independently of ion kind, the covered area shrinks, which supports the formation of nanoparticles found in AFM and SEM images. The dose rate of the Au layer transformation depends on the ion kind. Light P ions are less effective than molecular PF4 ions at low doses. On the other hand, with the dose increase, this difference vanishes and structures are found at 0.054 DPA after bombardment with both P and PF4 ions almost the same. Ta ions are significantly more effective in gold nanoparticle formation. The covered area on the PS substrate is higher than on PMMA, i.e. ion-beam-induced formation of nanoparticles on PMMA proceeds more effectively than on PS.
Figure 3. Surface area fraction covered with gold after ion irradiation as a function of dose. The inset shows an enlarged part of the same at low doses.
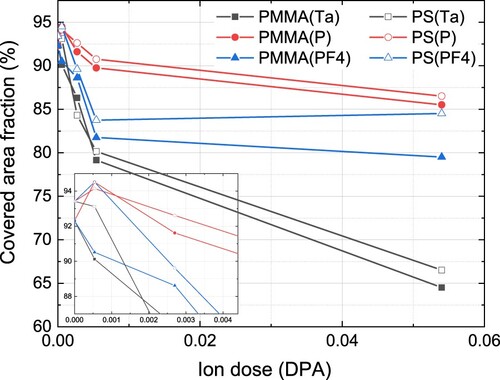
Interestingly, low-dose P and PF4 ion irradiation of the gold layer on the surface of PMMA smooths it increasing the covered area (see inset in Figure ). In the case of PS substrate, this effect is seen after P ion bombardment only. Heavy Ta ions start to coalesce layer to nanoparticles at the smallest doses used. A similar effect has been found in (Citation9) for Ta and PF4 ion bombardment of Au film on Si substrate. Thus, low-dose light ion irradiation could be used for controllable smoothing of thin metal layers deposited onto different substrates.
Ion bombardment would affect not only the gold layer but also the polymer substrate. To reveal the effect of irradiation on the structure of PS and PMMA, transmission spectra in the IR range were taken from the samples before and after irradiation. Figure presents typical FTIR spectra acquired before and after Ta ion bombardment. Several characteristic absorption peaks of the corresponding polymers are visible, which allows the identification of PMMA and PS. The spectra of samples irradiated with the highest doses used (DPAAu = 0.054) confirm that the polymer is not destroyed. The characteristic vibrations of the PMMA and PS group are still clearly visible (Figure (a)). The overall IR transparency of the sample increases with the dose increase. This effect is further illustrated in Figure (b), where the transmittance of the samples at 3500 cm−1 is plotted as a function of ion dose for all ions used. Comparing Figures and (b) one can see that the intensity of transmitted light correlates well with the surface area occupied by metal nanostructures. This area depends on the degree of modification of the metal layer (or on the dose the samples were irradiated with). Thus, due to the high speed of collecting Fourier spectra, this fact allows us to recommend FTIR as a useful method for rapid analysis of the stage of the process of synthesis of nanoparticles by ion bombardment.
Figure 4. (a) FTIR spectra of PMMA (solid line) and PS (dash line) based samples before and after irradiation with Ta ions to a dose of 0.054 DPA; (b) dependance of sample transmittance at 3500 cm−1 as a function of ion dose.
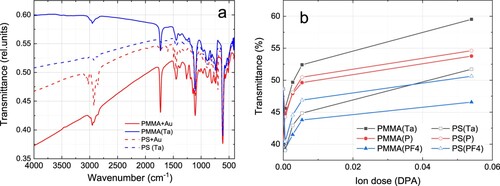
The effect of ion irradiation on polymer structure is illustrated in Figure , where intensities of some characteristic vibrational peaks of PMMA and PS are plotted as a function of ion dose. It is clear from the spectra that the intensity of peaks decreases. It can also be noted that PF4 ions at high doses affect the polymer to a much lesser extent in comparison with tantalum and phosphorus (Figure ). Thus, molecular ion irradiation is preferable in the case polymer structure needs to be preserved.
Figure 5. Transmission of specific peaks corresponding to chemical bond vibration in PMMA (a) and PS (b) as a function of ion dose.
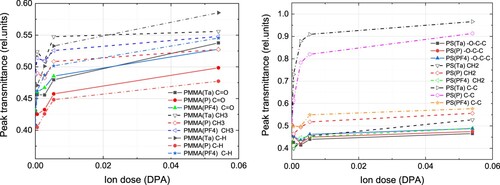
Note that irradiation with all species is done to the same dose in DPA, which means that each Au atom experienced the same displacements according to binary collision approximation. To explain the difference seen in Figures and , the role of individual cascade density should be taken into account. Indeed, ion stopping in the target form collision cascades, which shift atoms from their positions and develop instabilities in the thin gold layer thus providing conditions for particle growth. Heavy Ta ions form dense cascades where the nonlinearly high number of Au atoms could be displaced leading to the effective formation of well-separated particles. In the case of molecular PF4 ion imping the surface, atoms comprising the molecule split and each of them forms its collision cascade. At the same time, they all start to move in the target from almost the same point. Hence, at all individual collision cascades effectively overlap at the surface thus increasing the resulting density of a cumulative cascade. After they travel deep enough this overlap vanishes. From that point, the damage created by molecular ions would be lower than that created by monatomic P ions because the molecule consists of 4 F atoms, which are lighter than P atoms and form dilute collision cascades and produce lower ionization. This is seen in the FTIR spectra.
4. Conclusion
The transformation of thin Au film on polystyrene and polymethylmethacrylate substrate under irradiation with keV molecular PF4 and monatomic P, Ta ions is studied. Irradiation results in the formation of nanoparticles on both kinds of polymers. Heavy Ta ions are most effective in altering the Au layer, whereas its transformation by light P or molecular PF4 ions is less pronounced. Irradiation with PF4 ions has a much smaller effect on the PS and PMMA substrate bulk compared to monatomic P ions. Molecular PF4 ions are more effective in Au NP formation than monatomic P ions. Collision cascade density plays an important role in the process of thin metallic film coagulation on a polymer substrate. During ion bombardment, transmitted IR light intensity increases proportionally to the area covered by the metal film, which makes it possible to use FTIR as a rapid technique to estimate the state of the nanoparticle formation process.
Our study suggests that the ion beam production technique is highly attractive as a tool to produce metal–polymer nanocomposites via a thin film dewetting route. In addition, micro- and nano-structure fabrication using template-confined dewetting by sophisticated template engineering is promising because of its low cost, high throughput and high efficiency. Nanocomposites obtained could be used as substrates for surface-enhanced Raman scattering investigations. Other possible applications range from sensing to nano-optics/photonic devices.
Acknowledgement
Work at Alferov University was performed within the framework of the State Task ‘Active Composite Materials and Analysis Techniques for (Bio)Sensorics’ (Theme Code FSRM-2023-0009).
Disclosure statement
No potential conflict of interest was reported by the author(s).
Additional information
Funding
References
- Jin, S.; Meng, X.; Jin, S.; Zhu, M. J. Nanosci. Nanotechnol. 2013, 13, 1282–1285.
- Zakaria, H.M.; Shah, A.; Konieczny, M.; Hoffmann, J.; Nijdam, A.J.; Reeves, M.E. Langmuir 2013, 29, 7661–7673.
- Song, J.; Kim, D.; Lee, D. Langmuir 2011, 27, 13854–13860.
- Rempel, A.A. Adv. Chem. 2007, 76 (5), 474–500.
- Studzinskii, V.M.; Karabeshkin, K.V.; Kondrateva, A.S.; Fedorenko, E.D.; Karaseov, P.A.; Mishin, M.V. St. Petersburg State Polytech. Univ. J. Phys. Math. 2022, 15 (3.2), 69–74. doi:10.18721/JPM.153.213
- Lo Savio, R.; Repetto, L.; Guida, P.; Angeli, E.; Firpo, G.; Volpe, A.; Ierardi, V.; Valbusa, U. Solid State Comm. 2016, 240, 41–45.
- Mikhaylov, A.N.; Kostyuk, A.B.; Korolev, D.S.; Zhavoronkov, I.Y.; Chugrov, I.A.; Belov, A.I.; Burdov, V.A.; Ershov, A.V.; Tetelbaum, D.I. Bull. Rus. Acad. Sci. Phys. 2012, 76, 214–217.
- Prakash, J.; Tripathi, A.; Rigato, V.; Pivin, J.C.; Tripathi, J.; Chae, K.H.; Gautam, S.; Kumar, P.; Asokan, K.; Avasthi, D.K. J. Phys. D: Appl. Phys. 2011, 44, 125302.
- Tuzhilkin, M.S.; Bespalova, P.G.; Mishin, M.V.; Kolesnikov, I.E.; Karabeshkin, K.V.; Karaseov, P.A.; Titov, A.I. Semiconductors 2020, 54 (1), 137–143.
- Raceendran, P.; Fu, J.; Wallen, S.L. Green Chem. 2006, 8, 34–38.
- Popok, V.N.; Barke, I.; Campbell, E.E.B.; Meiwes-Broer, K.-H. Surf. Sci. Rep. 2011, 66 (10), 347–377. DOI: 10.1016/j.surfrep.2011.05.002.
- Ziegler, J.F.; Ziegler, M.D.; Biersack, J.P. Nucl. Instrum. Meth. Phys. Res. B. 2010, 268 (11-12), 1818–1823. DOI: 10.1016/j.nimb.2010.02.091.