Abstract
Photocatalytic oxidation (PCO) is a promising technology for indoor air purification due to low operating cost, potentially long service life, and low maintenance. Ultraviolet light-emitting diode (UVLED) is a new concept in the field of PCO, which has several advantages over conventional UV light sources. Limited research has been conducted using UVLED PCO for air treatment. This study demonstrated the potential application of UVLED for the removal of volatile organic compounds (VOCs; toluene and xylene) from indoor air under different operating conditions including flow rate (25–117 cubic feet per minute [cfm]), types of catalysts (Degussa P25, sol-gel TiO2, nitrogen-doped TiO2, clay TiO2, and Bi2O3), LED intensity, and humidity in a continuous reactor. About 7–32% VOC removal occurred depending on the experimental conditions. The results show that UVLED can activate different types of photocatalysts effectively.
This study demonstrates the effectiveness of UVLED in photocatalytic oxidation applied for indoor air cleaning. Several TiO2 catalysts (Degussa P25, sol-gel TiO2, nitrogen-doped TiO2, clay TiO2, and Bi2O3) were used in the reactor to characterize the removal performance of indoor air pollutants, for example, VOCs. This is one of the very few studies that have, to date, examined toluene and xylene removal from indoor air using these catalysts with UVLED in a continuous reactor. The intent is to develop an energy-efficient continuous reaction system to remove VOCs from indoor air. The performance of the system was characterized with respect to air flow rate, humidity, types of catalysts, and light intensity.
Introduction
The indoor air quality is of great concern, especially in public places such as schools, offices, shopping malls, etc., mostly due to tightly sealed buildings for energy conservation and in some cases due to poor ventilation. Due to climatic conditions in many parts of the world, people spend most of their time indoors, where diverse indoor air contaminants are present in trace level to very high concentrations. The utilization of synthetic building materials, modern office equipment (photocopiers, laser printers, and computers) and furnishings, cooking and cleaning products, numerous personal care products, as well as outdoor air pollution can contribute to significant indoor air pollution. Indoor air pollutants mainly consist of particulates and chemical and microbial pollutants. Volatile organic compounds (VOCs) are among the most common chemical pollutants present in the indoor air (CitationWorld Health Organization [WHO], 1989). Some of these compounds are associated with sick building syndrome (SBS), including mucous membrane irritation, headache, and fatigue; others are known carcinogens (e.g., formaldehyde, acrolein) (CitationWang et al., 2007). Millions of people are currently suffering from the consequences of poor indoor air quality and billions of dollars per year are lost in the world due to lost productivity because of poor indoor air quality and subsequent poor health (CitationFisk and Rosenfeld, 1997). Among the various technologies that are available for the removal of VOCs from air, photocatalytic oxidation (PCO) is the most promising method mainly due to its capability of complete mineralization of the organic pollutants (CitationTompkins, 2001), whereas most of the other very effective technologies such as filtration and adsorption simply transfer the pollutants from one phase to another, and require frequent replacement of the exhausted units.
PCO using ultraviolet A (UVA) radiation has been widely used for the removal of organics in both air and water. Although active UV photocatalytic oxidation (UVPCO) methods (CitationHodgson et al., 2007) are more efficient, passive methods, in which organic compounds react over irradiated indoor surfaces, have also been found as promising pollution abatement technology. In passive methods, the photocatalytic materials (usually TiO2) are supported on inert materials that must be strongly attached to building surfaces and/or to the air purifiers' hardware, resisting environmental aging and mechanical abrasion. Various support materials for TiO2 include glass, quartz, paper, cement, activated carbon fibers, ceramics, stainless steel, and polymeric matrices such as polyethylene terephthalate (PET) and cellulose acetate. Significant research efforts have been devoted to improve the photocatalytic efficiency of TiO2-based nanoparticles through chemical and physical transformations. Such changes include doping with transition metal ions and nonmetallic elements as well as structural transformations at nano scale, and immobilization on highly porous inert supports (CitationBhattacharyya et al., 2004; CitationKibanova et al., 2009).
CitationKibanova et al. (2009) demonstrated that clay-supported TiO2 (clay TiO2 hereafter) photocatalysts could potentially increase the performance of air treatment technologies via enhanced adsorption and reactivity of target VOCs. Both natural and synthetic clays had received significant attention as supports of TiO2-based photocatalysts for air and water remediation (CitationBhattacharyya et al., 2004; CitationKibanova et al., 2009; CitationKun et al., 2006). Clays often present a large surface area for reversible and irreversible adsorption of organic contaminants. Embedding TiO2 nanoparticles in clay matrices is expected to improve the photocatalytic efficiency by enhancing VOC retention through adsorption in clay pores. In addition, clays can also act as electron acceptors or donors (CitationSolomon, 1968), and have the ability to catalyze diverse chemical processes such as polymerization, reduction, decomposition, or acid-base reactions (CitationErnstsen, 1996).
The most common light source for TiO2 photocatalysis is the black-light-type UV lamp (CitationFujishima and Zhang, 2006), which emits light in the UVA band (λmax = 355 nm). Lately, light-emitting diodes (LEDs) that can emit ultraviolet light, in the range of 360 and 400 nm wavelengths, well suited for activating anatase and rutile-type TiO2, respectively, are being considered as a better light source due to their many advantages. The UVLED (mW range) light sources can save significant amount of energy in comparison with the traditional UV lamps (12 and 16 W) and much safer without the presence of mercury as the source of free electrons and UV photons (CitationChen et al., 2005). In addition, UVLEDs are durable, robust, efficient, and can be installed in any PCO configuration. The first use of UVLED was in the PCO of perchlorothylene (PCE) with Degussa P25 as a catalyst and 49 mW cm−2 of UV light output, with conversion of PCE up to 43% (CitationChen et al., 2005). CitationShie et al. (2008) showed the feasibility of UVLED instead of traditional UV lamp to treat formaldehyde using silver-titanium oxide catalysts where the concentration of formaldehyde decreased by 66% relative to the initial concentration using 40 LEDs (each 20 mW). Although these authors demonstrate the feasibility of UVLED as a light source for PCO, comprehensive and methodical research is needed for establishing UVLED as a light source for greater application. In this work, we demonstrate the applicability of UVLED in photocatalytic oxidation of VOCs such as toluene and xylene using different immobilized catalysts. The photocatalytic activity of several catalysts, such as Degussa P25, sol-gel TiO2, nitrogen-doped TiO2 (N-doped TiO2), clay TiO2, was tested using UVLED in a pilot-scale continuous reactor treating 25–117 cubic feet per minute (cfm) of air spiked with toluene and xylene. Increasingly, application of bismuth oxide (Bi2O3) as a photocatalyst for organic removal is found in literature. Bi2O3 as a photocatalyst has a lot of merits, such as a wide energy gap (from 2 to 3.96 eV), high refractive index, and dielectric permittivity (CitationDuan et al., 2010; CitationHe et al., 2007). In this work, a proprietary Bi2O3 catalyst was tested along with TiO2-based catalysts.
Experimental Method
Materials
Toluene and xylene with ≥99.5% purity and analytical-grade methanol, ethanol, and acetone were purchased from Caledon Chemicals (Georgetown, Ontario, Canada). Titanium tetraisopropoxide with ≥97% purity and sodium bentonite (clay) were purchased from Sigma Aldrich (Oakville, ON, Canada) and Wyo Ben, USA (Billing, MT, USA), respectively. Poly(diallyldimethyl ammonium) (PDDA) (20 wt% solution in water, average molecular weight [MW] 200,000–350,000), poly(sodium 4-styrenesulfonate) (PSS) (30 wt% solution in water, MW = 70,000) were purchased from Sigma Aldrich. TiO2 (Degussa P25) nanoparticles were obtained from Degussa (Dusseldorf, Germany), with average diameter of 20 nm. N-doped TiO2 and Bi2O3 were obtained from Vive Nano (Toronto, ON, Canada).
Experimental setup
The experiments were conducted in a continuous-flow reactor. The schematic diagrams of the experimental setup are shown in and The indoor air purification device mainly consists of two components: the photocatalytic reactor and a gas buffer tank. The gas buffer tank, with a volume of 200 gallons, was used to mix the VOCs with incoming purified air to obtain a concentration between 4 and 15 ppm of VOCs in the inlet of the reactor. Air flow rate in the system was varied from 25 to 117 cfm using a blower (Nutech-99-NBQ-COMBI-456; Lifebreath Indoor Air Systems, London, ON, Canada). The photoreactor with a dimension of 0.66 m × 0.36 m × 0.36 m, and a volume of 0.086 m3 or 26.56 gallons was made of mild steel. The air residence time in the reactor varied from 1.56 to 7.3 sec. The reactor consists of a catalyst-coated screen, and with 60–120 UV light-emitting diodes (LEDs) (DComponents-FG360-R5-WC015; DComponents, South Burlington, VT, USA) mounted on a perforated aluminum sheet. A wire mesh was used for catalyst coating in the reactor and face area of the mesh was 12 in. × 12 in. (McMaster-Carr Supply Company, Elmhurst, IL, USA). Sixty mounted UVLEDs with a maximum UV power of 1.2 mW produced a combined power of 0.072 W at 360 nm. The catalyst loadings were in the range of 10–12 g/m2, and the distance between the catalyst sheet and LEDs was about 5 cm. The arrangements of the catalyst-coated mesh and the UVLEDs in the reactor are shown in It has to be noted that this is a preliminary arrangement to test the concept and further design optimization will be conducted at a later stage. The gas-phase concentration of the VOCs was measured using a semiautomated gas sampling system with a vacuum pump (Welch 8917A; Welch-Ilmvac, Niles, IL), a six-port switching and sampling valve (Valco, 6CW; Valco-Canada, Brockville, ON, Canada) and a 20-μL stainless steel sample loop. The gas samples were analyzed in a gas chromatograph with flame ionization detector (GC-FID; Agilent 7890A; Agilent Technologies, Mississauga, ON, Canada) with a HP-5 (30 m × 320 μm x 0.25 μm; Agilent Technologies, Mississauga, ON, Canada) column. The column temperature was maintained at 50 °C with a ramping rate of 2 °C/min up to 60 °C, with the injector temperature of 250 °C and the detector temperature of 250 °C.
Catalysts preparation
In this work, several catalysts and two different methods of immobilization, namely manual and dip coating of the catalysts on wire mesh, were used. Manual coating using a paint brush was applied mainly for Degussa P25, clay TiO2, and sol-gel TiO2. A modified method of CitationLiu et al. (2007) was used to prepare clay TiO2 catalyst. About 2.4 mL of titanium tetraisopropoxide was mixed with 20 mL of ethanol with constant stirring for about 20 min, and water was added dropwise to the sol-gel solution. Hydrochloric acid (0.5 N) was added dropwise until the solution's color changed to white to clear. Once the solution turned into a transparent gel, 20 mL of ethanol was added to the gel. For clay TiO2, 0.8 g of sodium bentonite and 0.67 g of silica (30 wt%) solution were left to swell in 100 mL of distilled water for 24 hr. The ratio of clay to silica gel was 80 wt% to 20 wt%. The volume of the mixture was brought to 500 mL by adding ultrapure water. Sol-gel diluted with ethanol was then added to the clay solution. The mixture of clay solution and sol-gel was then stirred for 24 hr. The solution was centrifuged at 3800 rpm and the temperature was kept at 25 °C for 30 min. The clay/TiO2 settled at the bottom of the centrifuge was used to coat the wire mesh. The coated wire mesh was kept in an oven at ≈90 °C overnight. The dried wire mesh was then calcined in a furnace for about 10 hr at 450 °C.
Sol-gel TiO2 was prepared by using the method of CitationBhattacharyya et al. (2004): Ten milliliter of glacial acetic acid was mixed with 90 mL of deionized water at room temperature to obtain solution A, and 0.1 mol titanium iso-propoxide was dissolved in 100 mL of anhydrous ethanol with constant stirring to form solution B. Then, solution B was added dropwise into the solution A for 60 min under vigorous stirring. Subsequently, the obtained sol was stirred continuously for 2 hr and coated on mesh. The coated mesh was kept at 90 °C for 6 hr and finally calcined at 600 °C for 4 hr.
Dip coating was used in the preparation of meshes coated with Degussa P25, N-doped TiO2, and Bi2O3 catalysts. The mesh was cleaned by using warm water and detergent, acetone, followed by ultrapure water. After cleaning, the mesh was dried in air. The clean mesh was first deposited with a precursor layer of PDDA/PSS using 3 by 3 cycles of alternate immersion of the mesh in aqueous solutions of PDDA (1.0 mg mL−1) and PSS (1.0 mg mL−1) for 5 min, with intermediate water rinsing and air drying. The deposition of the precursor layer promoted the adhesion of the subsequently deposited nanoparticle films. Then the mesh was alternatively dipped in P25 (Degussa) aqueous solution (1.0 mg mL−1, pH 3.0) and PSS aqueous suspension (1 mg mL−1) for 5 min, with intermediate water rinsing and air drying. By repeating the deposition process of TiO2 and PSS layers in a cyclic fashion, multilayer films of (TiO2/PSS)·n were achieved, where n represents the number of deposition cycles. The prepared coatings were calcined at 500 ˚ C for 1 hr to remove the organic part of the film. The similar procedure was followed for the preparation of N-doped TiO2– and Bi2O3-coated meshes.
Results and Discussion
A series of experiments was conducted to evaluate the effect of important parameters such as type of catalysts, flow rate, type of coating, light intensity, and relative humidity on the removal efficiencies of toluene and xylene using UVLED. Five types of catalysts were used in the experiments, namely, Degussa P25, clay TiO2, Sol-gel TiO2, N-doped TiO2, and Bi2O3. The flow rate was varied from 25 to 117 cfm (residence time varied from 1.56 to 7.3 sec). Prior to actual experiments, accuracy of the analytical system was established by conducting repeated measurements of inlet and outlet concentrations of the VOCs at different flow rates. The errors were calculated on the basis of the differences between experimental and theoretical concentrations, and the maximum error was always less than 10%. Experiments at different concentrations were carried out in duplicate and data were collected under steady state. The conversion of the VOCs was calculated from the difference between the inlet and outlet VOC concentrations, as shown in Equationeq 1:
Dark adsorption
Prior to photocatalysis, dark adsorption of the VOCs on the photocatalysts in absence of UVLED was established at different flow rates. Typical experimental data for toluene and xylene adsorption are shown in and b, respectively. Adsorption equilibrium was achieved within 30 min when the outlet concentration became fairly stable. Thereafter, the light source was turned on to conduct photocatalysis experiments. At 25 cfm air flow rate, the highest adsorption for toluene of 20.5% was found for Degussa P25, followed by 16.5% of clay TiO2, and 12% of sol-gel TiO2. Only 12%, 17%, and 11% of inlet xylene concentration was adsorbed by Degussa P25, clay TiO2, and sol-gel TiO2, respectively. Clay has higher surface area (≈350 m2/g) compared with 50 m2/g of Degussa P25; however, the coating process on the mesh reduced the available surface area. Although mixing due to higher flow rate did not affect adsorption rate significantly, the steady state was reached earlier at higher flow rates. There was much variability in the data in initial 10–15 min due to large volume of the buffer tank used in the experiment.
Photolysis
Prior to the photocatalysis of VOCs, experiments were performed to assess the decomposition efficiency of VOCs by UVLED only; that is, due to photolysis only. Broadly, photodissociation, photolysis, and photodecomposition are chemical reactions in which the bonds of a chemical compound are ruptured due to absorption of photons. The amount of light absorbed at a particular wavelength is proportional to the concentration of the compound and its molar absorbance. Photolysis tests were carried out in the concentration range of 4–15 ppm in absence of catalysts but with the UVLEDs on. The light was switched on once the concentration of the VOCs in the reactor and the outlet was stabilized. and b show the steady-state percent removal of toluene and xylene by photolysis at different air flow rates. The average removal of toluene and xylene due to photolysis at 25 cfm was about 7.5% and 9.5%, respectively. Increasing residence time by lowering the flow rate, and increasing the number of UVLEDs to 120 from 60 increased the percent removal for both the compounds slightly. However, the UV absorbance of both compounds above 320 nm is negligible, and any removal due to photolysis is not discernible accurately within the experimental error (5–10%). Therefore, photolysis by UVLED does not play any significant role on the removal of these compounds.
Photocatalysis
The UVLED photocatalysis of toluene and xylene were conducted with various TiO2 catalysts and the results are shown in and b. It can be seen that the average steady-state removal of toluene at 25 cfm was 31% for Degussa P25, 22.5% for clay TiO2, and 19.5% for sol-gel TiO2, whereas xylene removal under the same condition was 23% for clay TiO2, 22% for Degussa P25, and 16.5% for sol-gel TiO2. For both compounds, the performances of Degussa P25 and clay TiO2 were quite comparable, whereas sol-gel TiO2 did not perform that well. Clay TiO2 and sol-gel TiO2 were used to improve the immobilization and coating of the catalyst on the mesh. However, despite increase in surface area, clay TiO2 did not improve the performance significantly, possibly due to poor manual coating and lower amount of active TiO2 catalyst in comparison with Degussa P25. Earlier, xylene showed better adsorption on clay TiO2, which also showed better performance in photocatalysis of xylene. Because TiO2 is the active catalyst, the catalyst with higher adsorption performance worked better.
Figure 5. Percent removal due to photocatalysis by three types of TiO2 catalysts for (a) toluene and (b) xylene (% removal is average of two experiments).
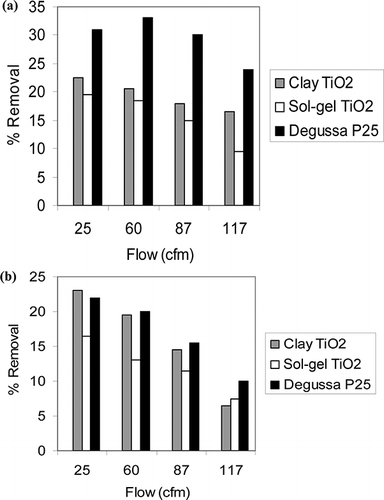
In addition to the above catalysts, two other catalysts, namely, N-doped TiO2 and Bi2O3, were also tested ( and b). Furthermore, two different types of coating methods were also tested: manual and dip coating. Manual coating was used for sol-gel TiO2 and clay TiO2, whereas dip coating was used for N-doped TiO2 and Bi2O3 catalysts. Both manual and dip coating were used for Degussa P25. Other experimental conditions such as flow rate and initial VOC concentration were kept similar for all the catalysts. Percent removal of both compounds for Degussa P25 with both types of coating were quite comparable. For toluene removal at 60 cfm, performances of the catalysts are in the following order: 33% for Degussa P25 > 28% for Bi2O3 > 20.5% for clay TiO2 and N-doped TiO2 > 18.5% for sol-gel TiO2. Similarly, for xylene removal, the following order was found: 25% for dip-coated Degussa P25 > 21% for Bi2O3 > 19.5% for clay TiO2 > 13% for sol-gel TiO2 and N-doped TiO2. Bi2O3 with a band gap of 2.72–2.8 eV can act both as a photocatalyst and a photosensitizer of TiO2. The application of Bi2O3 in photocatalysis is relatively new and no research was found on VOC photodegradation in gas phase using Bi2O3 and UVLED. Although both N-doped TiO2 and Bi2O3 can be activated by visual light, in this work 360-nm UVLED was used to activate all the catalysts. Typically, doped catalyst's performance in UV range is inferior to that in visible light range (data not reported in this work).
Figure 6. Percent removal with UVLED and different catalysts for (a) toluene and (b) xylene (% removal is average of two experiments).
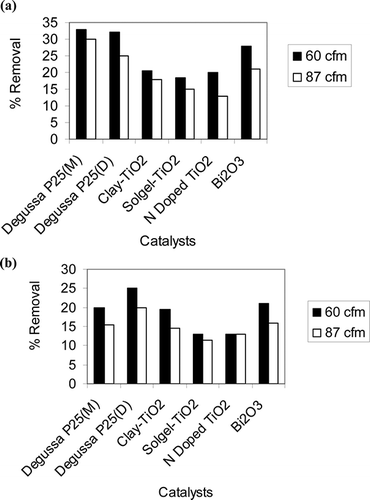
In order to compare the performances of UVLED-activated photocatalysis rate, first-order reaction rate constant was calculated using experimental C/C 0 values (C = concentration at the inlet; C 0 = concentration at the outlet), assuming continuous plug flow reactor at different flow rates, and the values are summarized in . As can be seen, the average rate constants for toluene and xylene for different catalysts vary from 0.053 to 0.15 min−1 and from 0.030 to 0.066 min−1, respectively. These values are higher than the values reported for toluene (0.0146–0.044 min−1) (CitationBouzaza and Laplanche, 2002). However, these authors used much higher concentration of toluene and the intensity used in those experiments was also not provided.
Table 1. First-order rate constants of toluene and xylene under different conditionsFootnote*
Effect of flow
In this study, four different flows, 25, 60, 87, and 117 cfm were used. The influence of flow rate on the degradation of VOCs is illustrated in and b for toluene and xylene using Degussa P25, respectively. Flow rate is a crucial factor for the PCO of organics in gas phase. The flow rate affects the photocatalytic reaction by changing the mass transfer rate and residence time in the reactor. Increased flow rate thus has two counteracting effects of improved mass transfer and lower residence time on the photocatalytic reaction. However, in these experiments, the effect of residence time is more pronounced than the effect of mass transfer. The immobilized catalysts on mesh have mostly external surface area, and diffusion of the VOCs into the pores is probably not an issue.
Effect of humidity
Relative humidity represents one of the most important parameters for PCO in the gas phase. According to CitationVildozo et al. (2010), the impact of humidity has a great effect on the efficiency of air treatment. In the absence of water vapor, the PCO of some VOCs are seriously retarded. On the other hand, with high percentages of water vapor on the catalyst surface, there is an inhibition in reaction rate because of the effect of competitive adsorption between water vapor and the organics. The water molecule adsorbed on the surface of the photocatalyst is oxidized to hydroxyl radical by the hole generated due to photon absorption of the catalyst (CitationVildozo et al., 2010). Increase of relative humidity increases the concentration of hydroxyl radical, which is the major free radical oxidizing the organic compounds. According to CitationLuo and Ollis (1996), the influence of water in photooxidation depends also on the contaminant. Their study using traditional UVA lamp showed that water vapor strongly inhibits isopropanol, trichloroethylene, and acetone oxidation, but enhances toluene oxidation. They also reported that water vapor has no significant effect on 1-butanol oxidation but increases m-xylene oxidation rate up to certain humidity, and decreases thereafter. They also reported that no toluene photodegradation occurred in the total absence of water in the toluene-air mixture. Toluene oxidation rate was enhanced by water concentrations at about 23–40% relative humidity and was somewhat inhibited at 60% relative humidity (CitationLuo and Ollis, 1996). Some studies also proved that the presence of water also affects the generation of PCO by-products. CitationBhowmick and Semmens (1994) showed that the presence of moisture decreases the concentration of phosgene produced by the photooxidation of chlorinated VOCs in the gas phase. In this work, experiments were conducted at 60 and 87 cfm flow rates using three different humidity values: 14%, 17%, and 20%, and sol-gel TiO2. Unfortunately, due to bubbler system used in the experiments, a wide variation in humidity could not be achieved in the reactor. The results shown in and b indicate that VOC removal increased slightly but distinctively with increasing humidity, and the effect is more pronounced at lower flow rate. Although, due to lower range of humidity and small differences in percent removal, the accuracy of data cannot be ascertained, qualitatively these results indicate that the mechanism of photocatalysis using UVLED is similar to that of traditional UVA lamp where hydroxyl radicals are generated in the presence of humidity and irradiated TiO2.
Conclusions
In this study, UVLEDs with 360 nm wave length and a total energy of 72,000 μW (60 LEDS) were used to activate five different types of photocatalysts to remove toluene and xylene from air in a pilot-scale continuous reactor with flow rates varying from 25 to117 cfm. Various catalysts tested included Degussa P25, clay TiO2, sol-gel TiO2, N-doped TiO2, and Bi2O3, and manual and dip coating methods were used to immobilize the catalysts on meshes. The results indicate that the removal of toluene and xylene was significantly affected by flow rate, type of catalyst, and efficiency of coating, and the removal varied from 10% to 32% and from 7.5% to 22% for toluene and xylene, respectively. The results indicate that UVLED activation of photocatalysts follows a similar mechanism as that occurs in PCO using traditional UVA lights.
Acknowledgments
The authors wish to acknowledge the financial support of NSERC, Canada, Nutech Group of Companies, London, Ontario, and the Ontario Centres for Excellence.
References
- Bhattacharyya , A. , Kawi , S. and Ray , M.B. 2004 . Photocatalytic degradation of orange II by TiO2 catalysts supported on adsorbents . Catal. Today , 98 : 431 – 439 . doi: 10.1016/j.cattod.2004.08.010
- Bhowmick , M. and Semmens , M.J. 1994 . Batch studies on a closed loop air stripping process . Water Res. , 28 : 2011 – 2019 . doi: 10.1016/0043-1354(94)90176-7
- Bouzaza , A. and Laplanche , A. 2002 . Photocatalytic degradation of toluene in the gas phase: Comparative study of some TiO2 supports . J. Photobiol. A Chem. , 150 : 207 – 212 . doi: 10.1016/S1010-6030(02)00088-6
- Chen , D.H. , Ye , X.J. and Li , K.Y. 2005 . Oxidation of PCE with a UV LED photocatalytic reactor . Chem. Eng. Technol. , 28 : 95 – 97 . doi: 10.1002/ceat.200407012
- Duan , F. , Zheng , Y. , Liu , L. , Chen , M. and Yi , X. 2010 . Synthesis and photocatalytic behaviour of 3D flowerlike bismuth oxide formate architectures . Mater. Lett. , 64 : 1566 – 1569 . doi: 10.1016/j.matlet.2010.04.046
- Ernstsen , V. 1996 . Reduction of nitrate by Fe2+ in clay minerals . Clays Clay Miner. , 44 : 599 – 608 . doi: 10.1346/CCMN.1996.0440503
- Fisk , W.J. and Rosenfeld , A.H. 1997 . Estimates of improved productivity and health from better indoor environments . Indoor Air , 7 : 158 – 172 . doi: 10.1111/j.1600-0668.1997.t01
- Fujishima , A. and Zhang , X.T. 2006 . Titanium dioxide photocatalysis: Present situation and future approaches . C. R. Chim. , 9 : 750 – 760 . doi: 10.1016/j.crci.2005.02.055
- He , W. , Wei , Q. , Xiaohong , W. , Xianbo , D. , Long , C. and Zhaohua , J. 2007 . The photocatalytic properties of bismuth oxide films prepared through the sol-gel method . Thin Solid Films , 515 : 5362 – 5365 . doi: 10.1016/j.tsf.2007.01.031
- Hodgson , A.T. , Destaillats , H. , Sullivan , D. and Fisk , W.J. 2007 . Performance of ultraviolet photocatalytic oxidation for indoor air cleaning applications . Indoor Air , 17 : 305 – 316 . doi: 10.1111/j.1600-0668.2007.00479.x
- Kibanova , D. , Cervini , S.J. and Destaillats , H. 2009 . Efficiency of clay-TiO2 nanocomposites on the photocatalytic elimination of a model hydrophobic air . Environ. Sci. Technol. , 43 : 1500 – 1506 . doi: 10.1021/es803032t
- Kun , R. , Mogyorosi , K. and Dekany , I. 2006 . Synthesis and structural and photocatalytic properties of TiO2/montmorillonite nanocomposites . Appl. Clay Sci. , 32 : 99 – 110 . doi: 10.1016/j.clay.2005.09.007
- Liu , J. , Li , X. , Zuo , S. and Yu , Y. 2007 . Preparation and photocatalytic activity of silver and TiO2 nanoparticles/montmorillonite composites . Appl. Clay Sci. , 37 : 275 – 280 . doi: 10.1016/j.clay.2007.01.008
- Luo , Y. and Ollis , D.F. 1996 . Heterogeneous photocatalytic oxidation of trichloroethylene and toluene mixtures in air: Kinetic promotion and inhibition . J. Catal. , 163 : 1 – 11 . doi: 10.1006/jcat.1996.0299
- Shie , J.L. , Lee , C.H. , Chiou , C.S. , Chang , C.T. , Chang , C.C. and Chang , C.Y. 2008 . Photodegradation kinetics of formaldehyde using light sources of UVA, UVC and UVLED in the presence of composed silver titanium oxide photocatalyst . J. Hazard. Mater. , 155 : 164 – 172 . doi: 10.1016/j.jhazmat.2007.11.043
- Solomon , D. 1968 . Clay minerals as electron acceptors and/or donors in organic reactions . Clays Clay Miner. , 16 : 31 – 39 . doi: 10.1346/CCMN.1968.0160105
- Tompkins , D.T. 2001 . Evaluation of Photocatalytic Air Cleaning Capability: A Literature Review and Engineering Analysis , ASHRAE Research Project RP-1134.2001 .
- Vildozo , D. , Ferronato , C. , Sleiman , M. and Chovelon , J.M. 2010 . Photocatalytic treatment of indoor air: Optimization of 2-propanol removal using a response surface methodology (RSM) . Appl. Catal. B Environ. , 94 : 303 – 310 . doi: 10.1016/j.apcatb.2009.11.020
- Wang , X. , Meng , S. , Zhang , X. , Wang , H. , Zhong , W. and Du , Q. 2007 . Multi-type carbon doping of TiO2 photocatalyst . Chem. Phys. Lett. , 444 : 292 – 296 . doi: 10.1016/j.cplett.2007.07.026
- WHO . 1989 . “ Indoor air quality: organic pollutants ” . In Euro Report and Studies , Vol. 111 , Copenhagen : WHO Regional Office for Europe .