ABSTRACT
There is a growing fear that bacteria are emerging that are resistant to most known antibiotics, which could lead to an increasing number of fatalities due to bacterial infections. There is therefore an urgent need for the development of novel antibacterial products, preferably with novel mode of actions for which there are no known resistance pathways. We have previously found serendipitously that a simple urea-based compound functions as a potent antibacterial agent. In this manuscript, we use bacterial cytological profiling (BCP) to determine its mechanism of action. BCP reveals that the urea is acting through a membrane-based pathway that is comparable to, but distinct from, the mechanism of cationophore calcimycin. Additional liposome-based assays suggest that the likely mechanism of action of this mono-urea is transmembrane anion transport. This finding highlights that synthetic anionophores could be used as potential antibiotics.
Graphical abstract
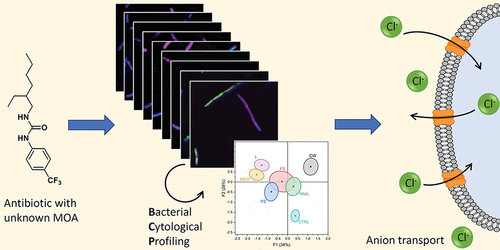
Introduction
The increase in multidrug-resistant bacteria combined with insufficient development of new antibiotics is creating a global health crisis [Citation1,Citation2]. Deepening our understanding of antibiotic mechanisms can provide useful insights to help increase the speed of drug development [Citation3]. Traditional antibiotics are classified according to 5 different modes of action: inhibition of cell wall synthesis, protein synthesis, nucleic acid synthesis, or metabolic pathways (folate synthesis), and membrane disruption [Citation4]. The latter has received a lot of attention in recent years, because membrane disruption leads to fast killing of bacteria and is less prone to inducing resistance [Citation5,Citation6]. Previously, we designed a small library of antibacterial agents that contain crown ether and urea moieties to selectively bind to the bacterial membrane lipid PE (phosphatidylethanolamine) [Citation7]. We also included a number of control compounds, such as urea-based compound 1 (), to see if both the urea and crown ether moiety are necessary for strong PE binding and antibacterial activity. Even though compound 1 exhibited minimal binding of PE in 1H NMR titrations and liposome studies, the minimum inhibitory concentration (MIC) of compound 1 with B. cereus was promising (MIC = 6.5 µM). Because compound 1 was originally designed as an inactive control compound, we wanted to elucidate the mechanism of its potent antibacterial activity. In this manuscript, we report the use of bacterial cytological profiling (BCP) to determine the mechanism of 1, because it allows the unbiased determination of the mode of action using a combination of microscopy and statistical analysis. Our results indicate that synthetic urea-based compound 1 functions through a membrane-related mechanism, most likely transmembrane anion transport.
Results and discussion
Antibacterial activity
In our earlier study we showed that compound 1 possesses antibacterial activity against B. cereus (MIC = 6.5 µM) [Citation7], a bacterium that is closely related to the bioterrorism organism B. anthracis [Citation8]. In addition, compound 1 has been previously reported as a broad-spectrum antimicrobial agent in a patent from 1971, but details of its biological activity and its mode of action were never reported [Citation9]. The minimum inhibitory concentration (MIC) of compound 1 was therefore determined against a range of organisms using standard broth microdilution methods (Supplementary Information) [Citation10]. This revealed that compound 1 is also effective against other Gram-positive organisms, including the clinically relevant S. aureus, but not against Gram-negative bacteria such as E. coli (see ).
Bacterial cytological profiling
To determine the mechanism of the antibacterial activity of 1, we decided to use bacterial cytological profiling (BCP). In BCP, an antibacterial agent with unknown mode of action can be classified by comparing morphological changes in the bacteria induced by groups of antibiotics with various modes of action [Citation11]. In this study, Bacillus subtilis (ATCC 6051) was used because it is a relatively large model bacterium suitable for microscopy studies [Citation12]. The bacteria were stained with 3 fluorescent dyes in order to simultaneously visualise the cell membrane (FM4-64), the nucleoid (DAPI), and membrane permeability (Sytox Green). Morphological changes were analysed using an open-source image analysis software, CellProfilerTM, and compound 1 was grouped into a category of known antibiotics by clustering analysis [Citation11,Citation13,Citation14]. Six antibiotics from the five distinct mechanism classes were chosen based on previously reported BCP studies: phosphomycin and vancomycin (cell wall inhibition), gentamicin (protein synthesis inhibition), rifampicin (nucleic acid synthesis inhibition), BactrimTM (folate synthesis inhibition), and calcimycin (membrane disruption) [Citation11,Citation13,Citation15]. Two cell-wall targeting antibiotics, phosphomycin and vancomycin, were chosen because they impart very different changes to the cell phenotype. Vancomycin physically blocks cell wall synthesis by binding to the growing peptidoglycan chain, which leads to minimal changes in cell size.4 Phosphomycin targets the enolpyruvate transferase MurA enzyme within bacteria and creates misregulation during cell wall synthesis, resulting in elongated cells [Citation13,Citation16]. We included both extremes in morphology that can be induced by cell wall-targeting antibiotics to ensure accurate classification of the mechanism of compound 1.
For the BCP assay, B. subtilis was grown to an early exponential phase in sterile cation-adjusted Müller-Hinton broth, followed by 2 h incubation with a control (untreated or DMSO) or 5x MIC of each antibiotic in vitronectin-coated 96-well plates (see Supplementary Information) [Citation17]. To fluorescently stain the bacteria, 50 μL of dye mix (5 μg/mL DAPI, 2.5 μM Sytox Green, 10 μg/mL FM4-64 in Hank’s Balanced Salt Solution) was added to each well and incubated 45 minutes in the dark at 25°C. Plates were analysed within 12 hours on an inverted Nikon Eclipse Ti A1 confocal microscope. Experiments were performed in triplicate on different days with at least 50 cells analysed per well. Morphological changes induced by known antibiotics were consistent with previously reported results ( and Supplementary Information) [Citation11,Citation13,Citation17]. The separation of antibiotic classes was determined using linear discriminant analysis (LDA) based on 23 variables generated by CellProfilerTM (see Supplementary Information for details). LDA uses supervised pattern recognition to find combinations of features to separate classes of objects. LDA also allows pre-categorisation to maximise variability between categories and minimise variability within categories, and thereby helps to identify and weigh the important parameters that separate one category from another [Citation13,Citation18]. Thus, the untreated and DMSO-treated results were pre-categorised as ‘controls’ and phosphomycin and vancomycin were pre-categorised as ‘cell wall inhibitors’. The LDA analysis revealed that measurements of area, length, Sytox Green intensity, and circularity (form factor, eccentricity) all played a large role in separating the antibiotics based on their mode of action. Validation of the model was done using a sub-set of the data chosen randomly. The factor scores (F1-F4) generated from LDA were also used in Euclidean clustering analysis. This LDA and clustering analysis reveals that compound 1 is most closely related to the membrane-active antibiotic calcimycin, mainly due to the high Sytox Green intensity and similar cell shape () [Citation11]. In particular, the high Sytox Green intensity seen for compound 1 is indicative of a membrane-related mechanism of action, as Sytox Green is a membrane impermeable dye that will only show fluorescence upon binding DNA if the membrane is compromised [Citation19].
Figure 2. Images of B. subtilis after 2 h incubation with the specified antibiotic (5x MIC) or control. Composite images were generated by Fiji v2.3.0 with all three channels overlayed (FITC (green), DAPI (blue), and Cy5 (magenta)), and brightness enhanced for clarity. Scale bar = 5 µm.
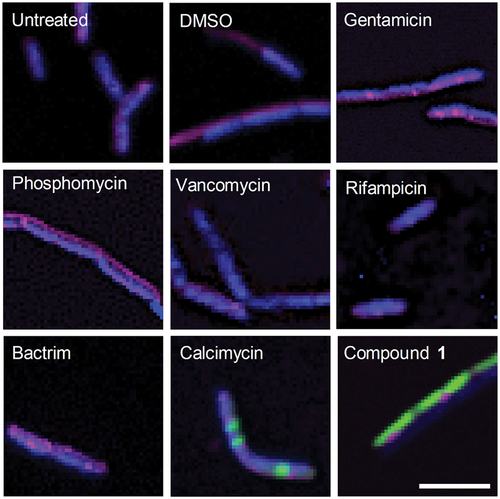
Figure 3. Results of the linear discriminant analysis (LDA) and Euclidean clustering analysis. Factor scores (F1-F4) are based on linear discriminant analysis (LDA) of the parameters calculated by CellProfilerTM. (a) F1 versus F2 and F1 versus F3 plots. Ellipsoids represent 90% confidence intervals of the mean and were generated using OriginPro 2023. (b) Heat map showing the intensities of factor scores F1-F4, and dendrogram generated from Euclidean clustering analysis of F1-F4 using Ward’s method. Graphs generated using OriginPro 2023.
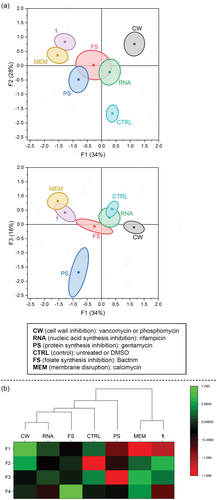
Transmembrane anion transport
While the BCP analysis clearly shows that compound 1 acts through a membrane-related mechanism, it is apparent that its mode of action is not the same as calcimycin. The small circular nucleoid induced by calcimycin is not observed for compound 1 (). Calcimycin is a Ca2+ ionophore [Citation20] and the structure of 1, as well as the BCP analysis, do not suggest that 1 can function through a similar cationophore mode of action. There are, however, many other types of antibacterial mechanisms that involve disruption of the membrane, such as pore formation, detergent-like lysis and lipid binding [Citation21,Citation22]. Liposome-based calcein assays showed no leakage of the entrapped dye out of the liposomes in the presence of 1 (Supplementary Information), indicating that pore formation or detergent-like cell lysis are unlikely mechanisms for 1. In addition, we originally included the known pore-forming antibiotic nisin [Citation23] in our microscopy studies for BCP analysis. However, nisin-treated cells are difficult to analyse because of its rapid induction of cell lysis [Citation13]. Images of B. subtilis treated with nisin show cloud-like structures stained with FM 4–64 after only 10 minutes of incubation, which indicate burst membranes of dead bacteria, as well as intact bacteria stained with Sytox Green (). Comparatively, compound 1 shows very little change in morphology after 10 minutes incubation and no permeabilization of Sytox Green (). This clearly shows that the mechanism of compound 1 is different to the mechanism of nisin. However, the slow uptake of Sytox Green in bacterial cells has been previously observed for many cationophores, such as CCCP (H+ transport), nigericin (H+/K+ transport), and valinomycin (K+ transport) [Citation24]. Whereas nisin forms large pores and causes Sytox Green permeabilization within minutes, cationophores do not directly disrupt the membrane and only alter ion homoeostasis. However, the altered ion gradients can lead to cell lysis and membrane permeabilization as a secondary effect and therefore require longer incubation times to observe Sytox Green uptake. While this is consistent with the behaviour observed for compound 1, cationophore activity is an unlikely mechanism for this small molecule as already discussed above.
Figure 4. Images of B. subtilis after 10 min incubation with nisin (0.25x MIC), or compound 1 (5x MIC). Composite images were generated by Fiji v2.3.0 with all three channels overlayed (FITC (green), DAPI (blue), and Cy5 (magenta)), and brightness enhanced for clarity. Scale bar represents 5 µm.
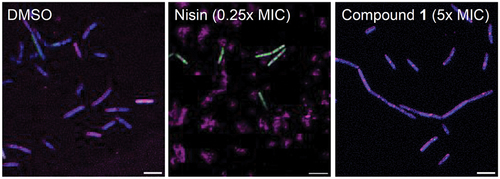
Our previous study had shown that compound 1 does not bind to the headgroup of the zwitterionic lipids PE (phosphatidylethanolamine) or PC (phosphatidylcholine) [Citation7]. However, bacterial membranes predominantly consist of negatively charged lipids, such as PG (phosphatidylglycerol) [Citation25]. 1H NMR titrations in DMSO:chloroform showed that 1 does not bind strongly to this lipid either (Ka = 23 M−1, see Supplementary Information). This implies that mode of actions resulting from lipid binding, such as membrane thinning, lipid clustering or changes in membrane fluidity, are also unlikely. One of the remaining possible mechanisms for the antibacterial activity of 1 is transmembrane transport of anions (anionophore activity). Similar small-molecule ureas have previously been show to transport chloride in model liposomes [Citation26,Citation27]. Anion transport is challenging to prove using BCP analysis due to the lack of appropriate control antibiotics with the same mode of action. Prodigiosin is a well-known natural product with antimicrobial activity that has anion transport ability [Citation28,Citation29]. However, prodigiosin is a pure electroneutral H+/Cl− transporter [Citation30], and is also able to induce DNA damage [Citation31] and generate reactive oxygen species [Citation32]. The controversy regarding the exact mechanism of prodigiosin makes it unsuitable as a reference antibiotic in BCP studies [Citation33,Citation34]. To investigate the anion transport ability of compound 1, we therefore reverted to liposome-based studies. All liposome studies used large unilamellar vesicles (LUVs) made from anionic POPG lipids, because this better reflects the bacterial membrane lipid composition (natural membranes, of course, also include numerous membrane proteins which are absent in these model studies).
Initially, we investigated the ability of 1 to facilitate the efflux of Cl– out of POPG liposomes using a standard ion selective electrode (ISE) assay. In brief, 200 nm LUVs encapsulating NaCl are prepared and suspended into a NaNO3 solution. Chloride efflux induced upon the addition of various concentrations of compound 1 is then measured using a chloride-selective ISE. The results show that 1 is able to facilitate chloride transport under these conditions, with and EC50,300s value of 0.85 ± 0.16 mol% with respect to lipid (Supplementary Information). The most likely mechanisms for chloride transport in this assay is Cl–/NO3 – exchange or Na+/Cl– symport, and in both cases transport can be electrogenic or electroneutral. To understand the anion transport mechanism of compound 1 in more detail, we preformed the valinomycin-monensin assay developed by Gale and co-workers [Citation35,Citation36]. LUVs encapsulating KCl are dispersed into a buffered potassium gluconate solution, and Cl– efflux facilitate by either 1 alone, 1+valinomycin or 1+monensin is measured using an ISE (). Gluconate is a highly polar anion and Cl–/gluconate exchange is therefore not possible, and transport can only occur via K+/Cl– symport. In the absence of valinomycin or monensin, compound 1 is not able to facilitate any chloride efflux (). This indicates that 1 alone cannot function as a K+/Cl– symporter, in agreement with the lack of cationophore activity suggested by the BCP analysis in B. subtilis. In the presence of valinomycin (an electrogenic K+ transporter) [Citation37] and in the presence of monensin (an electroneutral H+/K+ exchanger) [Citation38] fast chloride efflux is observed (). This suggests that 1 can facilitate both electrogenic Cl– transport and electroneutral H+/Cl– symport (or OH–/Cl– exchange). Previous studies have indicated that OH–/Cl– exchange is more likely for simple urea-based anionophores [Citation35]. In addition, the fact that compound 1 alone does not cause leakage of Cl– when gluconate is the external anion () and does not lead to leakage of calcein entrapped in liposomes (Supplementary Information), suggests that it most likely functions as an anion carrier. This is further corroborated by the differences in bacterial morphology induced by nisin (a pore/channel) and compound 1 (an anion-selective mobile carrier) ().
Figure 5. Valinomycin-monensin assay. (a) Experimental principle. If chloride transport is observed in the presence of valinomycin (Val), transport is due to electrogenic processes. If chloride efflux is observed in the presence of monensin (Mon), transport is due to electroneutral processes involving H+ or OH−. (b) 200 nm POPG LUVs encapsulating a buffered 300 mM KCl solution (10 mM HEPES buffer, pH 7.4) were suspended in a buffered 300 mM potassium gluconate solution (10 mM HEPES, pH 7.4). Transport was started by the addition of a DMF solution of compound 1, valinomycin, monensin or combinations thereof. Transport was measured using an ISE and DMF was used as a control. Data represents the average of 3 independent trials and error bars show standard deviations.
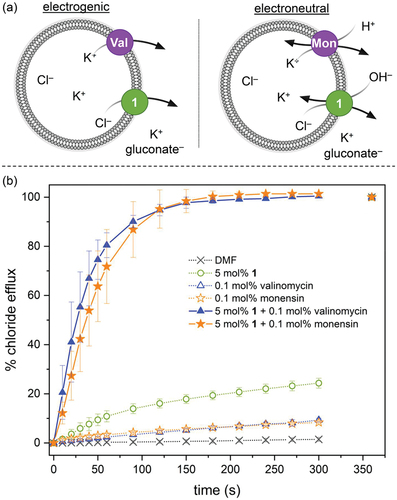
Haemolytic activity
Very few synthetic anionophores have been tested for antibacterial activity so far [Citation39–43]. This is somewhat surprising because synthetic anion transporters possess many desirable properties for antibiotic development. The membrane-related mechanism and the fact that its target is the abundant chloride anion, should make antibacterial resistance unlikely. Furthermore, the difference in lipid composition [Citation25], membrane curvature and membrane potential [Citation44] between bacterial cells and human cells provides a means to create selectivity towards bacteria. However, it is not uncommon for lipophilic molecules to insert themselves randomly in membranes and thereby cause membrane damage and leakage [Citation45]. We thus determined if compound 1 would exhibit non-selective membrane lysing in human erythrocytes following a standard haemolysis assay (Supplementary Information) [Citation46]. Compound 1 exhibited low haemolysis compared to its MIC (1.5–6 µM). Even at the highest concentration tested (400 µM), only 27% of red blood cells were lysed by 1. These results highlight that compound 1, and potentially other synthetic transmembrane anion transporters, could be potential leads for the development of potent and selective antibacterial agents.
Conclusions
In this manuscript, we found that a simple mono-urea displays potent antibacterial activity against a variety of Gram-positive bacteria. Bacterial cytological profiling revealed that the mode of action of this compound is membrane-related, but distinct from known cationophores (calcimycin) and pore-forming antibiotics (nisin). Liposome-based studies suggest that the mono-urea instead functions as an electrogenic and electroneutral anion transporter, and indicate that synthetic anionophores have potential in the quest for new antibiotics.
Supplemental Material
Download PDF (3 MB)Acknowledgments
The authors would like to thank Tulane University for generous start-up funds and the Louisiana Board of Regents for funding (Grant No. LEQSF(2018-21)-RD-A-15).
Disclosure statement
No potential conflict of interest was reported by the author(s).
Data Availability Statement
Raw data is available from the authors upon request.
Supplementary material
Supplemental data for this article can be accessed online at https://doi.org/10.1080/10610278.2023.2178921.
Additional information
Funding
References
- Blair JMA, Webber MA, Baylay AJ, et al. Molecular mechanisms of antibiotic resistance. Nat. Rev. Microbiol. 2015;13(1):42–51.
- Nelson RE, Hatfield KM, Wolford H, et al. National Estimates of Healthcare Costs Associated With Multidrug-Resistant Bacterial Infections Among Hospitalized Patients in the United States. Clin. Infect. Dis. 2021;72(Suppl Supplement_1):S17–S26.
- Hudson MA, Lockless SW. Elucidating the Mechanisms of Action of Antimicrobial Agents. mBio. 2022;e02240–21.
- Reygaert WC. An overview of the antimicrobial resistance mechanisms of bacteria. AIMS Microbiology. 2018;4(3):482.
- Delcour AH. Outer membrane permeability and antibiotic resistance Biochime. Biophys. 2009;1794(5):808–816.
- Epand RM, Walker C, Epand RF, et al. Molecular mechanisms of membrane targeting antibiotics. Biochim Biophys Acta. 2016;1858(5):980–987.
- Herschede SR, Gneid H, Dent T, et al. Bactericidal urea crown ethers target phosphatidylethanolamine membrane lipids. Org Biomol Chem. 2021;19(17):3838–3843.
- Helgason E, Økstad OA, Caugant DA, et al. Bacillus anthracis, bacillus cereus, and bacillus thuringiensis—one species on the basis of genetic evidence. Appl Environ Microbiol. 2000;66(6):2627.
- Duerr D, Hitz HR, Duennenberger M, et al., N-2-ethylhexyl-n’-aryl ureas as antibacterial agents. 1971. U.S. Patent No. 3,592,932.
- Balouiri M, Sadiki M, Ibnsouda SK. Methods for in vitro evaluating antimicrobial activity: a review. J Pharm Anal. 2016;6(2):71–79.
- Nonejuie P, Burkart M, Pogliano K, et al. Bacterial cytological profiling rapidly identifies the cellular pathways targeted by antibacterial molecules. Proc Natl Acad Sci U S A. 2013;110(40):16169.
- Levin PA, Angert ER. Small but mighty: cell size and bacteria. Cold Spring Harb Perspect Biol. 2015;7(7):a019216.
- Lamsa A, Lopez-Garrido J, Quach D, et al. Rapid inhibition profiling in Bacillus subtilis to identify the mechanism of action of new antimicrobials. ACS Chem Biol. 2016;11(8):2222–2231.
- Carpenter AE, Jones TR, Lamprecht MR, et al. CellProfiler: image analysis software for identifying and quantifying cell phenotypes. Genome Biol. 2006;7(10):1–11.
- Schafer AB, Wenzel M. A how-to guide for mode of action analysis of antimicrobial peptides. Front Cell Infect Microbiol. 2020;10:540898.
- Cushnie TP, O’Driscoll NH, Lamb AJ. Morphological and ultrastructural changes in bacterial cells as an indicator of antibacterial mechanism of action. Cell Mol Life Sci. 2016;73(23):4471–4492.
- Sridhar S, Forrest S, Warne B, et al. High-content imaging to phenotype antimicrobial effects on individual bacteria at scale. mSystems. 2021;6(3):e00028–21.
- Quach DT, Sakoulas G, Nizet V, et al. Bacterial cytological profiling (BCP) as a rapid and accurate antimicrobial susceptibility testing method for staphylococcus aureus. eBioMedicine. 2016;4:95–103
- Roth BL, Poot M, Yue ST, et al. Bacterial viability and antibiotic susceptibility testing with SYTOX green nucleic acid stain. Appl Environ Microbiol. 1997;63(6):2421–2431.
- Guyot J, Jeminet G, Prudhomme M, et al. Interaction of the calcium ionophore A.23187 (Calcimycin) with Bacillus cereus and Escherichia coli. Lett Appl Microbiol. 1993;16(4):192–195.
- Nguyen LT, Haney EF, Vogel HJ. The expanding scope of antimicrobial peptide structures and their modes of action. Trends Biotechnol. 2011;29(9):464–472.
- Williams ES, Gneid H, Marshall SR, et al. A supramolecular host for phosphatidylglycerol (PG) lipids with antibacterial activity. Org Biomol Chem. 2022;20(30):5958–5966.
- Breukink E, van Heusden HE, Vollmerhaus PJ, et al. Lipid II is an intrinsic component of the pore induced by nisin in bacterial membranes. J Biol Chem. 2003;278(22):19898–19903.
- Lamsa A, Liu W-T, Dorrestein PC, et al. The Bacillus subtilis cannibalism toxin SDP collapses the proton motive force and induces autolysis. Mol Microbiol. 2012;84(3):486–500.
- Epand RF, Savage PB, Epand RM. Bacterial lipid composition and the antimicrobial efficacy of cationic steroid compounds (Ceragenins). Biochim. Biophys. Acta, Biomembr. 2007;1768(10):2500–2509.
- Andrews NJ, Haynes CJE, Light ME, et al. Structurally simple lipid bilayer transport agents for chloride and bicarbonate. Chem Sci. 2011;2(2):256–260.
- Moore SJ, Wenzel M, Light ME, et al. Towards “drug-like” indole-based transmembrane anion transporters. Chem Sci. 2012;3(8):2501–2509.
- Seganish JL, Davis JT. Prodigiosin is a chloride carrier that can function as an anion exchanger. Chem Commun. 2005;46:5781–5783.
- Rastogi S, Marchal E, Uddin I, et al. Synthetic prodigiosenes and the influence of C-ring substitution on DNA cleavage, transmembrane chloride transport and basicity. Org Biomol Chem. 2013;11(23):3834–3845.
- Sato T, Konno H, Tanaka Y, et al. Prodigiosins as a New Group of H+/Cl−Symporters That Uncouple Proton Translocators. J Biol Chem. 1998;273(34):21455–21462.
- Melvin MS, Tomlinson JT, Saluta GR, et al. Double-Strand DNA Cleavage by Copper·Prodigiosin. J Am Chem Soc. 2000;122(26):6333–6334.
- Kimyon Ö, Das T, Ibugo AI, et al. Serratia secondary metabolite prodigiosin inhibits pseudomonas aeruginosa biofilm development by producing reactive oxygen species that damage biological molecules. Front Microbiol. 2016;7:972.
- Lin S-R, Chen Y-H, Tseng F-J, et al. The production and bioactivity of prodigiosin: quo vadis? Drug Discov Today. 2020;25(5):828–836.
- Choi SY, Lim S, K-h Y, et al. Biotechnological Activities and Applications of Bacterial Pigments Violacein and Prodigiosin. J Biol Eng. 2021;15(1):10.
- Wu X, Judd LW, HoweENW, et al. Nonprotonophoric Electrogenic Cl− Transport Mediated by Valinomycin-like Carriers. Chem. 2016;1(1):127–146.
- Wu X, Howe ENW, Gale PA. Supramolecular Transmembrane Anion Transport: new Assays and Insights. Acc Chem Res. 2018;51(8):1870–1879.
- Pinkerton M, Steinrauf L, Dawkins P. The molecular structure and some transport properties of valinomycin. Biochem Biophys Res Commun. 1969;35(4):512–518.
- Łowicki D, Huczyński A. Structure and Antimicrobial Properties of Monensin A and Its Derivatives: summary of the Achievements. Biomed Res Int. 2013;2013:742149.
- Share AI, Patel K, Nativi C, et al. Chloride anion transporters inhibit growth of methicillin-resistant Staphylococcus aureus (MRSA) in vitro. Chem Commun. 2016;52(48):7560–7563.
- Elie C-R, Hébert A, Charbonneau M, et al. Benzimidazolium-based synthetic chloride and calcium transporters in bacterial membranes. Org Biomol Chem. 2013;11(6):923–928.
- Elie CR, David G, Schmitzer AR. Strong Antibacterial Properties of Anion Transporters: a Result of Depolarization and Weakening of the Bacterial Membrane. J Med Chem. 2015;58(5):2358–2366.
- Gravel J, Schmitzer AR. Imidazolium and benzimidazolium-containing compounds: from simple toxic salts to highly bioactive drugs. Org Biomol Chem. 2017;15(5):1051–1071.
- Carreira-Barral I, Rumbo C, Mielczarek M, et al. Small molecule anion transporters display in vitro antimicrobial activity against clinically relevant bacterial strains. Chem Commun. 2019;55(68):10080–10083.
- Benarroch JM, Asally M. The Microbiologist’s Guide to Membrane Potential Dynamics. Trends Microbiol. 2020;28(4):304–314.
- Matsuzaki K. Control of cell selectivity of antimicrobial peptides. Biochim. Biophys. Acta, Biomembr. 2009;1788(8):1687–1692.
- Oddo A, Hansen PR. Hemolytic Activity of Antimicrobial Peptides. In: Hansen PR, editor. Antimicrobial Peptides: methods and Protocols. New York: Springer New York; 2017. p. 427–435.