Abstract
The COVID-19 pandemic is caused by the severe acute-respiratory-syndrome-coronavirus-2 that uses ACE2 as its receptor. Drugs that raise serum/tissue ACE2 levels include ACE inhibitors (ACEIs) and angiotensin-II receptor blockers (ARBs) that are commonly used in patients with hypertension, cardiovascular disease and/or diabetes. These comorbidities have adverse outcomes in COVID-19 patients that might result from pharmacotherapy. Increasing ACE2 could potentially increase the risk of infection, severity or mortality in COVID-19 or it might be protective as it forms angiotensin-(1–7) which exhibits anti-inflammatory/anti-oxidative effects and prevents diabetes- and/or hypertension-induced end-organ damage. Thus, there existed clinical uncertainty. Here, we review studies implicating 15 classes of drugs in increasing ACE2 levels in vivo and the available literature on the clinical safety of these drugs in COVID-19 patients. Further, in a re-analysis of clinical data from a meta-analysis of 9 studies, we show that ACEIs/ARBs usage was not associated with an increased risk of all-cause mortality. Literature suggests that ACEIs/ARBs usage generally appears to be clinically safe though their use in severe COVID-19 patients might increase the risk of acute renal injury. For definitive clarity, further clinical and mechanistic studies are needed in assessing the safety of all classes of ACE2 raising medications.
Introduction
The current pandemic of coronavirus disease 2019 (COVID-19) arises from the highly infectious, novel coronavirus termed as severe acute respiratory syndrome coronavirus 2 (SARS-CoV-2) that was identified in late 2019 in Wuhan, Hubei Province, China. The World Health Organisation declared it as a pandemic on March 11, 2020. At the time of writing (29 June, 2020), there have been over 10 Million cases worldwide with around 500,000 deaths. The clinical spectrum of COVID-19 is diverse and ranges from asymptomatic upper respiratory infection to critically ill pneumonia associated with acute respiratory distress syndrome (ARDS) [Citation1,Citation2]. Reports suggest that older age, and co-morbidities such as hypertension, type 2 diabetes mellitus (T2DM), and cardiovascular disease (CVD) are associated with greater rates of mortality in COVID-19 patients [Citation1]. Indeed, the increased mortality and morbidity of COVID-19 in patients with hypertension is an association that has now been observed in epidemiological studies [Citation3,Citation4]. For example, in a study of 191 patients with COVID-19, Zhou et al [Citation4] found hypertension to have a odds ratio of 3.05 (95% CI, 1·57–5·92) for in-hospital mortality. However, it remains unclear if this association was related to the underlying pathology (i.e. hypertension) or another associated comorbidity or pharmacotherapy.
There has been a growing concern that this association with hypertension is confounded by treatment with specific antihypertensive medications. Indeed, many of COVID patients with hypertension and/or CVD are routinely treated with therapies (such as ACE inhibitors (ACEIs), angiotensin II receptor blockers (ARBs)), mineralocorticoid receptor inhibitors (MCRIs) and statins) that can potentially raise serum or tissue levels of the membrane-bound form of the monocarboxypeptidase angiotensin-converting enzyme 2 (ACE2). Since ACE2 is the receptor by which SARS-CoV-2 enters and infects human cells, use of ACE2-raising drugs, in particular ACEIs and ARBs, have led to concerns regarding whether these drugs would increase the likelihood of infection, increase disease severity or even mortality in COVID-19 patients. Though these concerns were mostly hypothetical, they rapidly led to speculation that ACEIs and ARBs might be harmful in patients with COVID-19 and the resulting confusion amongst clinicians and patients alike has culminated in the publication of several observational studies on the clinical safety of ACEIs/ARBs in COVID-19 patients. In this article, we review the reasons for this initial confusion, examine the evidence for and provide a listing of the 15 classes of clinically used drugs that are reported to raise ACE2 in vivo, and further appraise key recent literature on the clinical safety of these ACE2 raising drugs, in particular ACEIs and ARBs, in COVID-19 patients.
To address some of the key issues, it is necessary to consider the role of ACE2 not only in COVID-19 pathogenesis but also as a component of renin-angiotensin-aldosterone system (RAAS) – a key regulator of homeostasis in both the cardiovascular and respiratory systems.
ACE2 is the receptor by which SARS-CoV2 enters human cells to cause COVID-19 disease
Angiotensin-converting enzyme 2 (ACE2) is a 100 kD type I cell-surface glycoprotein consisting of 805 amino acids that was first discovered 20 years ago [Citation5,Citation6]. ACE2 is a member of the membrane-bound carboxydipeptidase family of enzymes and is widely distributed in the human body, including the lungs, heart, kidney, small intestine, oral mucosa and nasal cavities [Citation7–9]. In the lungs, ACE2 expression is predominantly in type II alveolar cells and macrophages with modest expression in the epithelial cells lining the bronchi and trachea [Citation8].
Similar to SARS-CoV, the coronavirus that caused the SARS epidemic in China back in 2002-2003, the causative agent of COVID-19, SARS-CoV-2, also enters host target cells using ACE2 as its cell surface receptor [Citation4,Citation10,Citation11]. Thus, utilisation of ACE2 receptor as a mode of entry appears to be conserved amongst these coronaviruses. The viral spike glycoprotein (S protein) of SARS-CoV-2 binds to the membrane-bound ACE2 to facilitate viral attachment to target epithelial and/or endothelial cells [Citation12]. Yan et al. [Citation11] reported the structural basis of SARS-CoV2 interaction with ACE2 whereby the trimeric SARS-CoV-2 spike protein binds the peptidase domain of ACE2. This is followed by the cleavage of ACE2 C-terminal segment (residues 697 to 716) by the transmembrane protease serine 2 (TMPRSS2) that allows SARS-CoV-2 viral particles to fuse with host cell membrane with subsequent viral entry and replication in target cells [Citation10,Citation11]. The importance of ACE2 as an entry portal for coronaviruses was also inferred from studies in ACE2 knockout mice where viral infection and replication of SARS-CoV infection were markedly reduced [Citation13]. However, despite the S-protein of SARS-CoV sharing nearly 80% amino acid identity with that of the SARS-CoV-2, there are some important differences. Unlike SARS-CoV, SARS-CoV-2 does not appear to use human dipeptidyl peptidase-4 (DPP4) as a receptor [Citation14] and SARS-CoV-2 has a 10 to 20-fold higher binding affinity for ACE2 [Citation15] that likely explains its greater infectivity which has ultimately led to the current COVID-19 pandemic.
It is noteworthy that the cell surface/membrane-bound ACE2 can be cleaved by a disintegrin and metalloprotease 17 (ADAM-17) -also known as tumour necrosis factor-α converting enzyme (TACE)- to release or ‘shed’ a soluble bioactive form of ACE2 (ACE2s) [Citation16]. It is thought that ADAM-17-mediated proteolytic cleavage of ACE2 receptor can be activated by Ang II and SARS-CoV-2 and the resultant decrease in cell surface ACE2 may account for the lung injury observed in COVID-19 patients [Citation17]. ACE2 levels at the cell surface, intracellular and extra-cellular fluid will be determined from a balance between ACE2 synthesis, shedding and/or degradation. The precise impact of SARS-CoV-2 and/or ACE2-raising drugs, like ACEIs and ARBs, on these processes regulating tissue/fluid ACE2 levels and RAAS activity in COVID-19 patients are not known and requires further study (see also ).
Figure 1. ACE2 as a key component of the RAAS and as the cell surface receptor for SARS-CoV-2 infection. The RAAS comprises two ‘arms’ or pathways that are opposing or counter-regulating in their actions and it is their relative balance that determines the physiological/pathological consequences. This simplified schematic shows that upon conversion of Angiotensinogen into Angiotensin 1 by renin, the octapeptide Angiotensin II (Ang II) is produced by the action of angiotensin-converting enzyme (ACE) on Angiotensin I that can exert its actions via the AT1 receptor (AT1R). Ang II can also be converted by ACE2 to Angiotensin-(1-7) (Ang-1-7) that can act via it Mas receptor (MasR). The predominance of the ACE-Ang II- AT1 receptor ‘arm’ or pathway leads to actions such as vasoconstriction, cell proliferation, pro-inflammatory and pro-fibrotic signals whereas the activation of the ACE2-Ang-(1-7)-MasR pathway leads to opposing actions of vasodilation, anti-proliferative, anti-inflammatory and anti-fibrotic signals. In addition to its role in RAAS, ACE2 is the receptor by which SARS-CoV-2 gains entry into human cells. Endocytosis of the complex between ACE2 and SARS-CoV-2 viral particles may lead to a reduction in membrane-bound ACE2 levels. Membrane-bound ACE2 can be shed as a soluble form of ACE2 (ACE2s) following cleavage by a disintegrin and metalloprotease 17 (ADAM-17). ACE2s retains it biological activity and might also act as a ‘decoy’ receptor to inhibit SARS-CoV-2 binding to the intended membrane-bound ACE2 receptor. The sites of action for ACE inhibitors and Angiotensin Receptor Blockers (ARBs)-routinely used for treating hypertension and cardiovascular diseases- are shown and these drugs are known to raise ACE2 levels in various fluids/tissues in vivo. Overall balance of ACE2 levels on cells or in body fluids will be dependent on the balance of its synthesis, shedding and/or degradation. The potential interplay of SARS-CoV-2 and the impact of ACE2-rasing medications in COVID-19 patients and the potential implications for shifting the balance between the different ‘arms’ of RAAS is further discussed in the main text.
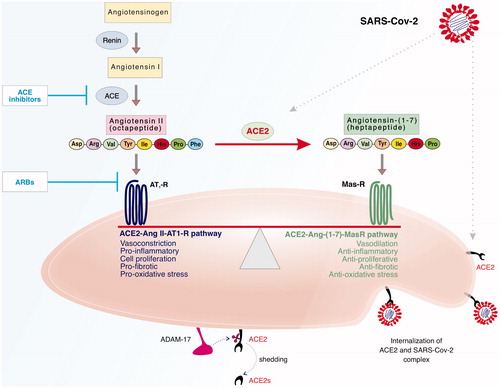
ACE2 levels are altered in several other pathological states including hypertension, diabetes, and respiratory disease [Citation18–20]. For example, we previously showed that induction of experimental diabetes in rats results in lowering of ACE2 protein levels [Citation21]. ACE2 expression and/or activity in different body fluids or tissues might actually result from either the underlying pathology, pharmacotherapy and/or compensatory mechanisms depending on the timing of when measurements were undertaken [Citation18–20]. Variations in ACE2 expression and genetic polymorphisms in ACE2 with resulting variation in enzyme activity have also been documented (for review see Devaux et al. 2020) [Citation22]. Women and children tend to have lower ACE2 expression and thus, it is tempting to correlate these with the more favourable/protective outcomes in such subject groups. On the other hand, older patients and patients with co-morbidities such as hypertension and diabetes also have altered ACE2 levels and are associated with more unfavourable outcomes with COVID-19. Thus, the true impact of variations in ACE2 genetic polymorphisms as well as gene and protein expression levels on COVID-19 pathology is not yet fully understood and requires further study.
ACE2 as a key link between the two counter-regulating arms of RAAS
The renin-angiotensin-aldosterone system (RAAS) is crucial to the homeostasis of both the cardiovascular and respiratory systems. The RAAS comprises two ‘arms’ or pathways that are opposing or counter-regulating in their actions and it is their relative balance that determines the physiological/pathological consequences [Citation20–26]; see ). Physiologically, the classical ‘arm’ of RAAS plays a vital role in regulating blood pressure and electrolyte balance [Citation23]. A key component of the classical RAAS is the octapeptide, angiotensin II (Ang II) that is produced via several biochemical steps in the body (see ). Renin from the kidneys converts angiotensinogen into angiotensin I. Then angiotensin-converting enzyme (ACE), secreted by the kidneys and lungs, metabolises angiotensin I into Ang II. It is the latter, through its type 1 (AT1) receptor that stimulates vasoconstriction, cardiovascular response, and aldosterone and anti-diuretic hormone production which culminates in increased blood pressure and body fluid volume through sodium, potassium, and free water resorption [Citation23]. Pathophysiologically, activation of the ACE-Ang II-AT1 receptor ‘arm’ leads to cell proliferation, pro-inflammatory, pro-oxidative stress and pro-fibrotic signals. Overactivity of this arm of the RAAS has been implicated in many pathologies including hypertension, cardiovascular diseases, diabetes-induced complications and respiratory diseases [Citation23–27] and can be countered by RAAS inhibitors, such as ACEIs, ARBs and MCRIs. RAAS inhibitors are commonly used in the management of hypertension, heart failure, cardiac myopathies, and for delaying progression of cardiac/renal pathologies [Citation28].
ACE2 appears to be the key link between the classical arm of the RAAS with its the non-classical or counter-regulating arm. In the latter, Ang II is metabolised/converted by ACE2 to Angiotensin-(1-7) (Ang-1-7) that can exert its actions via the G-protein coupled Mas receptor (MasR). The predominance of the ACE2-Ang-(1-7)-MasR ‘arm’ of RAAS leads to opposing actions of vasodilation, anti-proliferative, anti-inflammatory, anti-oxidative stress and anti-fibrotic signals [Citation23]. Indeed, we were the first, back in 1993, to show in a pithed rat model that Ang-(1-7) exerted blood pressure lowering effects opposite to those of Ang II and that these were likely mediated not via AT1 or AT2 receptors but most likely through a new, though at that time unidentified, Ang-(1-7) receptor [Citation29]. We later confirmed these depressor effects of Ang-(1-7) in animal models of hypertension [Citation30,Citation31]. We and others have subsequently shown that Ang-(1-7) via its MasR exerts anti-inflammatory, anti-oxidative and anti-fibrotic properties in cardiovascular and pulmonary systems [Citation21,Citation27,Citation32–35]. Activation of ACE2-Ang-(1-7)-MasR arm of the RAAS is protective in several pathologies including cardiovascular, respiratory and diabetes-induced complications [Citation36,Citation37,Citation20,Citation23,Citation27,Citation38,Citation39]. Indeed, it is now thought that part of the mechanism of action of RAAS inhibitors may actually involve raising levels of ACE2 and subsequent production of Ang-(1-7 [Citation40,Citation41]- that is to say a shifting of the balance towards the counter-regulating ACE2-Ang-(1-7)-MasR pathway. Thus, ACE2 plays a pivotal role in maintaining the balance between the two counter-regulating arms of the RAAS (see ). The dependence of SARS-CoV-2 infections on ACE2 receptor-mediated cell entry, and the possibility of ACE downregulation post-viral entry into host cells [Citation4,Citation13,Citation42,Citation43], suggests that available ACE2 levels could also be regulated by SARS-CoV2. Thus, coronavirus interactions with ACE2 likely impact on the overall balance of RAAS pathways and as such this interaction could even be considered as a new third ‘arm’ of the RAAS in COVID-19 patients- the so called RAAS-SARS-CoV-2-axis [Citation44]. Indeed, reduced ACE2 levels in the lung are a hallmark of acute lung injury [Citation13,Citation45–47], whereas diabetes and hypertension- known co-morbidities for unfavourable clinical outcomes in COVID-19 [Citation1]- are also thought to be associated with altered ACE2 activity. The precise SARS-CoV-2-induced biochemical changes in the various components of RAAS remain unknown and such studies would be necessary to determine the true pathophysiological impact of the RAAS-SARS-CoV-2-axis.
ACE2 raising drugs- what is the experimental evidence for clinically approved drugs in increasing ACE2 in vivo?
Experimental evidence, largely from animal studies, exists for the ability of at least 15 classes of medications, routinely used for treating patients with hypertension, cardiovascular diseases, diabetes and other conditions, to increase ACE2 in vivo (reviewed in ). Clinically approved drugs such as ARBs, ACEIs, MCRIs (known as RAAS inhibitors), statins, peroxisome proliferator-activated receptor-γ (PPAR-γ) agonists, glucagon-like peptide 1 receptor (GLP-1) agonists and non-steroidal anti-inflammatory drugs have been reported to raise ACE2 mRNA expression and/or protein levels in various tissues or plasma of animals or humans (see for more extensive listing).
Table 1. Examples of experimental studies providing evidence of raised ACE2 mRNA expression, protein levels and/or activity with clinically used medications.
Drug-induced stimulation of ACE2 expression and/or activity appears to be tissue-specific and variable amongst the disease models, stage of a given disease and amongst drug classes. The most widely studied of the ACE2-rasing drugs are the RAAS inhibitors (ACEIs, ARBs and MCRIs). ARBs, also known as angiotensin receptor antagonists or simply as ‘sartans’ (e.g. losartan, olmesartan, Irbesartan and telmisartan), are reported to raise ACE2 mRNA expression and/or protein levels/activity in the plasma, heart, kidney and aorta of animal models [Citation41,Citation49–54] (see also ). For example, Wang et al. [Citation76] reported that several ARBs, namely olmesartan, losartan, valsartan, candesartan, telmisartan, and irbesartan, can all increase ACE2 protein by about 2-fold in the hearts of aorta-constricted mice. Similar to ARBs, ACEIs such as enalapril and lisinopril are also reported to raise ACE2 mRNA expression and/or protein levels/activity in the plasma, heart, and kidney of animals [Citation49,Citation50,Citation56,Citation57]. However, the effects of these drugs were not always consistent as for example, neither enalapril nor losartan changed the level of ACE2 mRNA in the lung, ileum, kidney, or heart of male C57BL/6J mice [Citation77] thereby implying that modulation of ACE2 levels with RAAS inhibitors is likely to be tissue-specific and further dependent on the presence of pathology as use of these drugs in healthy animals did not lead to changes in ACE2 levels.
In human patients with heart failure, the MCRI, spironolactone, was shown to raise ACE2 activity and ACE2 mRNA expression in monocyte-derived macrophages one-month post therapy whereas in a mouse model, eplerenone increased cardiac ACE2 mRNA and protein activity in the heart [Citation59]. Statins, 3-hydroxy-3-methyl-glutaryl-coenzyme A (HMG-CoA) reductase inhibitors, can also increase cardiac and/or renal ACE2 protein in animal models [Citation60,Citation61].
Medications used for treating T2DM such as PPAR-γ agonists, also known as thiazolidinediones (TZDs) or simply as ‘Glitazones’ such as rosiglitazone and pioglitazone, have also been reported to increase ACE2 protein levels in the aorta or after chronic treatment in the liver, adipose tissue, and skeletal muscle [Citation62,Citation63]. In contrast, rosiglitazone was shown to reduce urinary ACE2 protein and activity but did not affect renal ACE2 levels in db/db diabetic mice [Citation78] implying tissue-specific and/or pathology specific effects of these ACE2 modulating drugs.
Liraglutide, a GLP-1-agonist or incretin hormone mimetic, also used for the treatment of diabetes, is the only medication reported to increase ACE2 expression in the lungs of rat models [Citation79–82]. An important unanswered question is whether this effect on ACE2 is specific to liraglutide or do all GLP1-receptor agonists, as well as dipeptidyl peptidase-4 (DPP4) inhibitors (that prevent GLP-1 degradation), raise ACE2 levels? We and other have shown that GLP1 hormone is secreted from relatively small population of the enteroendocrine L cells dispersed along the intestinal epithelium [Citation67–70]. GLP1 is known to potentiate insulin release through their distinct G-protein-coupled receptors (Gs proteins) highly expressed on pancreatic islet ß cells. When the Gs is activated simultaneously with rising glucose concentration, it leads to enhanced insulin release, thereby enabling the body to respond appropriately to ingested glucose entering the bloodstream [Citation83]. DPP4 is the enzyme responsible for the rapid N-terminal cleavage and inactivation of GLP-1. Both the intact form and inactivated GLP1 metabolites are rapidly cleared from the circulation via the kidney and therefore the pharmacological value of endogenous incretins is limited by their short half-life. Thus, two effective clinical strategies have been pursued to augment/preserve active GLP-1 hormone levels in the circulation for the treatment of T2DM. Firstly, by developing several structurally refined GLP1 receptor (GLP1R) agonists (such as Exenatide, Semaglutide and Liraglutide) and secondly by the use orally-acting DPP4 inhibitors (e.g. Saxagliptin, Linagliptin and Sitagliptin) that effectively raise endogenous GLP-1 levels by preventing its breakdown.
Various components of the RAAS have been reported in the mucosal and muscular layers of the gastrointestinal tract including in the colonic L cells where AT1 receptors were shown to be highly expressed (relative to the surrounding absorptive cell population) and stimulate GLP1 secretion in response to its ligand, Ang II [Citation84]. In a rat model of acute lung injury, Liraglutide, a GLP1 receptor agonist has been shown, independent of its glucose-lowering effect, to significantly raise ACE2 expression in lung tissue, increasing the hydrophilic surfactant protein A and the hydrophobic surfactant protein B [Citation65] which gets secreted by alveolar cells in the lung, and reduces the work of breathing [Citation85]. A similar result has been reported, using a mouse model of intrauterine growth retardation, in which Liraglutide potently stimulates the levels of ACE2 in the lung and prevents the reduction in surfactant protein A [Citation66].
In a disease model of cardiac fibrosis in the rat, treatment with either Liraglutide or the DPP4 inhibitor, Linagliptin, has been reported to increase ACE2 activity, and both are thought to exert cardioprotective effects [Citation67]. Thus, data so far suggest that the GLP-1 agonist and the DPP4 inhibitor Linagliptin can both raise ACE2 levels in the lungs and the heart of animals. However, whether this also occurs in humans and the relative importance of this finding in COVID-19 patients requires further study.
The non-steroidal anti-inflammatory agent, Ibuprofen, has also been implicated in raising ACE2 levels. In a study of rats with type 1 diabetes, Ibuprofen raised cardiac ACE2 protein levels, or more correctly, attenuated the diabetes-induced reduction in ACE2 levels [Citation72]. This is a common and noteworthy finding that for many of the drugs reported to raise ACE2 levels (see ), their ACE2-raising ability in vivo is usually seen only in the disease model and often reflects their ability to correct, normalise or attenuate disease-induced reductions in ACE2.
More importantly, strong clinical evidence is lacking regarding the ability of any of the above discussed medications (see also ), alone or in combination, on actually increasing ACE2 in human tissues in vivo especially the lungs that is of great importance to COVID-19 pathology. So what evidence, if any, exists in humans? Of note, there is a small study showing patients treated with ACEIs, but not ARBs, had increased mRNA levels of ACE2 in small intestine biopsy samples taken during routine gastroduodenoscopy and ileocolonoscopy [Citation58]. In contrast, ACEIs had no effect on ACE2 protein levels in renal biopsies from patients with renal pathologies, including recipients of kidney transplant [Citation86]. Other human studies have focussed on investigating plasma ACE2 activity or urinary ACE2 levels in patients who have received chronic treatment with ACEIs or ARBs but most have failed to show any significant changes [Citation87–90]. In a study of Japanese patients with hypertension, urinary ACE2 levels were reported to be higher among patients who received long-term treatment with olmesartan but not with other ARBs (losartan, candesartan, valsartan, and telmisartan) or with enalapril, an ACEI, compared to untreated control patients [Citation55]. Yet other human studies have measured Ang-(1-7) levels as a surrogate for ACE2 activity. In patients with essential hypertension exposed to the ACEI captopril for a period of 6 months, plasma Ang-(1–7) levels have been reported to be increased [Citation91]. However, in another study in patients receiving ACEIs for coronary artery disease, Ang-(1–7) levels were not significantly altered [Citation92]. Thus, data from human studies, like the animal studies, is variable and some have questioned whether RAAS inhibitors have any direct effects on ACE2-directed Ang II metabolism in clinical settings [Citation92,Citation93]. Alternatively, these variable effects of individual drugs on ACE2 levels in humans and in animals might imply that drug-induced effects on ACE2 are likely drug-specific and may not be uniform within a drug-class or across all the different classes of ACE2-raising drugs [Citation93]. Further, ACE2 expression and/or Ang-(1–7) synthesis/metabolism may change during the course of the disease (so will likely vary depending on the stage of the disease at which experimental read-outs are taken) and plasma levels of ACE2-Ang-(1–7) may not always reflect changes at a tissue level, that will likely also be regulated by a ‘local’ tissue-specific RAAS in addition to the general influence of the circulatory RAAS [Citation94]. Thus, ACE2 measurements of either mRNA, protein or enzyme activity- which are not all the same- need to be clearly stated along with the details of the specific tissue or fluid, stage of the disease and/or type/dose/duration of pharmacological therapy received as these are important variables that may help explain the exact mechanistic role that ACE2 may play in its clinical impact in COVID-19 patients. Additionally, for a more complete mechanistic understanding, the full complement of RAAS components should be analysed to determine the balance (or lack of) in a given clinical setting such as ratios of ACE-Ang II versus ACE2- Ang-(1-7).
Thus far members of at least 15 different classes of drugs have been implicated in enhancing ACE2 in animal studies (see ) but It remains to be elucidated if other, as yet unidentified drugs or drug classes can raise ACE2 mRNA or protein levels in humans. The precise mechanisms by which non-RAAS inhibitors such as statins, PPAR-γ agonists, ibuprofen and incretin mimetics etc, raise ACE2 levels is unclear. Chronic therapy (e.g. more than 1 month use of ACEIs/ARBs) is often needed to raise plasma/urine ACE2 levels in patients [Citation18,Citation19]. Furthermore, since many of these drugs are often used in combination such as in diabetes where ACEIs can be given together with TZDs, incretin mimetics or PPAR-γ agonists, the additive or synergistic effects of drug combinations on ACE2 remain unknown. It is also still unknown whether the drug-induced increases in ACE2 levels actually occur in COVID-19 patients and what their impact might be on cell entry or subsequent pathology of SARS-CoV-2 infection. Answers to these key questions should be sought in large-scale clinical studies with full biochemical profiling of RAAS components to fully elucidate the impact of ACE2-raising medications in COVID-19.
The concerns and confusion caused in the early stages of the pandemic around the potential use of ACE2-raising medications in COVID-19 patients
The major concern in the early stages of the pandemic was that if ACE2 levels are raised by commonly used medications (see ) then this will likely lead to increased number of ACE2 receptors for binding to SARS-CoV-2 and thus result in either increased risk of infection, severity of disease and/or mortality. For use of ACEIs/ARBs in COVID-19 patients, this concern was first raised in a hypothetical argument, without any experimental evidence, by Fang et al [Citation95] and subsequently gained momentum with other similar viewpoints [Citation96,Citation98]. The limited amount of clinical evidence available at the time appeared to support this ‘harm’ viewpoint and added to the controversy and ensuing confusion. It was thought that since many COVID-19 patients with co-morbidities like diabetes, hypertension, and other cardiovascular pathologies, that had increased susceptibility and adverse outcomes [Citation99,Citation100], were also on ACEI and ARBs, it was quite likely that this pharmacotherapy, at least in part, might be responsible for the unfavourable outcomes. Further fuel for the potential harm argument was presented by a retrospective study of 187 patients with COVID-19, where 19 patients on ACEIs or ARBs were associated with elevated troponin levels- a marker of adverse clinical outcomes [Citation101]. Taken together, these reports led to speculation that ACE2 raising medications, especially ACEIs and ARBs, might be harmful in patients with COVID-19.
Alternative perspectives highlighting the potential benefits of administering exogenous ACE2 or increasing levels of endogenous ACE2 by ACE2-raising drugs were also suggested [Citation102–104]. The potential use of soluble recombinant ACE2 was proposed as a competitive decoy to ‘mop up’ SARS-CoV2 virus and prevent its binding to the intended ACE2 membrane-bound target receptor [Citation105]. Additionally, it was thought that a reduction in membrane-bound ACE2, following endocytosis of ACE2 receptors post-SARS-CoV-2 infection (similar to that reported for SARS-CoV) would prevent its normal functions [Citation13,Citation42,Citation43,Citation106] and might predispose COVID-19 patients to lung injury. Thus, ACE2-raising medications could conceivably prevent virus-induced reduction in ACE2 levels and thereby offer clinical benefit in infected patients where respiratory distress and lung injury are associated with severe COVID-19 pathology. Lending support to the benefit theory, a study of only 10 elderly COVID-19 patients with hypertension in which ARBs showed more favourable outcomes compared with those on alternative therapies was published online as a pre-print [Citation107]. Theories on the mechanistic rationale for benefit in COVID-19 patients appeared thereafter. A key driver being that by increasing ACE2, more Ang II would be metabolised to Ang-(1-7) thereby shifting the balance of RAAS (see ) towards the beneficial anti-inflammatory and anti-fibrotic ACE2-Ang-(1-7)-MasR pathway that might oppose the ‘cytokine storm’ and/or be protective in lung pathologies observed in COVID-19 patients. Indeed, ACE2 and angiotensin (1-7) have been found to be protective in asthma [Citation27] and a number of different lung injury models [Citation103]. A study in a mouse acid lung injury model suggested that SARS-CoV (the virus causing SARS) exacerbated lung injury by reducing ACE2 that could be corrected by the ARB losartan [Citation13]. It is argued that because of the similarity of SARS-CoV and SARS-CoV-2 viruses, increasing ACE2 expression might also attenuate SARS-CoV-2–induced lung injury. However, thus far there is no direct clinical evidence that treatment with ACE2-raising medications, such as ACEIs or ARBs, can decrease severity of pulmonary injury in COVID-19 patients.
There was also the argument that abruptly stopping important ACE2-raising medications for patients whose pathology had been stabilised with these otherwise clinically desirable and safe treatments would itself lead to adverse outcomes. Indeed, despite the lack of evidence, there have been advocates for both the use and cessation of ACEIs, and/or ARBs during the treatment for COVID-19 patients. Thus, not knowing whether to continue or halt ACE2-raising pharmacotherapies, especially ACEIs and ARBs, in COVID-19 represented a clinical uncertainty and a psychological burden on both clinicians and patients alike [Citation96,Citation98]. An urgent evidence-based resolution was required and several clinical observational studies were initiated- the results of several of these have now been published and are reviewed in the proceeding sections.
Clinical studies on safety of ACE2-raising medications: How safe are they in COVID 19 in patients?
ACEIs and ARBs
Several clinical observational studies on the association of ACEIs and ARBs with COVID-19 patient outcomes have now been published (see ). Focussing on peer-reviewed publications only, we have grouped studies into those examining the association of ACEIs and/or ARBs usage and either a) risk of infection, b) severity of COVID-19 disease, c) risk of admission to hospital and finally d) mortality (see ).
Table 2. Examples of clinical studies showing that COVID-19 patients taking ACEIs/ARBs are not associated with increased risk of either a) infection, b) severity of COVID-19 disease, c) risk of admission to hospital or d) mortality.
Do ACEIs/ARBs increase risk of getting COVID-19 infections?
No study to date has shown a significantly increased risk of acquiring COVID-19 infections in patients taking ACEIs or ARBs (see ). In a large population-based study from the Lombardy region in Italy, Mancia et al. [Citation108] conducted a study with a total of 6272 patients with confirmed SARS-CoV-2 infection that were compared with 30,759 controls matched according to age, sex, and municipality of residence. Of the 1502 COVID-19 patients on ACEIs and 1394 on ARBs (total 2896 on ACEIs/ARBs), Mancia et al. [Citation108] reported no significant association between use of these drugs and COVIDs-19 infection in conditional logistic-regression multivariate analysis, either with ARBs (adjusted odds ratio [OR] 0.95; 95% CI 0.86, 1.05) or with ACEIs (OR 0.96; 0.87, 1.07). However, it was not clear for how long prior to COVID-19 infection these patients were on these medications- a factor that will likely impact on the extent, if any, to which drug-induced ACE2 levels are elevated.
Patients generally need to be on long-term drug therapy of at least 1 month to observe increased ACE2 levels [Citation18,Citation19]. Taking this into account, in another large study of 12,594 COVID-19 patients in New York (USA), in which patients were on drugs for at least 1 month prior to COVID-19 infection, Reynolds et al. [Citation109] showed that in 1019 patients on either ACEIs or ARBs, after propensity-score matching and Bayesian analysis, there was no positive association of risk of infection with prior treatment with ACEIs (median difference 0.1%; 95% CI −4.3%, 4.5%) or ARBs (1.6%; −2.6%, 5.8%) compared with untreated patients. The authors also reported similar findings for several other antihypertensive drug classes [Citation109]. Another smaller, single-centre retrospective cohort study from Wuhan, China came to the same conclusion [Citation110]. Taken together, these published studies (see also ), across different geographical regions, show that, despite the more frequent use of ACEIs and ARBs among patients with COVID-19 (due to a higher prevalence of cardiovascular disease) compared to controls, there is no increased risk of acquiring COVID-19 infections for patients on these medications.
Are ACEIs/ARBs associated with increasing severity of COVID-19
Several observational clinical studies, mostly out of China, Italy or USA, have investigated the possibility that ACE2-raising ACEIs or ARBs might lead to more severe symptoms/pathology in COVID-19 patients and these included the studies of Reynolds et al. [Citation109] and Mancia et al. [Citation108] discussed above. Neither of these large COVID-19 patient groups showed increased risk of developing more severe symptoms of COVID-19 if on ACEIs or ARBs. For example, Reynolds et al. [Citation109] showed no significant increased risk, defined as ≥10% difference, of serious illness in COVID-19 patients on ACEIs (median difference −3.3%; 95% CI −8.2%, 1.7%) or ARBs (0.1%, −4.8%, 4.9%) compared with untreated patients. However, there was a slightly higher risk in patients who had received calcium-channel blockers (4.4%; 0.5%, 8.2%) but still below the ≥10% significance cut-off defined by the authors (see also section on effects with other ACE-2 raising drugs), but no differences in recipients of other antihypertensive drug classes [Citation109].
In a retrospective, single-centre case series of 1178 hospitalised COVID-19 patients in Wuhan, China [Citation112], Li et al also investigated the association between ACEIs/ARBs and severity of illness in patients with hypertension. Of the 115 COVID-19 patients on ACEI/ARB, there was no significant difference between those with severe versus non-severe symptoms taking ACEIs (9.2% vs 10.1%; p = .80), ARBs (24.9% vs 21.2%; p = .40), or the composite of ACEIs/ARBs (32.9% vs 30.7%; p = .65).
Similarly, three other smaller studies out of China (two from Wuhan and one from Shenzheng) also suggested that there was no increased likelihood of patients on ACEIs and ARBs having severe COVID-19 disease [Citation112,Citation113]. Interestingly, one study showed that the pro-inflammatory cytokine IL-6 levels were reduced with ACEIs/ARBs and neutrophil depletion was prevented as evidenced by increased CD3+ and CD + cells [Citation113] implying that ACEI/ARB-induced beneficial anti-inflammatory effects. Taken together, the above studies have not shown any significant increase in the likelihood of ACEIs/ARBs leading to increased severity of COVID-19 disease. However, a recent small study from France [Citation101] has suggested that ACEIs/ARBs usage in severe COVID-19 patients, may lead to increased risk of developing kidney dysfunction and acute kidney injury (see further discussion of this study in a later section).
Are ACEIs/ARBs associated with increasing risk of hospitalisations in COVID-19 patients?
Studies from Madrid (Spain) and Wuhan (China) have investigated the risk of requiring hospital admission with COVID-19 patients on ACEIs or ARBs. In a case population study by de Abajo et al. [Citation114] that collected data for 1139 cases and 11 390 controls, 484 of the COVID-19 patients were on ACEIs/ARBs. No increased risk of hospitalisation was observed with either ACEIs (adjusted OR 0·80, 95%CI 0·64–1·00) or ARBs (1·10, 0·88–1·37). Age, gender and background cardiovascular risk were reported not to modify the adjusted OR between use of ACEIs/ARBs and COVID-19 patients requiring hospital admission. Interestingly, de Abajo et al. [Citation114] noted a decreased risk of hospitalisation for COVID-19 patients with diabetes also taking these RAAS inhibitors (adjusted OR 0·53, 95% CI 0·34–0·80) implying that ACEIs/ARBs provide greater benefit to COVID-19 patients with diabetes (the implications of this are further discussed in a later section). A smaller single-centre retrospective cohort study of 43 COVID-19 patients on ACEIs/ARBs from China [Citation110], also reported no significant increase in likelihood of being admitted to hospital if on ACEIs/ARBs therapy. The results of these studies examining risk of hospitalisation are, therefore, consistent with those showing no increase in the likelihood of ACEI/ARBS leading to increased severity of COVID-19 disease.
Are ACEIs/ARBs associated with increasing risk of mortality in COVID-19 patients?
When examining whether exposure to ACEIs/ARBs increases the risk of mortality in COVID-19 patients, several studies have now reported no association with enhanced risk of all-cause mortality [Citation101,Citation112] (Mancia et al. [Citation108]; See also ). For example, Li et al [Citation112] showed no differences between non-survivors and survivors on ACEIs (9.1% vs 9.8%; p = .85), ARBs (19.5% vs 23.9%; p = .42), or the composite of ACEIs/ARBs (27.3% vs 33.0%; p = .34). However, others have reported a significantly lower risk of mortality with ACEIs/ARBs in hypertensive COVID-19 patients [Citation116,Citation118]. Zhang et al [Citation116] conducted a large, well designed, retrospective, multi-centre study involving 1128 COVID-19 patients with hypertension across 9 hospitals in Hubei Province, China, including 188 patients taking ACEIs/ARBs. The authors reported that inpatient treatment with ACEIs/ARBs was associated with lower risk of all-cause mortality (HR 0.37; 95% CI, 0.15 to 0.89; p = .03; adjusted HR, 0.29; 0.12–0.69; p = .005) due to COVID-19 compared with those not on ACEIs/ARBs during 28 days of follow-up [Citation116,Citation118]. Thus, these studies combined suggest that ACEI/ARBs are not associated with an increased risk of mortality, but as shown by [Citation116,Citation118] may actually show reduced risk of fatality in some COVID-19 patients.
Several meta-analyses have now been performed on the above observational studies examining the safety of ACEIs/ARBs in COVID-19 patients and these are discussed briefly below.
Meta-analyses of clinical studies of COVID-19 patients receiving ACEIs/ARBs
A few meta-analyses have appeared in the literature [Citation101,Citation119,Citation120] that have analysed the pooled data from the many clinical observational studies discussed above and including those reviewed in . Taken together, these meta-analyses generally confirm the view that COVID-19 patients taking ACEIs/ARBs do not have an increased risk of either a) infection, b) severity of COVID-19 disease, c) risk of admission to hospital or d) mortality as discussed for the individual studies in the preceding sections. Interestingly, some benefit with ACE2-raising ACEIs/ARBs in subsets of COVID-19 patients especially with regards to reduced risk of mortality have been reported e.g. hypertensive patients [Citation118]. Interestingly, in the large sample sized meta-analysis of Zhang X et al. [Citation119] when clinical data sets were grouped by study location, there was also a significantly lower mortality risk in studies from China (OR = 0.65, 95% CI, 0.46 − 0.91, p = 0.013). Although the reasons for this are not entirely clear, if the data is correct, this could reflect differential response amongst ethnicities and/or the involvement of ACE2 polymorphisms.
The published meta-analyses suffer from several flaws related to the methodology employed, presentation of unadjusted effect sizes, and the use of only a small number of studies for pooled estimates. However, in addition to these shortcomings, most of the meta-analyses published to date are flawed mainly due to the inclusion of the now retracted study by Mehra et al [Citation121]. Because this retracted study had a large population sample of COVID-19 patients, its high weightage could likely skew the results towards its results. To investigate this further, we performed a re-analysis of pooled data for the 9 data sets (with data from a total of 1630 COVID-19 patients on ACEIs/ARBs and 11620 not on these drugs) used in the meta-analysis of Zhang X et al. [Citation116]) after removing the retracted study for association with all-cause mortality- as the risk for this outcome was reported [Citation102] to be significantly lower for some COVID-19 patients on ACEIs/ACEIs.
In , we re-calculated the overall OR obtained in the meta-analysis of Zhang X et al. [Citation119] that contained data sets from Mehra et al [Citation121] individually for ACEIs and ARBs. We noted that combined weightage of data sets extracted from the retracted [Citation121] study was quite significant at about 40%, almost double the contribution of any other single study analysed in this data set (see ). With the inclusion this [Citation121] data, Zhang X et al. [Citation119] reported no increased risk of all-cause mortality in COVID-19 patients taking ACEIs/ARBs for multiple indications (overall OR 0.73; 95% CI 0.49, 1.08; calculated using a random-effects model of the DerSi- Monian-Laird model). In contrast when we re-calculated the OR using a robust model [Citation122], we obtained a revised value (OR 0.83; 95% CI 0.53, 1.26, see ) which was more conservative than that reported by [Citation100,102]. After removing the Mehra et al. [Citation121] data (), our re-analysis showed a similar overall OR 0.84; 95% CI (0.59, 1.19). This lack of change was attributed to significant heterogeneity in the two Mehra et al. data sets with results in opposite directions and following their removal, heterogeneity was decreased (see ). Thus, our re-analysed meta-analysis supports the findings that there is no association between ACEIs/ARBs use and increased risk of all-cause mortality. Of interest, the estimates were asymmetric across studies in both re-analyses (Doi plots and LFK (heterogeneity) index values are shown in ) and this asymmetry was towards a protective effect in smaller studies. This suggests that the null result is likely to be very conservative and there may not be a protective effect for these drugs. Although studies discussed above report there may be a drug-induced benefit in reducing risk of mortality for COVID-19 patients who are hypertensive and/or diabetic or from China, our re-analysis does not support the notion that ACEIs/ARBs offer significant reduction in the risk of all-cause mortality when these drugs are used for multiple indications (see ) and not just in hypertension.
Figure 2. Re-analysis of data from the meta-analysis of Zhang X et al [Citation119] on the association between ACEIs/ARBs use, irrespective of clinical indication, and all-cause mortality in COVID-19 patients using a robust model. Forest plots of the meta-analysis with (A) and without (B) Mehra et al [Citation121] data sets. Doi plots with Luis Furuya-Kanamori (LFK) index values are also shown for re-analysed data with (C) and without (D) Mehra et al [Citation121] data sets. Note that LFK index of greater than 2 suggests major asymmetry.
![Figure 2. Re-analysis of data from the meta-analysis of Zhang X et al [Citation119] on the association between ACEIs/ARBs use, irrespective of clinical indication, and all-cause mortality in COVID-19 patients using a robust model. Forest plots of the meta-analysis with (A) and without (B) Mehra et al [Citation121] data sets. Doi plots with Luis Furuya-Kanamori (LFK) index values are also shown for re-analysed data with (C) and without (D) Mehra et al [Citation121] data sets. Note that LFK index of greater than 2 suggests major asymmetry.](/cms/asset/dff20da2-6b4f-420b-a2d2-65b4c250cdf7/idrt_a_1797754_f0002_c.jpg)
The reduced risk of hospitalisation of COVID-19 patients with diabetes (a known risk factor for adverse outcomes in COVID-19 [Citation123]) also taking ACEIs/ARBs was recently reported by de Abajo et al. [Citation114]. The underlying mechanistic rationale for this clinical observation is not known, but animal models of diabetes are associated with high ACE-Ang II activity and/or reduced ACE2-Ang-(1-7) activity including in the lungs and cardiovascular system [Citation21,Citation40,Citation124,Citation125] implying an imbalance in RAAS pathways (see ). Add the impact of reduced ACE2 with SARS-CoV-2 following endocytosis, then the ACE2-raising ACEIs/ARBs drugs most likely shift the RAAS balance towards the more beneficial ACE2-Ang-1-7-MasR pathway. We have shown that activation of this beneficial pathway endogenously or exogenously by administering Ang-(1-7) or its analog prevents or improves the multiple diabetes-induced complications [Citation21,Citation34,Citation37,Citation125–127] including some through inhibiting the transactivation of EGFR/ErbB receptors [Citation128,Citation129]. In models of diabetes and/or hypertension, we have shown that administering Ang-(1-7) prevented endothelial dysfunction, cardiac and renal end-organ damage via mechanisms involving inhibition of NADPH oxidase, NF-kB, TLR-2, IRAK-1, IL-6, EGFR receptors, and renal TGF-beta [Citation32,Citation40,Citation128,Citation129] or activation of NO, catalase and PPAR-gamma pathways [Citation34,Citation130]. It should be noted here, that activation of ACE2-Ang-(1-7) pathway has little or no effects on normal/healthy animals but displays its beneficial anti-pressor effects or cardiovascular benefits only in models of disease e.g. hypertension and/or diabetes [Citation26,Citation37]. Thus, the context of underlying disease will be important when interpreting the role of ACE2-Ang-(-17) in COVID-19 or other pathologies. Also the effect of medication with potential ability to raise ACE2 levels might also be dependent on the underlying pathology- and this may be reflected in the reported benefits observed with RAAS inhibitors in COVID-19 patients with hypertension (reduced mortality) and diabetes (decreased risk of hospitalisation).
The importance of the impact of ACEI/ARB pharmacotherapy and stage of COVID-19 disease was highlighted recently in a study from France. In a retrospective longitudinal cohort study on consecutive patients with newly diagnosed severe COVID-19 [Citation117], chronic use of ACEIs/ARBs in 44 patients was associated with an increased risk of developing acute renal injury (AKI) stage ≥1 (OR, 3.28, 95% CI, 2.17-4.94). Despite the small number of patients, the authors showed that daily dose of ACEIs/ARBs was independently associated with increased risk of elevated kidney markers, such as urea nitrogen >0.52 g/L (OR, 1.26 [95% CI, 1.13–1.41] with an increased risk of +25 to +31% per each 10 mg increment of the lisinopril-dose equivalent. Interestingly, in multivariable multilevel modelling, elevated urea nitrogen was also independently associated with the risk of acute respiratory failure (OR, 3.54, 1.05-11.96) implying that increased urea nitrogen levels associated with ACEIs/ARBs use could predict the development of acute respiratory failure in these COVID-19 patients. This is the first clinical study, albeit with small sample size, providing evidence that chronic ACEIs/ARBs usage may lead to adverse outcomes (i.e. kidney dysfunction and AKI) in COVID-19 patients. The precise mechanisms by which RAAS inhibitors might lead to AKI in severe forms of COVID-19 requires further study.
The safety of other ACE-2-raising medications in COVID-19 patients is less well studied and largely remains unknown thus far. We review the information available for these medications next.
What about the clinical safety of other ACE-2 raising drugs in COVID-19?
In contrast to the many studies with ACEIs/ARBs, very few clinical studies have reported on the association of other ACE2-raising drugs with outcomes of COVID-19 patients such as those listed in . For example, the studies of Reynolds et al. [Citation109] and Mancia et al. [Citation108] also reported that, in addition to ACEIs/ARBs, beta-blockers, calcium-channel blockers (CCBs), and thiazide diuretics, which are also known to raise ACE2 (see ) were not associated with a significantly increased risk of COVID-19 infection or increased severity of disease [Citation108,Citation109]. Despite the higher use of MCRIs amongst hypertensive COVID-19 patients reported by Mancia et al. [Citation108] these drugs were not associated with an increased risk for COVID infections (adjusted OR 0.90 (0.75–1.07). Furthermore, a study by de Abajo et al. [Citation114] showed no increased risk of hospitalisation of COVID-19 patients on beta-blockers, CCBs, MCRIs (aldosterone antagonists) and thiazide diuretics.
A report on the protective effect of statins in older, nursing home COVID-19 patients has appeared recently [Citation131]. In a small retrospective cohort study in two Belgian nursing homes , out of total of 154 residents, 31 COVID-19 positive patients were on statins [Citation131]. In contrast to ACEI/ARBs, these authors found statin intake was associated with the absence of COVID-19 symptoms (unadjusted OR 2.91; CI 1.27-6.71; p = 0.011), which remained statistically significant after adjusting for age, sex, functional status, diabetes mellitus and hypertension (2.65 (1.13-6.68; p = 0.028) . The molecular mechanisms for this potential benefit with statins is unclear but it is known that statins, aside from their lipid-lowering activity, can exert anti-inflammatory and anti-oxidative stress effects that have culminated in a beneficial impact on cardiovascular diseases and lung injury [Citation132–134].
Similar to statins, Vitamin D, a steroid hormone made in the skin by the action of sunlight, may also have anti-inflammatory and immune-regulatory properties [Citation135,Citation136]. In COVID-19, there is currently no reports on prospective studies examining supplementation with vitamin D or its analogs and outcomes in patients but a recent study by Hastie et al. [Citation137] showed that vitamin D deficiency was associated with higher risk of SARS-CoV-2 infection; however the authors claim this was not causal. Based on preclinical and clinical studies suggesting the vitamin D deficiency is associated with increased acute respiratory tract infections [Citation136,Citation138], further clinical trials on whether vitamin D supplementation is beneficial in preventing COVID-19 infections are pending. However, as a precautionary measure, the UK government has proactively issued a recommendation for its population to take Vitamin D supplements during the COVID-19 pandemic [Citation139,Citation140]. Though non-judicial Vitamin D supplementation may run the risk of increased toxicity in non-deficient subjects [Citation135].
Since the majority of clinical studies with ACEIs/ARBs, and some other ACE2-raising drugs, imply either no harm or some benefit in COVID-19 patients, it would be interesting to investigate outcomes for these patients when receiving drugs that decrease ACE2 levels- especially since a recent report suggests that ACE2 mRNA levels might actually be upregulated in nasopharyngeal swab specimens post-SARS-CoV-2 infection [Citation141]. One such drug is insulin, which in a non-obese mouse model of diabetes was shown to reduce serum, urine and renal cortex ACE2 activity [Citation142]. In a human study of 15 hospitalised hyperglycaemic COVID-19 patients receiving insulin infusions, insulin administration was linked with a lower risk of SARS-CoV-2 infection in a risk-adjusted Cox regression analysis compared to those not on insulin therapy. Furthermore, insulin therapy led to a reduction in IL-6 and D-dimer levels [Citation143]. Since a potentially ACE2 lowering drug also showed beneficial effects (at least no harm) implies that the impact of ACE2 modulating drugs is likely to be more complex than simply their net effect on ACE2 levels. It will also be important to understand the full context of their impact based on which tissue(s) ACE2 levels/activity is modulated in humans as well the underlying pathophysiology and/or time-course of the disease. Further, interplay of other signalling pathways and drug-specific effects is likely to be important in determining the overall clinical effects of these drugs in patients and their outcomes. Of the many ACE2-raising drugs, it is likely that those medications, such as the GLP-1 agonist Liraglutide, which raise ACE2 in the lungs would be of particular relevance to COVID-19 pathology. However, no human clinical studies are available for GLP-1 agonists and/or DPP-4 inhibitors.
It is, therefore, becoming clear that further clinical studies are needed to fully assess the many ACE2-rasing drugs, not just RAAS inhibitors, and their resulting outcomes in COVID-19 patients. Some trials are already underway such as that with Ibuprofen (a NSAID) in COVID-19 patients that aims to evaluate the reduction in severity and progression of lung injury with lipid ibuprofen (https://clinicaltrials.gov/ct2/show/NCT04334629).
Concluding remarks
A large body of evidence from clinical observational studies reported thus far, as well as our recalculation of meta-analysis data on all-cause mortality, supports continued use of ACEIs/ARBs in COVID-19 patients. Although these drugs generally appear to be clinically safe, their chronic use in severe COVID-19 patients might increase the risk of acute renal injury [Citation117]. Evidence also exists for the beneficial effects of ACEIs/ARBs in some sub-populations of COVID-19 patients such as in lowering risk of mortality in hypertensive patients or decreasing risk of hospitalisation for COVID-19 patients with diabetes. The possible mechanisms by which ACE2-raising drugs might lead to beneficial outcomes in infected patients need further study but it is thought that increased ACE2 activity would result in a shifting of the balance within the RAAS towards the favourable ACE2-Ang-(1-7)-MasR pathway. This pathway is known to exert anti-inflammatory, anti-fibrotic and anti-oxidative stress signals that could directly benefit the COVID-19 pathology. Indeed, a recent report has shown that the anti-inflammatory agent dexamethasone is useful in the management of COVID-19 [Citation144] and thus, the anti-inflammatory effects of ACE2-Ang-1-7-MasR pathway may account for the increased survival of patients on ACEIs and ARBs in hypertension. If confirmed, this hypothesis would also suggest that activation of ACE2-Ang-(1-7) pathway e.g. via exogenous administration of Ang-(1-7) or ACE2 or its activators may also prove to be therapeutically useful in COVID-19 patients, in a manner similar to that with dexamethasone. However, dexamethasone use is associated with some key disadvantages. Chronic administration of dexamethasone is thought to lead to decreased placental ACE2 levels in an animal model [Citation145] though its effects on altering ACE2 levels in other tissues in vivo is not known. Dexamethasone also has immunosuppressant effects which could potentially increase the risk of infections, increase plasma viral load, decrease immune function and prevent antibody production [Citation146]. Although it is thought that dexamethasone use might be beneficial acutely in attenuating the ‘cytokine storm’, its chronic use might be counter-productive- though this needs further study in the context of COVID-19. If dexamethasone use truly reduces ACE2 levels in key tissues, a potential novel strategy to mitigate this potentially adverse effect might to be to co-administer ACE2, ACE2 activators or (Ang-1-7) together with dexamethasone. Though this strategy might require taking advantage of smart nanotechnology with targeted drug delivery systems that can facilitate tissue-specific delivery of ACE2, ACE2 activators or Ang-(1-7) depending on which tissue ACE2 depletion was noted upon dexamethasone usage in COVID-19 patients. Smart delivery systems now exist that could offer controlled spatial (tissue-specific targeting) and temporal (time-controlled) delivery of peptide and nucleic acid-based therapeutic agents [Citation147–151]. Indeed, pulmonary delivery of Ang-(1-7) with cyclodextrin as nano-carriers has also been reported in animals [Citation39], and an inhalable lung-specific delivery of Ang-(1-7) might offer a potentially novel therapeutic approach in overcoming lung injury- that may arise as a result of SARS-CoV-2-induced decrease in ACE2 levels in airway epithelial cells of COVID-19 patients.
The evidence for the safety of other ACE2-raising drugs, used alone or in combination, is sparse but the majority of the studies discussed herein suggested that these drugs are not associated with adverse outcomes with exception of one study indicating increased risk of AKI in patients receiving ACEI/ARBs in severe COVID-19 disease [Citation117]. Although the majority of clinical studies examining outcomes on COVID-19 patients have reported results for combined use of ACEIs and ARBs, it should be noted that these two drug classes have divergent cardiovascular effects and outcomes in patients [Citation152], and perhaps future studies should aim to report results for ACEIs and ARBs separately. Thus, evidence from the literature reviewed above supports the current guidelines issued by several societies including the recent joint statement of the British Cardiovascular Society together with the British Society for Heart Failure (https://www.britishcardiovascularsociety.org/news/ACEi-or-ARB-and-COVID-19) that COVID-19 patients can continue with their existing ACEI/ARB therapies. Based on a recent report [Citation117], we suggest that patients with severe COVID-19 on ACEIs or ARBS should be monitored for development of renal dysfunction and AKI. However, for definitive clarity there is still a need for large scale, well-conducted randomised clinical trials as well mechanistic studies investigating the safety of the many ACE2-raising drugs that are routinely used in the clinic in COVID-19 patients.
Disclosure statement
No potential conflict of interest was reported by the author(s).
Additional information
Funding
References
- Bourgonje AR, Abdulle AE, Timens W, et al. Angiotensin-converting enzyme 2 (ACE2), SARS-CoV-2 and the pathophysiology of coronavirus disease 2019 (COVID-19). J Pathol. 2020;251(3):228–248.
- Guan WJ, Ni ZY, Hu Y, Clinical characteristics of coronavirus disease 2019 in China. N Engl J Med. 2020;382(18):1708–1720.
- Wu C, Chen X, Cai Y, et al. Risk factors associated with acute respiratory distress syndrome and death in patients with coronavirus disease 2019 pneumonia in Wuhan, China. JAMA Intern Med. 2020a;180(7):934.
- Zhou F, Yu T, Du R, et al. Clinical course and risk factors for mortality of adult inpatients with COVID-19 in Wuhan, China: a retrospective cohort study. Lancet. 2020;395(10229):1054–1062.
- Donoghue M, Hsieh F, Baronas E, et al. A novel angiotensin-converting enzyme-related carboxypeptidase (ACE2) converts angiotensin I to angiotensin 1-9. Circ Res. 2000;87(5):e1–e9.
- Tipnis SR, Hooper NM, Hyde R, et al. A human homolog of angiotensin-converting enzyme. Cloning and functional expression as a captopril-insensitive carboxypeptidase. J Biol Chem. 2000;275(43):33238–33243.
- Xu H, Zhong L, Deng J, et al. High expression of ACE2 receptor of 2019-nCoV on the epithelial cells of oral mucosa. Int J Oral Sci. 2020;12. DOI:10.1038/s41368-020-0074-x
- Hamming I, Timens W, Bulthuis ML, et al. Tissue distribution of ACE2 protein, the functional receptor for SARS coronavirus. A first step in understanding SARS pathogenesis. J Pathol. 2004;203(2):631–637.
- Bunyavanich S, Do A, Vicencio A. Nasal gene expression of angiotensin-converting enzyme 2 in children and adults. JAMA. 2020;323(23):2427.
- Hoffmann M, Kleine-Weber H, Schroeder S, et al. SARS-CoV-2 Cell Entry Depends on ACE2 and TMPRSS2 and Is Blocked by a Clinically Proven Protease Inhibitor. Cell. 2020;181(2):271–280.e8.
- Yan R, Zhang Y, Li Y, et al. Structural basis for the recognition of SARS-CoV-2 by full-length human ACE2. Science. 2020;367(6485):1444–1448.
- Varga Z, Flammer AJ, Steiger P, et al. Endothelial cell infection and endotheliitis in COVID-19. Lancet. 2020;395:1417–1418.
- Kuba K, Imai Y, Rao S, et al. A crucial role of angiotensin converting enzyme 2 (ACE2) in SARS coronavirus-induced lung injury. Nat Med. 2005;11(8):875–879.
- Tai W, He L, Zhang X, et al. Characterization of the receptor-binding domain (RBD) of 2019 novel coronavirus: Implication for development of RBD protein as a viral attachment inhibitor and vaccine. Cell Mol. Immunol. 2020;17:613–620.
- Wrapp D, Wang N, Corbett KS, et al. Cryo-EM structure of the 2019-nCoV spike in the prefusion conformation. Science. 2020;367(6483):1260–1263.
- Lambert DW, Yarski M, Warner FJ, et al. Tumor necrosis factor-alpha convertase (ADAM17) mediates regulated ectodomain shedding of the severe-acute respiratory syndrome-coronavirus (SARS-CoV) receptor, angiotensin-converting enzyme-2 (ACE2). J Biol Chem. 2005;280(34):30113–30119.
- Oudit GY, Pfeffer MA. Plasma angiotensin-converting enzyme 2: novel biomarker in heart failure with implications for COVID-19. Eur Heart J. 2020;41(19):1818–1820.
- South AM, Diz DI, Chappell MC. COVID-19, ACE2, and the cardiovascular consequences. Am J Physiol Heart Circ Physiol. 2020;318(5):H1084–H1090.
- Gheblawi M, Wang K, Viveiros A, et al. Angiotensin converting enzyme 2: SARS-CoV-2 receptor and regulator of the renin-angiotensin system. Circ Res. 2020;126(10):1456–1474.
- Santos RAS, Oudit GY, Verano-Braga T, et al. The renin-angiotensin system: going beyond the classical paradigms. Am J Physiol Heart Circ Physiol. 2019;316(5):H958–H970.
- Yousif MHM, Makki B, El-Hashim AZ, et al. Chronic treatment with Ang-(1-7) reverses abnormal reactivity in the corpus cavernosum and normalizes diabetes-induced changes in the protein levels of ACE, ACE2, ROCK1, ROCK2 and omega-hydroxylase in a rat model of type 1 diabetes. J Diabetes Res. 2014;2014:142154.
- Devaux CA, Rolain JM, Raoult D. ACE2 receptor polymorphism: Susceptibility to SARS-CoV-2, hypertension, multi-organ failure, and COVID-19 disease outcome. J Microbiol Immunol Infect. 2020;53(3):425–435.
- Ferrario CM, Ahmad S, Joyner J, et al. Advances in the renin angiotensin system focus on angiotensin-converting enzyme 2 and angiotensin-(1-7). Adv Pharmacol. 2010;59:197–233.
- Paz Ocaranza M, Riquelme JA, García L, et al. Counter-regulatory renin-angiotensin system in cardiovascular disease. Nat Rev Cardiol. 2020;17(2):116–129.
- Re RN. Role of intracellular angiotensin II. Am J Physiol Heart Circ Physiol. 2018;314(4):H766–H771.
- Ferrario CM. Role of angiotensin II in cardiovascular disease therapeutic implications of more than a century of research. J Renin Angiotensin Aldosterone Syst. 2006;7(1):3–14.
- El-Hashim AZ, Renno WM, Raghupathy R, et al. Angiotensin-(1-7) inhibits allergic inflammation, via the MAS1 receptor, through suppression of ERK1/2- and NF-κB-dependent pathways. Br J Pharmacol. 2012; 166(6):1964–1976.
- Arendse LB, Danser AHJ, Poglitsch M, et al. Novel therapeutic approaches targeting the renin-angiotensin system and associated peptides in hypertension and heart failure. Pharmacol Rev. 2019;71(4):539–570.
- Benter IF, Diz DI, Ferrario CM. Cardiovascular actions of angiotensin(1-7). Peptides. 1993;14(4):679–684.
- Benter IF, Ferrario CM, Morris M, et al. Antihypertensive actions of angiotensin-(1-7) in spontaneously hypertensive rats. Am J Physiol. 1995;269(1 Pt 2):H313–H319.
- Benter IF, Diz DI, Ferrario CM. Pressor and reflex sensitivity is altered in spontaneously hypertensive rats treated with angiotensin-(1-7). Hypertension. 1995;26(6):1138–1144.
- Al-Maghrebi M, Benter IF, Diz DI. Endogenous angiotensin-(1-7) reduces cardiac ischemia-induced dysfunction in diabetic hypertensive rats. Pharmacol Res. 2009;59(4):263–268.
- Benter IF, Yousif MH, Dhaunsi GS, et al. Angiotensin-(1-7) prevents activation of NADPH oxidase and renal vascular dysfunction in diabetic hypertensive rats. Am J Nephrol. 2008;28(1):25–33.
- Dhaunsi GS, Yousif MH, Akhtar S, et al. Angiotensin-(1-7) prevents diabetes-induced attenuation in PPAR-gamma and catalase activities. Eur J Pharmacol. 2010; 638(1–3):108–114.
- Tallant EA, Diz DI, Ferrario CM. State-of-the-art lecture. Antiproliferative actions of angiotensin-(1-7) in vascular smooth muscle. Hypertension. 1999;34(4 Pt 2):950–957. Oct
- Benter IF, Yousif MH, Anim JT, et al. Angiotensin-(1-7) prevents development of severe hypertension and end-organ damage in spontaneously hypertensive rats treated with L-NAME. Am J Physiol Heart Circ Physiol. 2006;290(2):H684–H691.
- Benter IF, Yousif MH, Cojocel C, et al. Angiotensin-(1-7) prevents diabetes-induced cardiovascular dysfunction. Am J Physiol Heart Circ Physiol. 2007;292(1):H666–H672.
- Cao Y, Liu Y, Shang J, et al. Ang-(1-7) treatment attenuates lipopolysaccharide-induced early pulmonary fibrosis. Lab Invest. 2019;99(12):1770–1783.
- Magalhães GS, Gregório JF, Ramos KE, et al. Treatment with inhaled formulation of angiotensin-(1-7) reverses inflammation and pulmonary remodeling in a model of chronic asthma. Immunobiology. 2020;225(3):151957. PMID: 32517880
- Benter IF, Yousif MH, Al-Saleh FM, et al. Angiotensin-(1-7) blockade attenuates captopril- or hydralazine-induced cardiovascular protection in spontaneously hypertensive rats treated with NG-nitro-L-arginine methyl ester. J Cardiovasc Pharmacol. 2011;57(5):559–567.
- Ishiyama Y, Gallagher PE, Averill DB, Tallant EA, et al. Upregulation of angiotensin-converting enzyme 2 after myocardial infarction by blockade of angiotensin II receptors. Hypertension. 2004;43(5):970–976.
- Dijkman R, Jebbink MF, Deijs M, et al. Replication-dependent downregulation of cellular angiotensin-converting enzyme 2 protein expression by human coronavirus NL63. J Gen Virol. 2012;93(Pt 9):1924–1929.
- Li W, Moore MJ, Vasilieva N, et al. Angiotensin-converting enzyme 2 is a functional receptor for the SARS coronavirus. Nature. 2003;426(6965):450–454.
- Ingraham NE, Barakat AG, Reilkoff R, et al. Understanding the renin-angiotensin-aldosterone-SARS-CoV axis: a comprehensive review. Eur Respir J. 2020;56(1):2000912.
- Ye R, Liu Z. ACE2 exhibits protective effects against LPS-induced acute lung injury in mice by inhibiting the LPS-TLR4 pathway. Exp Mol Pathol. 2020;113:104350
- Gu H, Xie Z, Li T, et al. Angiotensin-converting enzyme 2 inhibits lung injury induced by respiratory syncytial virus. Sci Rep. 2016;6:19840
- Imai Y, Kuba K, Rao S, et al. Angiotensin-converting enzyme 2 protects from severe acute lung failure. Nature. 2005;436(7047):112–116.
- Mazucanti CH, Egan JM. SARS-CoV-2 disease severity and diabetes: why the connection and what is to be done? Immun Ageing. 2020; 17:21.
- Ferrario CM, Jessup J, Chappell MC, et al. Effect of angiotensin-converting enzyme inhibition and angiotensin II receptor blockers on cardiac angiotensin-converting enzyme 2. Circulation. 2005a;111(20):2605–2610.
- Jessup JA, Gallagher PE, Averill DB, Brosnihan KB, et al. Effect of angiotensin ii blockade on a new congenic model of hypertension derived from transgenic ren-2 rats. Am J Physiol Heart Circ Physiol. 2006;291(5):H2166–2172.
- Patel VB, Clarke N, Wang Z, et al. Angiotensin ii induced proteolytic cleavage of myocardial ace2 is mediated by tace/adam-17: a positive feedback mechanism in the ras. J Mol Cell Cardiol. 2014;66:167–176.
- Jin HY, Song B, Oudit GY, et al. Ace2 deficiency enhances angiotensin ii-mediated aortic profilin-1 expression, inflammation and peroxynitrite production. PLoS One. 2012;7(6):e38502.
- Soler MJ, Ye M, Wysocki J, et al. Localization of ace2 in the renal vasculature: amplification by angiotensin II type 1 receptor blockade using telmisartan. Am J Physiol Renal Physiol. 2009;296(2):F398–F405.
- Igase M, Strawn WB, Gallagher PE, et al. Angiotensin ii at1 receptors regulate ace2 and angiotensin-(1-7) expression in the aorta of spontaneously hypertensive rats. Am J Physiol Heart Circ Physiol. 2005;289(3):H1013–H1019.
- Furuhashi M, Moniwa N, Mita T, et al. Urinary angiotensin-converting enzyme 2 in hypertensive patients may be increased by olmesartan, an angiotensin II receptor blocker. Am J Hypertens. 2015;28(1):15–21.
- Ocaranza MP, Godoy I, Jalil JE, et al. Enalapril attenuates downregulation of Angiotensin-converting enzyme 2 in the late phase of ventricular dysfunction in myocardial infarcted rat. Hypertension. 2006;48(4):572–578.
- Ferrario CM, Jessup J, Gallagher PE, et al. Effects of renin-angiotensin system blockade on renal angiotensin-(1-7) forming enzymes and receptors. Kidney Int. 2005b;68(5):2189–2196.
- Vuille-dit-Bille RN, Camargo SM, Emmenegger L, et al. Human intestine luminal ACE2 and amino acid transporter expression increased by ACE-inhibitors. Amino Acids. 2015;47(4):693–705.
- Keidar S, Gamliel-Lazarovich A, Kaplan M, et al. Mineralocorticoid receptor blocker increases angiotensin-converting enzyme 2 activity in congestive heart failure patients. Circ Res. 2005;97(9):946–953.
- Tikoo K, Patel G, Kumar S, et al. Tissue specific up regulation of ACE2 in rabbit model of atherosclerosis by atorvastatin: role of epigenetic histone modifications. Biochem Pharmacol. 2015;93(3):343–351.
- Shin YH, Min JJ, Lee JH, et al. The effect of fluvastatin on cardiac fibrosis and angiotensin-converting enzyme-2 expression in glucose-controlled diabetic rat hearts. Heart Vessels. 2017;32(5):618–627.
- Sánchez-Aguilar M, Ibarra-Lara L, Del Valle-Mondragón L, et al. Rosiglitazone, a ligand to PPARγ, improves blood pressure and vascular function through renin-angiotensin system regulation. PPAR Res. 2019; 2019:1371758.
- Zhang W, Xu YZ, Liu B, et al. Pioglitazone upregulates angiotensin converting enzyme 2 expression in insulin-sensitive tissues in rats with high-fat diet-induced nonalcoholic steatohepatitis. ScientificWorldJournal. 2014; 2014:603409.
- Li Y-H, Wang Q-X, Zhou J-W, et al. Effects of rosuvastatin on expression of angiotensin-converting enzyme 2 after vascular balloon injury in rats. J Geriatr Cardiol. 2013;10(2):151–158.
- Romaní-Pérez M, Outeiriño-Iglesias V, Moya CM, et al. Activation of the GLP-1 receptor by liraglutide Increases ACE2 expression, reversing right ventricle hypertrophy, and improving the production of SP-A and SP-B in the lungs of type 1 diabetes rats. Endocrinology. 2015;156(10):3559–3569.
- Fandiño J, Vaz AA, Toba L, et al. Liraglutide enhances the activity of the ACE-2/Ang(1-7)/Mas receptor pathway in lungs of male pups from food-restricted mothers and prevents the reduction of SP-A. Int J Endocrinol. 2018;2018:6920620.
- Zhang L-H, Pang X-F, Bai F, et al. Preservation of glucagon-like peptide-1 level attenuates angiotensin II-induced tissue fibrosis by altering AT1/AT 2 receptor expression and angiotensin-converting enzyme 2 activity in rat heart. Cardiovasc Drugs Ther. 2015;29(3):243–255.
- Jessup JA, Brosnihan KB, Gallagher PE, et al. Differential effect of low dose thiazides on the renin angiotensin system in genetically hypertensive and normotensive rats. J Am Soc Hypertens. 2008;2(2):106–115.
- Varagic J, Ahmad S, Voncannon JL, et al. Nebivolol reduces cardiac angiotensin II, associated oxidative stress and fibrosis but not arterial pressure in salt-loaded spontaneously hypertensive rats. J Hypertens. 2012;30(9):1766–1774.
- Abdel-Fattah MM, Messiha BAS, Mansour AM. Modulation of brain ACE and ACE2 may be a promising protective strategy against cerebral ischemia/reperfusion injury: an experimental trial in rats. Naunyn Schmiedebergs Arch Pharmacol. 2018;391(9):1003–1020.
- Awwad ZM, El-Ganainy SO, ElMallah AI, et al. Assessment of Pregabalin-Induced Cardiotoxicity in Rats: Mechanistic Role of Angiotensin 1-7. Cardiovasc Toxicol. 2020;20(3):301–311.
- Qiao W, Wang C, Chen B, et al. Ibuprofen attenuates cardiac fibrosis in streptozotocin-induced diabetic rats. Cardiology. 2015;131(2):97–106.
- Bukowska A, Spiller L, Wolke C, et al. Protective regulation of the ACE2/ACE gene expression by estrogen in human atrial tissue from elderly men. Exp Biol Med (Maywood)). 2017;242(14):1412–1423.
- Tanno T, Tomita H, Narita I, et al. Olmesartan Inhibits Cardiac Hypertrophy in Mice Overexpressing Renin Independently of Blood Pressure: Its Beneficial Effects on ACE2/Ang(1-7)/Mas Axis and NADPH Oxidase Expression. J Cardiovasc Pharmacol. 2016;67(6):503–509.
- Lin M, Gao P, Zhao T, et al. Calcitriol regulates angiotensin-converting enzyme and angiotensin converting-enzyme 2 in diabetic kidney disease. Mol Biol Rep. 2016;43(5):397–406.
- Wang X, Ye Y, Gong H, et al. The effects of different angiotensin II type 1 receptor blockers on the regulation of the ACE-AngII-AT1 and ACE2-Ang(1-7)-Mas axes in pressure overload-induced cardiac remodeling in male mice. J Mol Cell Cardiol. 2016;97:180–190.
- Wu C, Ye D, Mullick AE, et al. Effects of renin-angiotensin inhibition on ACE2 and TMPRSS2 expression: insights into COVID-19. bioRxiv. 2020.
- Chodavarapu H, Grobe N, Somineni HK, et al. Rosiglitazone treatment of type 2 diabetic db/db mice attenuates urinary albumin and angiotensin converting enzyme 2 excretion. PLoS One. 2013;8(4):e62833.
- Reimann F, Habib AM, Tolhurst G, et al. Glucose sensing in L cells: a primary cell study. Cell Metab. 2008;8(6):532–539.
- Habib AM, Richards P, Cairns LS, et al. Overlap of endocrine hormone expression in the mouse intestine revealed by transcriptional profiling and flow cytometry. Endocrinology. 2012;153(7):3054–3065.
- Habib AM, Richards P, Rogers GJ, et al. Co-localisation and secretion of glucagon-like peptide 1 and peptide YY from primary cultured human L cells. Diabetologia. 2013;56(6):1413–1416.
- Gribble FM, Reimann F. Enteroendocrine cells: chemosensors in the intestinal epithelium. Annu Rev Physiol. 2016;78:277–299.
- Gribble FM, Reimann F. Function and mechanisms of enteroendocrine cells and gut hormones in metabolism. Nat Rev Endocrinol. 2019;15(4):226–237.
- Pais R, Rievaj J, Larraufie P, et al. Angiotensin II Type 1 receptor-dependent GLP-1 and PYY secretion in mice and humans. Endocrinology. 2016;157(10):3821–3831.
- McCormack FX, Whitsett JA. The pulmonary collectins, SP-A and SP-D, orchestrate innate immunity in the lung. J Clin Invest. 2002;109(6):707–712.
- Lely AT, Hamming I, van Goor H, et al. Renal ACE2 expression in human kidney disease. J Pathol. 2004;204(5):587–593.
- Epelman S, Shrestha K, Troughton RW, et al. Soluble angiotensin-converting enzyme 2 in human heart failure: relation with myocardial function and clinical outcomes. J Card Fail. 2009;15(7):565–571.
- Walters TE, Kalman JM, Patel SK, et al. Angiotensin converting enzyme 2 activity and human atrial fibrillation: increased plasma angiotensin converting enzyme 2 activity is associated with atrial fibrillation and more advanced left atrial structural remodelling. Europace. 2017;19:1280–1287.
- Ramchand J, Patel SK, Kearney LG, et al. Plasma ACE2 activity predicts mortality in aortic stenosis and is associated with severe myocardial fibrosis. JACC Cardiovasc Imaging. 2020;13(3):655–664.
- Ramchand J, Patel SK, Srivastava PM, et al. Elevated plasma angiotensin converting enzyme 2 activity is an independent predictor of major adverse cardiac events in patients with obstructive coronary artery disease. PLoS One. 2018;13(6):e0198144.
- Luque M, Martin P, Martell N, et al. Effects of captopril related to increased levels of prostacyclin and angiotensin-(1-7) in essential hypertension. J Hypertens. 1996;14(6):799–805.
- Campbell DJ, Zeitz CJ, Esler MD, et al. Evidence against a major role for angiotensin converting enzyme-related carboxypeptidase (ACE2) in angiotensin peptide metabolism in the human coronary circulation. J Hypertens. 2004;22:1971–1976.
- Vaduganathan M, Vardeny O, Michel T, et al. Renin-angiotensin-aldosterone system inhibitors in patients with Covid-19. N Engl J Med. 2020;382(17):1653–1659.
- Bader M, Ganten D. Update on tissue renin-angiotensin systems. J Mol Med. 2008;86(6):615–621.
- Fang L, Karakiulakis G, Roth M. Are patients with hypertension and diabetes mellitus at increased risk for COVID-19 infection? Lancet Respir Med. 2020;8(4):e21.
- Sommerstein R, Kochen MM, Messerli FH, GräNi C. Coronavirus Disease 2019 (COVID-19): do angiotensin-converting enzyme inhibitors/angiotensin receptor blockers have a biphasic effect? J Am Heart Assoc. 2020;9(7):e016509.
- Hanff TC, Harhay MO, Brown TS, et al. Is there an association between COVID-19 mortality and the renin-angiotensin system-a call for epidemiologic investigations. Clin Infect Dis. 2020;71(15):870–4.
- Sommerstein R. Re: Preventing a COVID-19 pandemic: ACE inhibitors as a potential risk factor for fatal COVID-19. BMJ. 2020;368:m810. DOI:10.1136/bmj.m810
- Wu Z, McGoogan JM. Characte ristics of and important lessons from the coronavirus disease 2019 (COVID-19) outbreak in China: summary of a report of 72 314 cases from the Chinese Center for Disease Control and Prevention. JAMA. 2020;323(13):1239.
- Huang Z, Cao J, Yao Y, et al. The effect of RAS blockers on the clinical characteristics of COVID-19 patients with hypertension. Ann Transl Med. 2020;8(7):430.
- Guo T, Fan Y, Chen M, et al. Cardiovascular implications of fatal outcomes of patients with coronavirus disease 2019 (COVID-19). JAMA Cardiol. 2020;5(7):811.
- Gurwitz D. Angiotensin receptor blockers as tentative SARS-CoV-2 therapeutics. Drug Dev Res. 2020. DOI:ddr.21656/ddr.21656. [Online ahead of print].
- Patel AB, Verma A. COVID-19 and angiotensin-converting enzyme inhibitors and angiotensin receptor blockers: what is the evidence? JAMA. 2020. DOI:10.1001/jama.2020.4812. [Online ahead of print].
- Mourad JJ, Levy BI. Interaction between RAAS inhibitors and ACE2 in the context of COVID-19. Nat Rev Cardiol. 2020;17(5):313.
- Monteil V, Kwon H, Prado P, et al. Inhibition of SARS-CoV-2 infections in engineered human tissues using clinical-grade soluble human ACE2. Cell. 2020;181(4):905.e7–913.e7.
- Zhou P, Yang XL, Wang XG, et al. A pneumonia outbreak associated with a new coronavirus of probable bat origin. Nature. 2020;579(7798):270–273.
- Liu Y, Huang F, Xu J, et al. Anti-hypertensive angiotensin II receptor blockers associated to mitigation of disease severity in elderly COVID-19 patients. 2020 [Posted 2020 Mar 27].
- Mancia G, Rea F, Ludergnani M, et al. Renin-Angiotensin-Aldosterone System Blockers and the Risk of Covid-19. N Engl J Med. 2020;382(25):2431–2440.
- Reynolds HR, Adhikari S, Pulgarin C, et al. Renin-Angiotensin-Aldosterone System Inhibitors and Risk of Covid-19. N Engl J Med. 2020;18382(25):2441–2448.
- Yang G, Tan Z, Zhou L, et al. Effects of ARBs and ACEIs on virus infection, inflammatory status and clinical outcomes In COVID-19 patients with hypertension: a single center retrospective study. Hypertension. 2020;76(1):51–58.
- Feng Z, Li J, Yao S, et al. The use of adjuvant therapy in preventing progression to severe pneumonia in patients with coronavirus disease 2019: a multicenter data analysis. 2020. DOI:10.1101/2020.04.08.20057539
- Li J, Wang X, Chen J, et al. Association of renin-angiotensin system inhibitors with severity or risk of death in patients with hypertension hospitalized for coronavirus disease 2019 (COVID-19) Infection in Wuhan, China. JAMA Cardiol. 2020;5(7):825.
- Meng J, Xiao G, Zhang J, et al. Renin-angiotensin system inhibitors improve the clinical outcomes of COVID-19 patients with hypertension. Emerg Microbes Infect. 2020;9(1):757–760.
- de Abajo FJ, Rodríguez-Martín S, Lerma V, MED-ACE2-COVID19 study group, et al. Use of renin-angiotensin-aldosterone system inhibitors and risk of COVID-19 requiring admission to hospital: a case-population study. Lancet. 2020;395(10238):1705–1714.
- Tedeschi S, Giannella M, Bartoletti M, et al. Clinical impact of renin-angiotensin system inhibitors on in-hospital mortality of patients with hypertension hospitalized for Coronavirus Disease 2019. Clin Infect Dis. 2020;71(15):899–901.
- Zhang P, Zhu L, Cai J, t al. Association of inpatient use of angiotensin-converting enzyme inhibitors and angiotensin II receptor blockers with mortality among patients with hypertension hospitalized with COVID-19. Circ Res. 2020b;126(12):1671–1681.
- Merc Urmes I, et al. Long-term ACE Inhibitor/ARB use is associated with severe renal dysfunction and acute kidney injury in patients with severe COVID-19: results from a referral center cohort in the North East of France. Clin Infect Dis. 2020. DOI:10.1093/cid/ciaa677. [Online ahead of print].
- Zhang JJ, Dong X, Cao YY, et al. Clinical characteristics of 140 patients infected with SARS-CoV-2 in Wuhan, China. Allergy. 2020a;75(7):1730–1741.
- Zhang X, Yu J, Pan LY, et al. ACEI/ARB use and risk of infection or severity or mortality of COVID-19: A systematic review and meta-analysis. Pharmacol Res. 2020;158:104927. DOI: 10.1016/j.phrs.2020.104927. PMID: 32422341.
- Pirola CJ, Sookoian S. Estimation of Renin-Angiotensin-Aldosterone-System (RAAS)-Inhibitor effect on COVID-19 outcome: A Meta-analysis. J Infect. 2020;28;81(2):276–81.
- Mehra MR, Desai SS, Kuy S, et al. Cardiovascular disease, drug therapy, and mortality in Covid-19. N Engl J Med. 2020;382(26):2582–2582.
- Doi SAR, Barendregt JJ, Khan S, et al. Advances in the meta-analysis of heterogeneous clinical trials I: the inverse variance heterogeneity model. Contemp Clin Trials. 2015;45(Pt A):130–138.
- Guo W, Li M, Dong Y, et al. Diabetes as a risk factor for the progression and prognosis of COVID-19. Diabetes Metab Res Rev. 2020:e3319.DOI:10.1002/dmrr.3319. [Online ahead of print].
- Roca-Ho H, Riera M, Palau V, et al. Characterization of ACE and ACE2 expression within different organs of the NOD mouse. IJMS. 2017;18(3):563.
- Yousif MHM, Dhaunsi GS, Makki BM, et al. Characterization of Angiotensin-(1-7) effects on the cardiovascular system in an experimental model of type-1 diabetes. Pharmacol Res. 2012;66(3):269–275.
- Kilarkaje N, Yousif MH, El-Hashim AZ, et al. Role of angiotensin II and angiotensin-(1-7) in diabetes-induced oxidative DNA damage in the corpus cavernosum. Fertil Steril. 2013;100(1):226–233.
- Dhaunsi GS, Yousif MH, Makki B, et al. Angiotensin-(1-7) downregulates diabetes-induced cGMP phosphodiesterase activation in rat corpus cavernosum. Biomed Res Int. 2017;2017:50849617.
- Akhtar S, Yousif MH, Dhaunsi GS, et al. Angiotensin-(1-7) inhibits epidermal growth factor receptor transactivation via a Mas receptor-dependent pathway. Br J Pharmacol. 2012;165(5):1390–1400.
- Akhtar S, Chandrasekhar B, Attur S, et al. Transactivation of ErbB family of receptor tyrosine kinases is inhibited by angiotensin-(1-7) via its mas receptor. PLoS One. 2015;10(11):e0141657.
- Abwainy A, Babiker FA, Akhtar S, et al. Endogenous Angiotensin-(1-7)/MAS receptor/NO is a novel pathway involved in the cardioprotective effects of pacing postconditioning. Am J Physiol Heart Circ Physiol. 2016;310(1):H104–12.
- De Spiegeleer A, Bronselaer A, Teo JT, et al. 2020. The effects of ARBs, ACEIs and statins on clinical outcomes of 2 COVID-19 infection among nursing home residents. J Am Med Dir Assoc. 2020. DOI:10.1016/j.jamda.2020.06.018.
- Castiglione V, Chiriacò M, Emdin M, et al. Statin therapy in COVID-19 infection. Eur Heart J Cardiovasc Pharmacother. 2020;6(4):258–259.
- Jacobson JR, Barnard JW, Grigoryev DN, et al. Simvastatin attenuates vascular leak and inflammation in murine inflammatory lung injury. Am J Physiol Lung Cell Mol Physiol. 2005;288(6):L1026–L1032.
- Shyamsundar M, McKeown ST, O'Kane CM, et al. Simvastatin decreases lipopolysaccharide-induced pulmonary inflammation in healthy volunteers. Am J Respir Crit Care Med. 2009;179(12):1107–1114.
- Habib AM, Nagi K, Thillaiappan NB, et al. Vitamin D and Its Potential Interplay With Pain Signaling Pathways. Front Immunol. 2020;11:820
- Zisi D, Challa A, Makis A. The association between vitamin D status and infectious diseases of the respiratory system in infancy and childhood. Hormones (Athens)). 2019;18(4):353–363.
- Hastie CE, Mackay DF, Ho F, et al. Vitamin D concentrations and COVID-19 infection in UK Biobank. Diabetes Metab Syndr. 2020;14(4):561–565.
- Martineau AR, Jolliffe DA, Hooper RL, et al. Vitamin D supplementation to prevent acute respiratory tract infections: systematic review and meta-analysis of individual participant data. BMJ (Clinical Research ed.). 2017;356:i6583.
- SACN 2020. Rapid review: Vitamin D and acute respiratory tract infections. Published June, 2020 [Accessed 2020 Jul 14]. https://app.box.com/s/g0ldpth1upfd7fw763ew3aqa3c0pyvky.
- NICE. COVID-19 rapid evidence summary: vitamin D for COVID-19. 2020 [Published 2020 Jun 29; Accessed 2020 June 14]. https://www.nice.org.uk/advice/es28/resources/covid19-rapid-evidence-summary-vitamin-d-for-covid19-pdf-1158182526661.
- Zhuang MW, Cheng Y, Zhang J, et al. Increasing host cellular receptor-angiotensin-converting enzyme 2 (ACE2) expression by coronavirus may facilitate 2019-nCoV (or SARS-CoV-2) Infection. J Med Virol. 2020. DOI:10.1002/jmv.26139. [Online ahead of print].
- Riera M, Marquez E, Clotet S, et al. Effect of insulin on ACE2 activity and kidney function in the non-obese diabetic mouse. PloS One. 2014;9(1):e84683.
- Sardu C, D’Onofrio N, Balestrieri ML, et al. Outcomes in patients with hyperglycemia affected by COVID-19: can we do more on glycemic control. Dia Care. 2020;43(7):1408–1415.
- RECOVERY Collaborative Group. Effect of Dexamethasone in Hospitalized Patients with COVID-19 – Preliminary Report. medRxiv preprint. 2020.
- Ghadhanfar E, Alsalem A, Al-Kandari S, et al. The Role of ACE2, angiotensin-(1-7) and Mas1 receptor axis in glucocorticoid-induced intrauterine growth restriction. Reprod Biol Endocrinol. 2017;15(1):97.
- Theoharides TC, Conti P. Dexamethasone for COVID-19? Not so fast. J Biol Regul Homeost Agents. 2020;34(3). DOI:10.23812/20-EDITORIAL_1-5. [Online ahead of print].
- Riley RS, June CH, Langer R, et al. Delivery technologies for cancer immunotherapy. Nat Rev Drug Discov. 2019;18(3):175–196.
- Tibbitt MW, Dahlman JE, Langer R. Emerging frontiers in drug delivery. J Am Chem Soc. 2016;138(3):704–717.
- Juliano RL. The delivery of therapeutic oligonucleotides. Nucleic Acids Res. 2016;44(14):6518–6548.
- Ruoslahti E. Tumor penetrating peptides for improved drug delivery. Adv Drug Deliv Rev. 2017; 110–111:3–12.
- Akhtar S, Benter IF. Nonviral delivery of synthetic siRNAs in vivo. J Clin Invest. 2007;117(12):3623–3632.
- Strauss MH, Hall AS. The divergent cardiovascular effects of angiotensin-converting enzyme inhibitors and angiotensin II type 1 receptor blockers in adult patients with type 2 diabetes mellitus. Can J Diabetes. 2018;42(2):124–129.