Abstract
Context: Baicalin has many pharmacological activities, including protective function against myocardial ischemia by antioxidant effects and free radical scavenging activity. However, its rapid elimination half-life in plasma and poor water solubility limits its clinical efficacy.
Objective: Novel baicalin-loaded PEGylated nanostructured lipid carriers (BN-PEG-NLC) were developed to improve bioavailability of BN, to prolong retention time in vivo and to enhance its protective effect.
Methods: In this study, BN-PEG-NLC were prepared by the emulsion-evaporation and low temperature-solidification method using a mixture of glycerol monostearate and polyethylene glycol monostearate as solid lipids, and oleic acid as the liquid lipid. The physicochemical properties of NLC were characterized. The pharmacokinetic and pharmacodynamic behaviors of BN-PEG-NLC or BN-NLC were evaluated in acute MI rats.
Results and discussion: The particle size, zeta potential, and entrapment efficiency for BN-PEG-NLC were observed as 83.9 nm, −32.1 mV, and 83.5%, respectively. The release profiles of BN from both BN-PEG-NLC and BN-NLC were fitted to the Ritger–Peppas modal, which presented burst release initially and prolonged release afterwards. Pharmacokinetics results indicated that BN-PEG-NLC exhibited a 7.2-fold increase in AUC in comparison to BN solution, while a 3-fold increase in comparison to BN-NLC. Biodistribution results revealed that BN-PEG-NLC exhibited higher heart drug concentration compared with BN-NLC as well as BN solution. In the present study, BN-PEG-NLC significantly ameliorated infarct size.
Conclusion: The results of the present study imply that PEG-NLC could be the biocompatible carriers for heart-targeted drug delivery to improve myocardial ischemia.
Introduction
Cardiovascular diseases (CVD), accounting for about 30% of deaths worldwide, collectively comprised of disorders affecting the heart and blood vessels as well as their associated adverse conditions (Behera et al., Citation2015). Atherosclerotic CVD, such as acute myocardial infarction (MI) and stroke, continue to be major causes of death and disability worldwide (Go et al., Citation2014). In patients with ST-segment elevation acute MI, early reperfusion therapy is a standard strategy to limit MI size. However, MI causes a significant decrease in blood flow and significant challenges remain in designing of efficient delivery systems for myocardial therapy (Mueller et al., Citation1981). While MI also leads to the enhanced permeability and retention (EPR) effect, which is the primary mechanism of accumulation of nanocarriers in the infarct areas (Lukyanov et al., Citation2004; Yao et al., Citation2015).
Nanotechnology-based drug delivery systems (nano-DDS) may accumulate in ischemia-reperfusion (IR) myocardium. Thus, nano-DDS may be feasible for myocardial IR injury targeting ischemic myocardium and inflammatory (Matoba & Egashira, Citation2014). Recent advances in nano-DDS for MI include liposomes, PEGylated liposomes, PEG-PE micelles, mono-PEGylated conjugates, PLGA nanoparticles, silica nanoparticles, core-shell hybrid liposomal vesicles, lipid nanoparticles, etc. (Galagudza et al., Citation2010; Galagudza et al., Citation2012; Zhang et al., Citation2012; Almer et al., Citation2013; Simón-Yarza et al., Citation2013). These nano-DDS successfully delivered drugs to the ischemic myocardium and reduced MI size. Nanostructured lipid carriers (NLC) are composed of binary mixture of solid lipid and a spatially different liquid lipid as the carrier (Joshi & Müller, Citation2009). Zhang et al. (Citation2010) have studied the ability of NLC to improve the delivery of Tanshinone II A (a lipophilic cardiovascular drug) by in vitro studies. Li et al. (Citation2013) have tested the pharmacokinetics profiles of breviscapine NLC (Bre-NLC), and found that after intravenous administration in rats, the Bre-NLCs exhibited a 32 times increase in the AUC0–t and a 12 times increase in t1/2 as compared to the commercially available breviscapine solution (a cardiovascular drug). Therefore, NLC were chosen to carry a traditional Chinese medicine for the treatment of CVD.
Baicalin (BN), one of the major bioactive flavone glucuronides isolated from the dried roots of Scutellaria baicalensis Georigi. It has been reported that BN possess a variety of pharmacological properties, including antioxidant properties and free radical scavenging activity, anti-tumor, anti-thrombotic, anti-apoptotic properties, etc. (Liu X et al., Citation2013). Recent researches have been proven that BN attenuated acute MI via several mechanisms: inhibiting mitochondrial damage-mediated apoptosis, mediating the mitogen-activated protein kinase pathway, antioxidant and anti-apoptotic properties (Wang et al., Citation2013; Kong et al., Citation2014). However, due to the glycosyl group on the ring, BN is extensive first-pass metabolism, low bioavailability, short half-life, poor water solubility, etc. (Wang et al., Citation2015). In order to enhance its clinical efficacy, we designed long-circulating NLC to deliver BN the regions of MI.
In this study, we designed a novel baicalin-loaded PEGylated nanostructured lipid carriers (BN-PEG-NLC) to improve the pharmacokinetics profiles of BN, including a prolonged circulation time and an increase in AUC. BN-PEG-NLC was prepared by the emulsion-evaporation and low temperature-solidification method. The physicochemical parameters such as particle size, polydispersity index (PDI), zeta potential, and entrapment efficiency (EE), and so on were measured. In vitro release and in vitro stability in the fresh plasma were analyzed. In vivo pharmacokinetics, biodistribution, and infarct size were assessed in rats after intravenous injection.
Materials and methods
Materials
BN, oleic acid (OA), Tween 80, and 2,3,5-triphenyltetrazolium chloride, rutin were obtained from Sigma-Aldrich (St. Louis, MO). Glycerol monostearate (GMS) was purchased from Aladdin Industrial Corporation (Shanghai, China). Polyethylene glycol monostearate (PEG-SA, the polymerization degree of ethylene glycol is 55) was purchased from Tokyo Kasei Kogyo Co., Ltd. (Japan). Soybean lecithin (SL) in injection grade was purchased from Shanghai Taiwei Pharmaceutical Co., Ltd. (Shanghai, China).
Animals
Male Sprague-Dawley (SD) rats weighing between 240 and 260 g were obtained from the Center of Experimental Animals of Shandong Province (China). Animals were housed at a constant room temperature, exposed to a natural photoperiod (light/dark cycle of 12:12 h), and were allowed access to standard rodent chow and water ad libitum. All animal experiments were performed in accordance with the requirements of the National Act on the Use of Experimental Animals (People’s Republic of China).
Preparation of BN-PEG-NLC and BN-NLC
The BN-PEG-NLC () and BN-NLC were prepared by the emulsion-evaporation and low temperature-solidification method (Luan et al., Citation2014; Liu Z et al., Citation2015; Lin et al., Citation2016). The lipid phase, containing GMS, PEG-SA, OA, and SL (2:2:2:1, w/w/w) and BN, was dissolved in 5 mL ethanol. Then, the lipid mixture was kept at 75 °C and 400 rpm, and dispersed into 10 mL of aqueous solution containing 0.5% Tween 80 (heated at 75 °C) under continuous stirring at 400 rpm in a water bath for 2.5 h. After the organic solvent was removed, the hot nanoemulsion was dispersed rapidly into 20 mL of cold distilled water (0–2 °C) with stirring at 800 rpm for 2 h.
Figure 1. A sketch of the preparation of BN-PEG-NLC. BN: baicalin; PEG: polyethylene glycol; NLC: nanostructured lipid carriers.
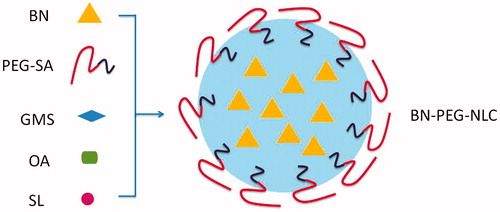
BN-NLC were prepared by the same technique, using GMS instead of PEG-SA.
Blank NLC (NLC) were prepared by the same method without the use of BN, and using GMS instead of PEG-SA.
Characterization of NLC
Particle size and zeta potential analysis
Mean particle size and zeta potential of BN-PEG-NLC and BN-NLC were measured by Malvern Zetasizer Nano® Instruments (Malvern, UK). All samples were diluted with double-distilled water to have a suitable concentration for examination and each sample was determined in triplicate.
Entrapment efficiency and drug loading
The EE and drug loading (DL) were determined by the method of centrifugation ultrafiltration. NLC dispersions (1 mL) were centrifuged for 30 min at 3500 rpm at 4 °C. The filtrate was withdrawn as Sample A to determine the concentration of the free drug. NLC dispersions (1 mL) were disrupted with 5 mL of methanol, and then centrifuged at 15 000 rpm for 10 min. The supernatant was withdrawn as Sample B to determinate the total content of BN drug. Free drug or total drug content was determined by Waters 2695 HPLC (Waters Corporation, Milford, MA) (Zhang et al., Citation2006). Chromatographic separations were carried out using the Intertsil® ODS-3V (25 cm × 4.6 mm, 5 μm). Mobile phase was consisted of a mixture of acetonitrile, water and H3PO4 (45:55:0.2, v/v/v). Flow rate was kept at 0.5 mL/min and system was maintained at 35 °C, the detection was carried out at λ = 278 nm. Injection volume was 10 μL.
The EE and DL could be calculated by the following equations:
Where WA is the amount of non-encapsulated drug, WB is the amount of total drug, and Wlipid is the amount of lipid added. Each sample was measured in triplicate.
In vitro release kinetics
In vitro release kinetics of BN-PEG-NLC, BN-NLC, and free BN solution were performed over 48 h using the dialysis bag method, with 50 mL PBS (pH6.8) as the release medium (Wei et al., Citation2014; Qu et al., Citation2015). 5 mL NLC formulation or BN solution was enclosed in dialysis bag and incubated with release medium at a stirring rate of 100 rpm and at 37 ± 0.5 °C. At predetermined time points, samples (1 mL) were withdrawn from the release medium and analyzed by the HPLC method mentioned above. After sampling, the release medium was replaced by fresh PBS immediately.
Experimental model of acute MI
Acute MI model was established by the permanent ligation of left coronary artery method (Chen et al., Citation2011; Jin et al., Citation2015; Shi et al., Citation2016). Briefly, rats were anesthetized with 10% chloraldurate solution at a dose of 3.8 mL/kg via intraperitoneal (IP) injection, incubated, and ventilated using a respirator pump. Then, the heart was exteriorized, and ligated from the pulmonary conus to the left atrial appendage (2–3 mm). The heart returned to its normal position, and the left thorax closed immediately. Time began when the left coronary artery was ligated completely. Sham-operated rats were subjected to the same surgical procedure without coronary artery ligation (CAL). Electrocardiography (ECG) was continuously monitored during the procedure. ST segment elevation could be observed after successful induction of acute MI.
In vivo pharmacokinetic evaluation
Rats were randomly divided into five groups (n = 6 for each group): the sham group, the AMI group (AMI rats receiving normal saline), the BN group, the BN-NLC group, and the BN-PEG-NLC group. The rats of BN, BN-NLC, and BN-PEG-NLC groups were treated with the respective drugs at a dose of 10 mg/kg via intravenous injection. Blood samples were obtained prior to i.v. (0 h) and at 15 min, 30 min, 45 min, 1 h, 2 h, 4 h, 6 h, 8 h, 12 h, 16 h, 24 h, and 36 h after i.v. Samples were separated immediately by centrifugation at 4000 rpm for 5 min and stored at −20 °C for further analysis. The concentrations of BN in rat plasma were determined by the HPLC method mentioned above.
Biodistribution evaluation
Rats were randomly divided into three groups (n = 6 for each group): the BN group, the BN-NLC group, and the BN-PEG-NLC group. At 15 min after i.v., rats were sacrificed. Blood samples were collected, and the heart, liver, spleen, lung, kidney, and brain of rats were removed, washed, weighed, and homogenized. All of the samples were stored at −20 °C for further analysis.
BN concentrations in plasma and tissues were determined by HPLC assay. Plasma or tissue homogenates (500 μL) were mixed with 50 μL of rutin (80.0 μg/mL) as the internal standard and 250 μL of ammonium acetate buffer (pH 3.5) (Zhang et al., Citation2015; Feng et al., Citation2016; Wei et al., Citation2016). The mixture was vortexed with 2 mL of acetonitrile and centrifuged at 8000 rpm for 10 min to precipitate the proteins. The supernatant was collected and evaporated under a stream of nitrogen at 40 °C. The dry sample was dissolved in 100 μL of mobile phase, and 20 μL of the supernatant was injected into the HPLC system as described above.
Effects of BN-PEG-NLC, BN-NLC, and free BN on infarct size
Rats were randomly divided into five groups as the section of “In vivo pharmacokinetic evaluation”. Rats were sacrificed at 36 h after i.v. The hearts were excised and sliced into 2 mm thick sections parallel to the atrioventricular groove. Infarct size of heart sample was measured with 1% 2,3,5-triphenyltetrazolium chloride for 30 min in the dark and photographed for identification of infarct area (Galagudza et al., Citation2012). The normal myocardium areas were stained brick red, and the infarct areas were unstained. The infarct size could be calculated by the following equation:
Where SIS is the size of the infarct area, Swhole is the whole size of the left ventricle.
Statistical analysis
Results are presented as means ± SD. Statistical comparison was performed with two-tailed Student’s t-tests or one-way or two-way ANOVA. A probability value of p < 0.05 was considered statistically significant.
Results
Characterization of NLC
The mean particle size, polydispersity index (PDI), zeta potential, EE, and DL of NLC are represented in . Particle sizes of NLC were in the colloidal size range which ranged between 80 nm and 90 nm. The mean particle sizes of BN-PEG-NLC, BN-NLC, and NLC were 83.9 ± 1.6 nm, 80.8 ± 3.2 nm, and 82.6 ± 2.3 nm, respectively. Narrow PDI values (<0.3) were obtained.
Table 1. Characterization of NLC.
Zeta potential of NLC ranged between −30 and −40 mV. No significant differences were observed in zeta potential of different formulations.
The EE of BN-PEG-NLC or BN-NLC was 83.5 ± 1.2% or 90.6 ± 2.7%, respectively. The DL of BN-PEG-NLC or BN-NLC was 6.0 ± 0.08% or 6.5 ± 0.12%, respectively.
In vitro release kinetics
The in vitro release profiles of BN from BN-PEG-NLC, BN-NLC, or BN solution are shown in . The release profiles of BN from both BN-PEG-NLC and BN-NLC were fitted to the Ritger–Peppas modal, and the equations were as follows respectively: lnQ = 0.5412 lnt + 2.7582, r = 0.9860; lnQ = 0.5472 lnt + 2.6437, r = 0.9896. The drug release rate was rapid in the initial period (the first 12 h), and then remained at a steady and lower level. In the BN-PEG-NLC in the release medium, approximately 90% of BN was released during 24 h whereas in the BN-NLC, 80% of BN was released in the same period. After 48 h, both NLC reached completely release. The release of BN from BN solution was fast and complete with the first 8 h.
In vivo pharmacokinetic evaluation
presents the plasma concentration-time profiles of BN following single dose i.v. administration of BN solution, BN-NLC, and BN-PEG-NLC. BN solution was rapidly cleared from the circulation within 8 h, whereas BN-NLC and BN-PEG-NLC exhibited a prolonged plasma circulation time (24 h and 36 h, respectively). The plasma drug concentration-time curves of three formulations were all fitted with the two-compartment open model, which was chosen to analyze the pharmacokinetic data. presents the pharmacokinetics parameters. BN-PEG-NLC exhibited maximum AUC followed by BN-PEG and BN solution. BN-PEG-NLC exhibited a 7.2-fold increase (33.26 ± 4.27 versus 4.62 ± 0.63 mg/L. h) in comparison to BN solution, while BN-NLC exhibited 3-fold increase (14.02 ± 1.33 versus 4.62 ± 0.63 mg/L. h) in AUC. Similarly, BN-PEG-NLC exhibited 4.2-fold increase in t1/2β (9.82 ± 0.87 versus 2.32 ± 0.31 h) in comparison to BN solution, while BN-NLC displayed 1.8-fold increase (4.29 ± 0.54 versus 2.32 ± 0.31 h). The plasma clearance (CL) and the volume of distribution (V) of BN-PEG-NLC (0.76 L/kg/h and 4.26 L/kg) were lower than those of BN solution (2.61 L/kg/h and 8.31 L/kg).
Figure 3. The plasma concentration-time profiles of BN following single dose i.v. administration of BN solution, BN-NLC, and BN-PEG-NLC. BN: baicalin; PEG: polyethylene glycol; NLC: nanostructured lipid carriers.
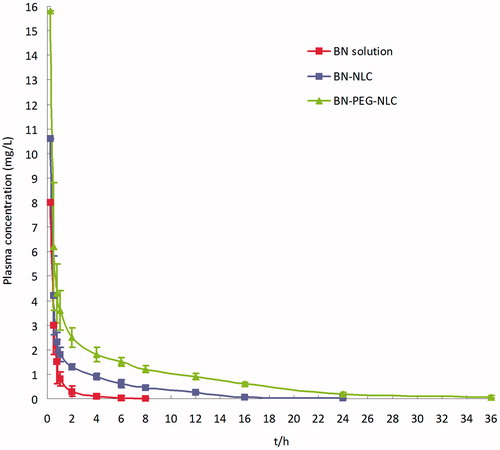
Table 2. Pharmacokinetic parameters of BN after i.v. injecting of BN solution, BN-NLC, and BN-PEG-NLC in rats at a dose of 10 mg/kg (n = 6).
Biodistribution evaluation
shows BN tissue concentration of heart, liver, spleen, lung, kidney, and brain at 15 min following i.v. administration of BN solution, BN-NLC, and BN-PEG-NLC in acute MI rat models. BN-PEG-NLC exhibited maximum drug concentration in the heart and plasma followed by BN-PEG and BN solution. BN-PEG-NLC exhibited a 2.9-fold increase (36.26 ± 5.23 versus 12.33 ± 2.17 mg/L) in comparison to BN solution, while BN-NLC exhibited 1.6-fold increase (36.26 ± 5.23 versus 12.33 ± 2.17 mg/L) in heart drug concentration. Similarly, BN-PEG-NLC exhibited 2.0-fold increase in plasma drug concentration (15.91 ± 1.87 versus 7.92 ± 1.12 mg/L) in comparison to BN solution, while BN-NLC displayed 1.35-fold increase (10.83 ± 1.46 versus 2.32 ± 0.31 mg/L).
Effects of BN-PEG-NLC, BN-NLC, and free BN on infarct size
and show the effect of BN solution, BN-NLC and BN-PEG-NLC on infract size. BN-NLC caused a significant reduction in infract size as compared with the BN solution group and the AMI group (37.8 ± 3.2%, 48.9 ± 4.6% versus 61.9 ± 5.7%, respectively, p < 0.05). BN-PEG-NLC resulted in a further significant reduction in infract size as compared with the BN-NLC group (26.2 ± 3.6% versus 37.8 ± 3.2%, respectively, p < 0.05).
Discussion
The major finding of the present study is that PEG-NLC could be an ideal carrier for BN, which enables passive targeted delivery of BN to the ischemic area of the heart and finally reduced infarct size.
Nano-carriers have been applied to target the heart such as liposomes, micelles, nanoparticles, core-shell hybrid liposomal vesicles, and lipid nanoparticles. The particle size determines the effectiveness of drug delivery to the ischemic myocardium and inflammatory monocytes, as nano-sized drug delivery system (nano-DDS) may accumulate in injured tissues, including ischemic myocardium, where vascular permeability is enhanced (Dauber et al., Citation1990; Baxter, Citation2002; Matoba & Egashira, Citation2014). Hence, we designed NLCs with particle size below 100 nm, which is large enough to go through the leaky blood vessels and small enough to escape the macrophage attack. This is in agreement with reports from Takahama et al. (Citation2009) and Binsalamah et al. (Citation2011). They prepared liposomes or nanoparticles with the size of 100–200 nm respectively and found that nano-sized carriers attained higher drug concentration in the ischemic myocardium than free drugs. Particle sizes of NLCs (BN-PEG-NLC, BN-NLC, and NLC) had no significant variation (p > 0.05). Nanoparticles with zeta potential above −30 mV would have good stability (Wang et al., Citation2015). In our study, the zeta values of NLC were between −30 and −40 mV.
The EE and DL of BN-PEG-NLC and BN-NLC showed that both NLC had good drug-loading capacity. NLC, consist of solid (GMS or PEG-SA) and liquid lipids (OA), have less perfect crystals with many imperfections offering space and thus increase the ability of BN for entering the matrix (Beloqui et al., Citation2016). The EE and DL of BN-PEG-NLC were lower those of BN-NLC. This phenomenon may be due to the decrease of the amount of solid lipid (GMS), which was partially substituted by PEG-SE in BN-PEG-NLC formulations (Luan et al., Citation2014).
The in vitro release studies were performed in PBS (pH 6.8). The release profiles were fitted to the Ritger–Peppas modal (Peppas, Citation2014). In the release curve of both NLC (), a biphasic drug release pattern was found. The rapid release in the initial stage could be due to the release of the drug adsorbed on the surface of NLC. Thereafter, the sustained release could be due to the longer diffusion distance and the lower drug concentration gradient between the NLC and the medium (Yan et al., Citation2015). Apparently, the release rate of BN-PEG-NLC was faster than that of BN-NLC. It could be explained by the fact that amphiphilic PEG chains on the NLC acting as a hydrophilic shield may reduce the surface tension of the NLC, and make the NLC surface to be wet easily (Yuan et al., Citation2007; Liu X et al., Citation2015).
In pharmacokinetic evaluation, BN-PEG-NLC exhibited higher AUC in comparison with BN-NLC and BN solution (p < 0.01). This phenomenon could be due to the sustained release of BN from NLC matrix and the long circulation effect of the PEG chains. It was reported that PEG could improve the surface hydrophilicity of NLC and prevented the absorption of lipoproteins and opsonins effectively (Luan et al., Citation2014). Therefore, the conformational clouds of PEG over the carriers could avoid the recognition of the reticuloendothelial system (RES) and prolong NLC circulation time in vivo (Zhang et al., Citation2013; Pawar et al., Citation2016).
In biodistribution evaluation, BN-PEG-NLC exhibited higher heart BN concentration in comparison with BN solution (p < 0.05). The cardiac distribution tendency of BN-PEG-NLC was higher than that of BN-NLC in MI rats (p < 0.05). This phenomenon could be due to the EPR effect in ischemic tissues, which is the primary mechanism of accumulation of passive targeting nanoparticles in the infarct areas (Frangogiannis et al., Citation2002; Verma et al., Citation2005; Yao et al., Citation2015). It was reported that the PEG can cause steric hindrance in the interaction of nanoparticles with their targets. Therefore, PEG-NLC was an effective carrier for heart-targeted drug delivery. The BN concentration in spleen and kidney was lower in BN-PEG-NLC than other samples. The explanation could be the BN concentration in heart was higher so the concentration in spleen and kidney was lower.
Infarct size is conceived as one of critical indices for evaluating the cardiac damage in the generation of ischemic heart disease. Some reports have proven that BN could ameliorate acute MI (Liu X et al., Citation2013; Wang et al., Citation2013; Kong et al., Citation2014). Whereas the disadvantages of free BN (extensive first-pass metabolism, low bioavailability, short half-life) hindered its clinical application. PEG-NLC could prolong BN circulation time in vivo and increase heart BN concentration, thus achieving significantly decreased infarct size of the heart.
Conclusion
In conclusion, PEG-NLC are the long-circulating and lipid-based nanoparticles that might be used as biocompatible carriers for heart-targeted drug delivery. The results of pharmacokinetic and pharmacodynamic studies revealed that PEG-NLC not only could enhance drug penetration into ischemic tissues but also improve therapeutic efficacy.
Declaration of interest
The authors report no conflicts of interest. The authors alone are responsible for the content and writing of this article.
References
- Almer G, Frascione D, Pali-Schöll I, et al. (2013). Interleukin-10: an anti-inflammatory marker to target atherosclerotic lesions via PEGylated liposomes. Mol Pharm 10:175–86
- Baxter GF. (2002). The neutrophil as a mediator of myocardial ischemia-reperfusion injury: time to move on. Basic Res Cardiol 97:268–75
- Behera SS, Pramanik K, Nayak MK. (2015). Recent advancement in the treatment of cardiovascular diseases: conventional therapy to nanotechnology. Curr Pharm Des 21:4479–97
- Beloqui A, Solinís MÁ, Rodríguez-Gascón A, et al. (2016). Nanostructured lipid carriers: promising drug delivery systems for future clinics. Nanomedicine 12:143–61
- Binsalamah ZM, Paul A, Khan AA, et al. (2011). Intramyocardial sustained delivery of placental growth factor using nanoparticles as a vehicle for delivery in the rat infarct model. Int J Nanomedicine 6:2667–78
- Chen YC, Cao WW, Cao Y, et al. (2011). Using neural networks to determine the contribution of danshensu to its multiple cardiovascular activities in acute myocardial infarction rats. J Ethnopharmacol 138:126–34
- Dauber IM, VanBenthuysen KM, McMurtry IF, et al. (1990). Functional coronary microvascular injury evident as increased permeability due to brief ischemia and reperfusion. Circ Res 66:986–98
- Feng X, Liu Y, Wang X, Di X. (2016). A rapid and sensitive LC-MS/MS method for the determination of linarin in small-volume rat plasma and tissue samples and its application to pharmacokinetic and tissue distribution study. Biomed Chromatogr 30:618–24
- Frangogiannis NG, Smith CW, Entman ML. (2002). The inflammatory response in myocardial infarction. Cardiovasc Res 53:31–47
- Galagudza M, Korolev D, Postnov V, et al. (2012). Passive targeting of ischemic-reperfused myocardium with adenosine-loaded silica nanoparticles. Int J Nanomedicine 7:1671–8
- Galagudza MM, Korolev DV Sonin DL, et al. (2010). Targeted drug delivery into reversibly injured myocardium with silica nanoparticles: surface functionalization, natural biodistribution, and acute toxicity. Int J Nanomedicine 5:231–7
- Go AS, Mozaffarian D, Roger VL, et al. (2014). Heart disease and stroke statistics-2014 update: a report from the American Heart Association. Circulation 129:e28–e292
- Jin JL, Lv RG, Guo J, et al. (2015). Improvement of left ventricular remodeling by inhibition of NF-κB in a rat model of myocardial infarction. Heart Lung Circ pii:S1443-9506:01495–X
- Joshi MD, Müller RH. (2009). Lipid nanoparticles for parenteral delivery of actives. Eur J Pharm Biopharm 71:161–72
- Kong F, Luan Y, Zhang ZH, et al. (2014). Baicalin protects the myocardium from reperfusion-induced damage in isolated rat hearts via the antioxidant and paracrine effect. Exp Ther Med 7:254–9
- Li M, Zheng Y, Shan FY, et al. (2013). Development of ionic-complex-based nanostructured lipid carriers to improve the pharmacokinetic profiles of breviscapine. Acta Pharmacol Sin 34:1108–15
- Lin M, Teng L, Wang Y, et al. (2016). Curcumin-guided nanotherapy: a lipid-based nanomedicine for targeted drug delivery in breast cancer therapy. Drug Deliv 23:1420–5
- Liu X, Gu J, Fan Y, et al. (2013). Baicalin attenuates acute myocardial infarction of rats via mediating the mitogen-activated protein kinase pathway. Biol Pharm Bull 36:988–94
- Liu X, Zhang Z, Jiang Y, et al. (2015). Novel PEG-grafted nanostructured lipid carrier for systematic delivery of a poorly soluble anti-leukemia agent Tamibarotene: characterization and evaluation. Drug Deliv 22:223–9
- Liu Z, Zhao H, Shu L, et al. (2015). Preparation and evaluation of Baicalin-loaded cationic solid lipid nanoparticles conjugated with OX26 for improved delivery across the BBB. Drug Dev Ind Pharm 41:353–61
- Luan J, Zhang D, Hao L, et al. (2014). Preparation, characterization and pharmacokinetics of Amoitone B-loaded long circulating nanostructured lipid carriers. Colloids Surf B Biointerfaces 114:255–60
- Lukyanov AN, Hartner WC, Torchilin VP. (2004). Increased accumulation of PEG-PE micelles in the area of experimental myocardial infarction in rabbits. J Control Release 94:187–93
- Matoba T, Egashira K. (2014). Nanoparticle-mediated drug delivery system for cardiovascular disease. Int Heart J 55:281–6
- Mueller TM, Marcus ML, Mayer HE, et al. (1981). Liposome concentration in canine ischemic myocardium and depolarized myocardial cells. Circ Res 49:405–15
- Pawar H, Surapaneni SK, Tikoo K, et al. (2016). Folic acid functionalized long-circulating co-encapsulated docetaxel and curcumin solid lipid nanoparticles: in vitro evaluation, pharmacokinetic and biodistribution in rats. Drug Deliv 23:1453–68
- Peppas NA. 1. (2014). 1. Commentary on an exponential model for the analysis of drug delivery. Original research article: a simple equation for description of solute release: I II. Fickian and non-Fickian release from non-swellable devices in the form of slabs, spheres, cylinders or discs, 1987. J Control Release 190:31–2
- Qu CY, Zhou M, Chen YW, et al. (2015). Engineering of lipid prodrug-based, hyaluronic acid-decorated nanostructured lipid carriers platform for 5-fluorouracil and cisplatin combination gastric cancer therapy. Int J Nanomedicine 10:3911–20
- Shi ZY, Liu Y, Dong L, et al. (2016). Cortistatin improves cardiac function after acute myocardial infarction in rats by suppressing myocardial apoptosis and endoplasmic reticulum stress. J Cardiovasc Pharmacol Ther pii:1074248416644988
- Simón-Yarza T, Tamayo E, Benavides C, et al. (2013). Functional benefits of PLGA particulates carrying VEGF and CoQ10 in an animal of myocardial ischemia. Int J Pharm 454:784–90
- Takahama H, Minamino T, Asanuma H, et al. (2009). Prolonged targeting of ischemic/reperfused myocardium by liposomal adenosine augments cardioprotection in rats. J Am Coll Cardiol 53:709–17
- Verma DD, Hartner WC, Levchenko TS, et al. (2005). ATP-loaded liposomes effectively protect the myocardium in rabbits with an acute experimental myocardial infarction. Pharm Res 22:2115–20
- Wang X, He F, Liao Y, et al. (2013). Baicalin pretreatment protects against myocardial ischemia/reperfusion injury by inhibiting mitochondrial damage-mediated apoptosis. Int J Cardiol 168:4343–5
- Wang W, Xi M, Duan X, et al. (2015). Delivery of baicalein and paclitaxel using self-assembled nanoparticles: synergistic antitumor effect in vitro and in vivo. Int J Nanomedicine 10:3737–50
- Wang Z, Wang J, Yang S, Hou S. (2015). Construction and in vitro/in vivo evaluation of 17-allylamino-17-demethoxygeldanamycin (17AAG)-loaded PEGylated nanostructured lipid carriers. Drug Dev Ind Pharm May 20:1–8
- Wei Y, Guo J, Zheng X, et al. (2014). Preparation, pharmacokinetics and biodistribution of baicalin-loaded liposomes. Int J Nanomedicine 9:3623–30
- Wei Y, Pi C, Yang G, et al. (2016). LC-UV determination of baicalin in rabbit plasma and tissues for application in pharmacokinetics and tissue distribution studies of baicalin after intravenous administration of liposomal and injectable formulations. Molecules 21:pii: E444. doi:10.3390/molecules21040444
- Yan JK, Ma HL, Chen X, et al. (2015). Self-aggregated nanoparticles of carboxylic curdlan-deoxycholic acid conjugates as a carrier of doxorubicin. Int J Biol Macromol 72:333–40
- Yao C, Shi X, Lin X, et al. (2015). Increased cardiac distribution of mono-PEGylated Radix Ophiopogonis polysaccharide in both myocardial infarction and ischemia/reperfusion rats. Int J Nanomedicine 10:409–18
- Yuan H, Wang LL, Du YZ, et al. (2007). Preparation and characteristics of nanostructured lipid carriers for control-releasing progesterone by melt-emulsification. Colloids Surf B Biointerfaces 60:174–9
- Zhang J, Han X, Li X, et al. (2012). Core-shell hybrid liposomal vesicles loaded with panax notoginsenoside: preparation, characterization and protective effects on global cerebral ischemia/reperfusion injury and acute myocardial ischemia in rats. Int J Nanomedicine 7:4299–310
- Zhang J, Cai W, Zhou Y, et al. (2015). Profiling and identification of the metabolites of baicalin and study on their tissue distribution in rats by ultra-high-performance liquid chromatography with linear ion trap-Orbitrap mass spectrometer. J Chromatogr B Analyt Technol Biomed Life Sci 985:91–102
- Zhang L, Xing D, Wang W, et al. (2006). Kinetic difference of baicalin in rat blood and cerebral nuclei after intravenous administration of Scutellariae Radix extract. J Ethnopharmacol 103:120–5
- Zhang L, Zhao ZL, Wei XH, Liu JH. (2013). Preparation and in vitro and in vivo characterization of cyclosporin A-loaded, PEGylated chitosan-modified, lipid-based nanoparticles. Int J Nanomedicine 8:601–10
- Zhang WL, Gu X, Bai H, et al. (2010). Nanostructured lipid carriers constituted from high-density lipoprotein components for delivery of a lipophilic cardiovascular drug. Int J Pharm 391:313–21