Abstract
Corona discharge based techniques are promising approaches for oxidizing elemental mercury (Hg0) in flue gas from coal combustion. In this study, in-situ soft X-rays were coupled to a DC (direct current) corona-based electrostatic precipitator (ESP). The soft X-rays significantly enhanced Hg0 oxidation, due to generation of electrons from photoionization of gas molecules and the ESP electrodes. This coupling technique worked better in the positive corona discharge mode because more electrons were in the high energy region near the electrode. Detailed mechanisms of Hg0 oxidation are proposed and discussed based on ozone generation measurements and Hg0 oxidation behavior observations in single gas environments (O2, N2, and CO2). The effect of O2 concentration in flue gas, as well as the effects of particles (SiO2, TiO2, and KI) was also evaluated. In addition, the performance of a soft X-rays coupled ESP in Hg0 oxidations was investigated in a lab-scale coal combustion system. With the ESP voltage at +10 kV, soft X-ray enhancement, and KI addition, mercury oxidation was maximized.
Implications: Mercury is a significant-impact atmospheric pollutant due to its toxicity. Coal-fired power plants are the primary emission sources of anthropogenic releases of mercury; hence, mercury emission control from coal-fired power plant is important. This study provides an alternative mercury control technology for coal-fired power plants. The proposed electrostatic precipitator with in situ soft X-rays has high efficiency on elemental mercury conversion. Effects of flue gas conditions (gas compositions, particles, etc.) on performance of this technology were also evaluated, which provided guidance on the application of the technology for coal-fired power plant mercury control.
Introduction
Mercury (Hg) pollution is arousing increasing attention due to its toxicity. Coal-fired power plants are the primary emission sources of anthropogenic releases of mercury and accounted for 53% of the total mercury emission in United States in 2005 (U.S. Environmental Protection Agency [EPA], Citation2011). In 2011, the EPA finalized the first U.S. national standard to regulate mercury emission from coal-fired power plants.
In flue gas from coal-fired power plants, mercury is usually present in ionic, particulate, and elemental states (Korpiel and Vidic, Citation1997; Fujiwara et al., Citation2002). Due to its high solubility in water, the ionic state of mercury can be removed by treatments such as wet scrubbers. Particulate mercury can be removed by particle treatment facilities such as electrostatic precipitators (ESPs) or a fabric filter baghouse. However, elemental mercury (Hg0) that is present as vapor is hard to capture.
Several types of approaches have been proposed to treat Hg0 in flue gas, including activated carbon injection (ACI) (Sjostrom et al., Citation2010), noncarbon sorbent injection (Worathanakul et al., Citation2008; Suriyawong et al., Citation2009), addition of oxidizing chemicals, such as chlorine (Richardson et al., Citation2003), and selective catalytic reaction (SCR) (Gao et al., Citation2013). Among them, ACI and SCR are already commercially available. ACI is the most commonly used technique, but it has limitations, such as high cost and a low applicable temperature range. The activated carbon also affects the reuse of the fly ash. The oxidation effect of SCR was quite variable and appears to be coal specific and catalyst specific (Feeley et al., Citation2003).
Corona discharge has been proven to oxidize Hg0 (Urabe et al., Citation1988), and its operation cost is much lower than that of ACI. There are three types of corona discharge: alternating current (AC), direct current (DC), and pulsed. Dielectric barrier discharge (DBD), a type of AC corona discharge, has been well studied as a Hg0 oxidation technique (Chen et al., Citation2006; Jeong and Jurng, Citation2007; Byun et al., Citation2008, Citation2011a, Citation2011b; Ko et al., Citation2008c; Lin et al., Citation2010; Wang et al., Citation2010; Jiang et al., 2012). Similarly, DC corona discharge (Liang et al., Citation2002; Wang et al., Citation2009, Citation2011a, Citation2013a) and pulsed corona discharge (Liang et al., Citation1998; Ko et al., Citation2008a, Citation2008b; Xu et al., Citation2009) have also been used to oxidize Hg0. Notably, corona discharge has additional beneficial effects other than Hg0 oxidation. DBD, pulsed corona discharge, and the system based on DC corona discharge by Wang et al. (Citation2013a) can also oxidize nitrogen oxides (NOx) and sulfur oxides (SOx). The corona-based ESP developed by Liang et al. (Citation2002) can remove both Hg0 and zinc-rich fly ash particles.
Although the process of Hg0 oxidation by corona discharge has been studied for years, its role in Hg0 oxidation by DC-energized ESPs in practical flue gas treatment is not clear. Hg0 could be captured and partially oxidized in ESPs at coal-fired power plants. In pilot-scale and full-scale ESPs, 8–58% Hg0 concentration reductions have been reported (Xie et al., Citation2002; Wang et al., Citation2008; Hu et al., Citation2009; Yang et al., Citation2013). However, the Hg0 oxidation in such processes was attributed to oxidizing compounds in the flue gas, such as hydrogen chloride (HCl). The effect of corona discharge was not investigated.
Soft X-ray radiation has been coupled with ESP to enhance its ability to collect particulate matter (Kulkarni et al., Citation2002; Hogan et al., Citation2004; Kettleson et al., Citation2009, Citation2013; Li et al., Citation2009b). Collection efficiency is enhanced when X-rays interact with particles, electrodes, and gas molecules to emit electrons, thereby generating positively charged particles and ions, which then increase particle charging. Considering this, soft X-ray radiation may also be able to enhance Hg0 oxidation in ESPs, based on the higher ionization environment in ESPs and also the potential interactions between soft X-rays and Hg atoms.
This study first evaluated the effect of a DC corona discharge–based ESP with in situ soft X-ray irradiation on mercury oxidation, considering both DC corona discharge alone and the combined effect of corona discharge and soft X-rays. In addition, the effects of flue gas composition and representative fly ash and sorbent particles in flue gas were evaluated in synthetic flue gas. Finally, mercury oxidation by ESP and soft X-rays was tested in a flue gas from coal combustion in a drop-tube furnace.
Experimental
Experimental setup
A cylinder-wire ESP was used in this study. The configuration of the ESP and the coupling of the ESP and the photoionizer are shown in Figure S1 of Supplemental Material. A DC power supply (Glassman High Voltage Inc., series EL; High Bridge, NJ) provided the voltage. The current through the ESP was monitored with a microampmeter (Nishizawa, U-120, class 1.5; Sakaki Sakakimachi, Japan). The soft X-rays were generated by a photoionizer (Hamamatsu Photonics Ltd., Iwata City, Japan; model C7114-01; 3.5–9.5 keV).
Two experimental systems were used. In the simulated flue gas system (), flue gas was obtained by mixing different types of gases (O2, CO2, and N2). Their flow rates were regulated by mass flow controllers (Omega, Stamford, CT; FMA 5510, FMA 5512). The total flow rate was kept at 1 liters per minute (lpm). Mercury was generated at 12 ng/min by a mercury permeation tube (Dynacal; Valco Instruments Co. Inc. and VICI AG, Houston, TX, USA). The effects of silicon dioxide (SiO2; Snowtex-o; Nissan Chemical, Houston, TX), titanium dioxide (TiO2; Aerodisp-W740X; Evonic Industries, Essen, Germany), and potassium iodide (KI; Mallinckrodt Chemicals, St. Louis, MO) particles were studied. Particles were generated by an atomizer (TSI model 3076; Shoreview, MN). Gases and particles were mixed in a clean quartz tube (61.0 cm length, 1.9 cm inner diameter). The ESP was set downstream of the tube. Hg0 concentration and submicron particle size distribution were measured by a mercury analyzer (Nippon Instruments Corporation, Tokyo, Japan; WA-4) and scanning mobility particle sizer (SMPS; TSI, model 3080; Shoreview, MN), respectively. The ozone concentration was measured by an ozone analyzer (Thermo, model 49i; Waltham, MA). An EPA method using a sampling train was used to measure both Hg0 and Hg2+ concentrations (Hedrick et al., Citation2001).
Figure 1. (a) Simulated flue gas experimental system (for experiment sets I to III). (b) Drop-tube furnace experimental system (for experiment set IV).
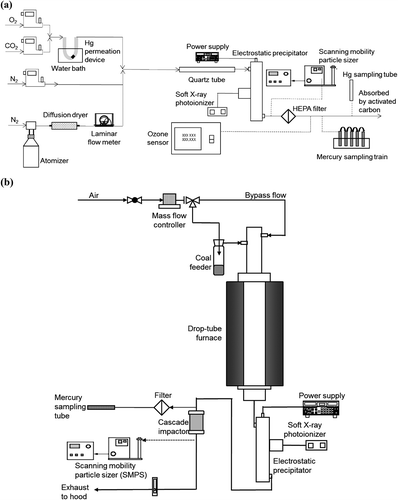
In the second system (), coal was combusted in a drop-tube furnace (Lindberg/Blue; ThermoElectronCorp., Madison, WI, USA) (Zhuang and Biswas, Citation2001; Suriyawong et al., Citation2006; Wang et al., Citation2013b). Pulverized PRB (Powder River Basin) sub-bituminous coal (supplied by Ameren UE, St. Louis, MO) was used in the experiment without KI addition, whereas Chinese S03 coal was used in the KI addition case. The composition of the coals used is given in Table S1. They were fed by a coal feeder (Quann et al., Citation1982) at 1 g/hr. The coal was carried by a 0.5 lpm airflow, and another 2.5 lpm airflow was directly injected into the ceramic tube. The ESP was again set downstream of the furnace, and the same measurements were taken as in the simulated flue gas system. A cascade impactor (Mark III; Pollution Control Systems Corp., Seattle, WA) was set before the SMPS to remove particles larger than 1 μm.
Experimental design
The experimental plan is summarized in . The effects of corona discharge and X-rays on elemental mercury oxidation were first evaluated (in set I). Then, the effects of two factors on this process were evaluated, namely, (1) the flue gas composition effect (pure O2, CO2, and N2, in set II) and (2) the particle effect (in set III). Finally, in set IV the effects of corona discharge and X-rays on elemental mercury oxidation were tested on practical flue gas from coal combustion.
Table 1. Test plan
Results and Discussion
Effects of corona discharge and soft X-rays
Based on previous studies (Kulkarni et al., Citation2002; Namiki et al., Citation2005; Suriyawong et al., Citation2008) and industrial ESP operation (Parker, Citation2003), −10, −8, 0, +8, and +10 kV were selected as the test conditions. Hg0 conversion was used as the indicator of mercury oxidation. The conversion efficiency was calculated using the following equation:
shows that at +10 kV, conversion efficiencies of 49.0% without soft X-rays and 65.6% with soft X-rays were reached, whereas at −10 kV, the conversion efficiencies were lower, 24.1% and 31.5% for ESP and ESP with soft X-rays, respectively. In contrast to the +10 kV and −10 kV cases, the conversion was not significant (below 4.8%) under other applied voltages, due to low electron concentrations in the ESP, which can be inferred from current-voltage characteristics (Figure S2). The phenomenon of higher Hg0 conversion at +10 kV than that at −10 kV could be explained by the electron density distribution, which will be modeled in a following section.
Possible mercury oxidation mechanisms
In the soft X-ray–coupled ESP, a possible Hg0 oxidation mechanism can be summarized in four steps. In step 1, energetic photons from corona discharges and soft X-rays (if applied) ionize gas molecules or electrodes in the ESP and form positive ion-electron pairs (Goldman et al., 1985; Chen, Citation2002; Kulkarni et al., Citation2002). In step 2, the newly generated high-energy electrons collide with gas molecules and the electrodes, which may result in four outcomes: (a) a gas molecule absorbs energy from the collision and dissociates into a positive ion and a new electron; (b) a new electron is released from the electrode after the collision between an electron and the electrode; (c) the gas molecule absorbs energy from the collision and dissociates into atoms; and (d) the gas molecule absorbs energy from the collision and transforms to the excited state. As a result of processes a and b, a great number of electrons are generated, and they initiate more collisions. The processes occurring in steps 1 and 2 are summarized in . The positive-voltage case () and negative-voltage case () have two major differences: the drifting directions of positive ions are opposite between the two cases, so are the drifting direction of electrons; and the process 2b just happens in the negative-voltage case. In step 3, nonelectron species generated in step 2 take part in further chemical reactions. The detailed reactions and produced species depend on the gas composition in the ESP will be discussed in later sections. In step 4, the oxidizing radicals generated in steps 2 and 3 react with and oxidize Hg0. For example, oxygen atoms (O) and ozone molecules (O3) oxidize Hg0 in the following pathways (Van Veldhuizen, Citation2000; Wang et al., Citation2009):
Figure 3. Schematic of ion and electron generation and movement in ESP coupled with soft X-rays (the serial numbers correspond to the steps discussed in the text). (a) Positive-voltage case; (b) negative-voltage case.
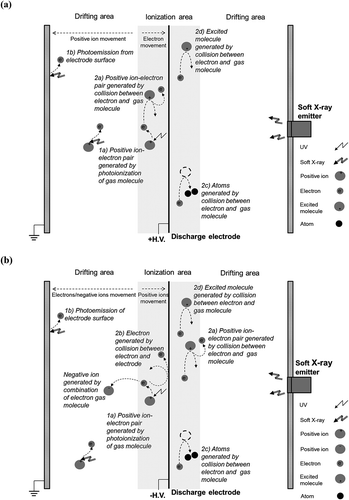
Production of Hg2+ was confirmed with mercury speciation measurement (Figure S3).
Applying soft X-rays resulted in more electron production in step 1, which promoted the electron impact reactions and chemical reactions in steps 2 and 3. Consequently, more radicals were formed and resulted in a high Hg0 oxidation rate. However, under soft X-rays alone, only an insignificant amount of Hg0 was oxidized, as shown in . There are two possible reasons. First, without an electric field in the ESP, the energy of electrons generated by photoionization due to X-rays was too low to initiate the chain reactions and finally could not oxidize Hg0. Second, when the electric field was off, there was no force driving electrons to move. Therefore, the possibility of collisions between electrons and gas molecules was much lower than when the ESP was on, which resulted in fewer reactions. For these reasons, soft X-rays alone were not used as a test condition in the experiments discussed in the following sections.
The O3 generated by an ESP is an active chemical species that oxidizes mercury. O3 generations under various voltages with soft X-rays on or off are shown in . In the voltage ranging from −8.8 to +10 kV, under the same voltage, the concentration of O3 was slightly higher when the soft X-rays were on than when it was off. However, when the voltage was between −10 and −8.8 kV, X-rays reduced O3 generation. Comparing the O3 generation with the Hg0 conversion result in , a higher O3 concentration was obtained under −10 kV than under +10 kV; however, Hg0 conversion was higher under +10 kV. Therefore, O3 might not be the most important species in oxidizing Hg0. This conclusion matches the theoretical calculation. The reduction of Hg0 concentration due to reactions with O3 in the ESP was calculated using
Kinetic parameters for this reaction have been reported in the literature (k is 2.1 × 10−18 cm3 molecule−1 sec−1, a is 1.0, and b is 0.8 [Hall, Citation1995]; k is 7.5 × 10−19 cm3 molecule−1 sec−1, a is 1.0, and b is 1.0 [Pal and Ariya, Citation2004]). With these kinetic parameters, the estimated Hg0 conversion efficiency due to reactions with O3 is at least 1 order of magnitude lower than the Hg0 conversion efficiencies for the experimental cases. Thus, it can be inferred that the reaction between Hg0 and O3 might not be the key mechanism of Hg0 conversion, and that O radicals contribute most in Hg0 oxidation in the system.
The above-mentioned results showed that a positive ESP voltage created better Hg0 oxidation than a negative one. Hence, a voltage of +10 kV was applied to the ESP in subsequent experiments.
Effect of flue gas composition
To further elucidate the mechanism, especially how gas composition determines radical formation (step 3 in the mechanism), Hg0 conversion was measured under pure O2, CO2, and N2, respectively. The Hg0 conversions and current measurements are shown in , with simulated flue gas scenarios as reference, and O3 generations in those cases are shown in Figure S4.
Figure 5. Mercury conversion efficiencies by ESP and soft X-rays under single-gas environments (total flow rate: 1 lpm; ESP currents for various cases are above the bars; gas combination: 4% O2, 12% CO2, 84% N2).
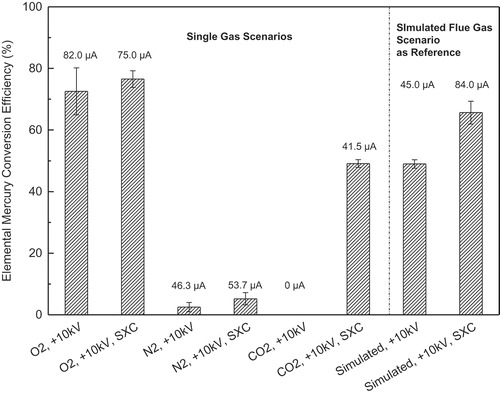
Under pure O2, O and O3 radicals were formed in the following reactions (Chen and Davidson, Citation2002):
When N2 was used, soft X-ray and ultraviolet (UV) radiations as well as high-energy electrons were present in the system, but there was no source for O radicals. In this case, Hg0 conversion was below 5%. These results verify that radicals generated from oxygen are the main cause of Hg0 oxidation rather than direct reactions between Hg0 molecule and high-energy radiations or electrons. The slight Hg0 conversion may be due to ionization of excited Hg atoms in the presence of N2 (Sibata et al., Citation1979).
Under pure CO2, no Hg0 was oxidized by ESP alone, and no current was detected. This result agrees with the previous understanding that CO2 plays a role in stabilizing the discharge process (Wang et al., Citation2009). However, when soft X-rays were added, a current of 41.5 μA and an Hg0 conversion of 49.1% were reached. The main reaction involved is as follows (Skalny et al., Citation2008a, Citation2008b):
This reaction leads to generation of O radicals. However, the extent of reaction was extremely low under corona discharge alone, because the electron impact dissociation rate of CO2 is 4–5 orders of magnitude lower than that of O2 (Li et al., Citation1995; Wang et al., Citation2011a). On the other hand, soft X-ray ionization can generate electrons, which promote the chain reactions and eventually result in a high concentration of radicals. ESP current measurements support this mechanism. No current was observed under ESP alone. However, when soft X-rays were on, a current of 41.5 μA was observed. The Hg0 conversion result indicates that soft X-ray enhancement has great potential in Hg0 oxidation by ESP when high concentration of CO2 is present in flue gas, such as in the oxy-combustion case.
The effect of O2 concentration on Hg0 oxidation by ESP and soft X-rays was also investigated in simulated flue gas (Figure S5). When ESP alone was used, the mercury conversion efficiency significantly increased as the oxygen concentration increased, because O2 was the major source for generation of chemically active species. However, when ESP and soft X-rays were both applied, the Hg0 conversion was relatively stable, because CO2 was also an important source for generation of chemically active species in this condition. The O3 concentrations under various O2 concentrations are shown in Figure S6.
Modeling of electron density in an ESP
Based on the above-discussed mechanisms and reaction pathways, a model for predicting electron density distribution was built. The simulation results can explain why the ESP coupled with soft X-rays oxidized Hg0 better under positive voltages than negative ones. Considering the configuration of the ESP, a one-dimensional axisymmetric model was used to represent a cross-section of the ESP. Its schematic diagram is shown in Figure S7.
Shown in eqs 8–10, the drift-diffusion equation, multicomponent diffusion equations, and Gauss Law are the governing equations for electron density, nonelectron species densities, and the electric field.
The generation rates of species were calculated based on 12 electron impact reactions and 11 chemical reactions (Table S2). The ionization rate due to soft X-ray radiation was estimated based on data from Kulkarni et al. (Citation2002).
Electron density distributions under +10 and −10 kV with and without soft X-rays are shown in . The number of electrons that were close to the electrode, where the electrons had higher energies, was higher for the positive-voltage cases than negative-voltage cases. Electrons having higher energy were more likely to trigger reactions, such as in eqs 5 and 7, through which O radicals were produced. This effect can explain our experimental observation that the Hg0 oxidation rate was higher under positive voltage. Meanwhile, with soft X-rays, the number of electrons was higher, which resulted in a higher Hg0 oxidation rate.
Effect of particles in flue gas
Flue gas usually contains high concentrations of particulate matter. The particles may participate in and influence the process of Hg0 oxidation by ESP and soft X-rays in various ways. The effects of three kinds of particles were studied in this section, including SiO2, TiO2, and KI particles. SiO2, a chemically inert substance, is one of the main components of fly ash particles. TiO2 is a well-known and widely applied photocatalyst that can be activated under UV light and catalyze many reactions (Wold Citation1993; Wang et al., Citation2011b, Citation2012). It has been reported that oxidation of Hg0 in flue gas by oxygen takes place in the presence of nanostructured TiO2 particles and UV light (Wu et al., Citation1998). KI particles were reported to have the ability to oxidize Hg0 in flue gas (Li et al., Citation2009a).
summarizes Hg0 conversions in the ESP with particle injection. The size distributions of the particles are shown in Figure S8. The SiO2 particles had no significant influence on Hg0 conversion. The slight decrease might be due to a small amount of electrons attaching to the particles instead of participating in the chain reactions.
Table 2. Elemental mercury conversion efficiencies under SiO2, TiO2, and KI particle injection (gas combination: 0.04 lpm O2, 0.12 lpm CO2, 0.84 lpm N2)
The TiO2 particles significantly reduced Hg0 conversion. UV photons from corona discharge and soft X-rays had energies higher than 3.0 eV, which is the band gap of TiO2. In this case, UV photons and soft X-rays were absorbed by TiO2 particles, and they excited electrons from TiO2 particles. The generated electron-hole pairs reacted with electron donors or acceptors adsorbed on the surface of TiO2 particles (Chen and Mao, Citation2007; Yates, Citation2009). Thus, the decrease of Hg0 conversion might be because both soft X-rays and UV light from corona discharge were partially absorbed by TiO2 particles. As a result, the extent of gas molecule ionization was reduced, and eventually fewer radicals were produced. On the other hand, the amount of Hg0 that absorbed on the surface of TiO2 particles was low, so the contribution of the TiO2 photocatalytic pathway on Hg0 oxidation was insignificant. Overall, TiO2 particle injection in this test reduced the Hg0 conversion. However, it has been reported that TiO2 particles fixed in a DBD reactor can react with corona discharge in the reactor and enhance mercury oxidation (Yang et al., Citation2012a, Citation2012b), which is contrary to the results of this study. The explanation is that in the specific experimental conditions of Yang et al. (Citation2012a, Citation2012b), active sites containing OH functional groups were formed on the surface of TiO2 under UV radiation, which resulted in Hg0 oxidation.
KI particles increased the Hg0 conversion by introducing a new pathway for Hg0 oxidation (Li et al., Citation2009a):
Mercury oxidation in flue gas from coal combustion
Mercury oxidation by ESP and soft X-rays was tested in flue gas from coal combustion in a drop-tube furnace (). The particle size distribution in the flue gas from PRB coal combustion is shown in . The size distribution is typical for coal combustion (Zhuang and Biswas, Citation2001; Suriyawong et al., Citation2006; Li et al., Citation2009b; Wang et al., Citation2013b). also shows that the ESP can remove at least 99.7% of the fly ash particles. For flue gas with particles, no clear Hg0 conversions were observed (conversion efficiencies were 1.1 ± 0.1% with ESP alone, and 1.5 ± 0.2% with both ESP and soft X-rays).
Comparing the flue gas from drop-tube furnace with the simulated gas used in experiment set I, the composition of the former is more complicated and contains water vapor, SO2, HCl, and NOx. Water vapor can enhance Hg0 oxidation due to ∙OH radical generation under corona discharge (Ko et al., Citation2008a; Wang et al., Citation2009; Yang et al., Citation2012b). SO2 and HCl also can enhance Hg0 conversion (Figures S10 and S11). NO interferes Hg0 oxidation because it competes with Hg0 on reacting with oxidizing radicals (Jeong and Jurng, Citation2007; Ko et al., Citation2008a, Citation2008c; Wang et al., Citation2009).
Based on the above discussion and the results presented in the last section, two factors, NO and particles in the flue gas, are possible to interfere Hg0 oxidation. However, Hg0 conversions in particle-free flue gas (particles were filtered out by a HEPA [high-efficiency particulate arrestance] filter before flying into the ESP) were still extremely low (conversion efficiencies were 0 with ESP alone, and 3.9 ± 0.4% with both ESP and soft X-rays). Therefore, particles were not the main reason for the conversion reduction. NO in the flue gas was the cause of the conversion reduction.
shows the conversions in the cases where 200 ppm KI was mixed into the coal before combustion. Higher conversions were observed when the ESP and soft X-rays were turned on, which evidences that Hg0 can be oxidized by ESP and soft X-rays in this condition. If the Hg0 concentration in the flue gas with ESP and soft X-rays off was used as baseline, the conversions due to ESP and to ESP with soft X-rays were 21.5% and 27.7%, respectively, which are 43.9% and 42.3% of the values for synthetic flue gas. One explanation might be that with I2 in flue gas working as an oxidizer in the ESP, the radicals generated by the ESP and soft X-rays were in excess after the preferred reaction with NO, and they oxidized the Hg0.
Conclusion
The process of Hg0 oxidation by ESP and soft X-rays was investigated. Studies were first conducted in synthetic flue gas. Hg0 was oxidized under +10 kV and −10 kV voltages of the ESP, and +10 kV had the better effect. Soft X-rays enhanced the oxidation. A mechanism for Hg0 oxidation was proposed: electrons were emitted due to photoionization caused by UV radiation from corona discharge and by soft X-ray radiation in the ESP, and then they initiated chain reactions that produced electrons, intermediate species, and other products. Radicals, such as O and O3, were produced in the chain reactions, and they oxidized Hg0. A model simulating the plasma processes was built for predicting the electron distribution in the ESP.
Oxidation mechanisms were further demonstrated by experiments conducted under single-gas environments. Especially, in pure CO2, soft X-ray photoionization showed significant enhancement on Hg0 oxidation. SiO2 particles did not have a significant effect on Hg0 oxidation process; TiO2 particles absorbed photons from corona discharge and soft X-rays and inhibited the Hg0 oxidation; KI particles enhanced Hg0 oxidation, because I− could be oxidized into I2 radicals.
In practical flue gas from coal combustion, the effects of ESP and soft X-rays on Hg0 oxidation were not significant, which might be due to interference from NO. With KI addition to the coal, beneficial effects of ESP and soft X-rays on Hg0 oxidation could be observed. With the ESP voltage at +10 kV, soft X-ray enhancement, and KI addition, mercury oxidation was maximized.
Nomenclature
α = | = | order of reaction between elemental mercury and ozone with respect to ozone |
b = | = | order of reaction between elemental mercury and ozone with respect to elemental mercury |
c = | = | concentration |
= | average concentration in the ESP | |
∆c = | = | change of concentration |
= | diffusivity | |
= | intensity of electric field | |
= | diffusive flux vector | |
k = | = | reaction rate constant |
n = | = | number concentration |
R = | = | generation rate |
s = | = | space charge density |
t = | = | time |
= | mass averaged fluid velocity vector | |
w = | = | mass fraction |
= | permittivity | |
= | electric mobility | |
ρ = | = | density |
Subscripts | = | |
e = | = | electron |
Hg0–O3 = | = | reaction between elemental mercury and ozone |
i = | = | species i |
off = | = | ESP is off |
on = | = | ESP is on |
0 = | = | reference index (represent vacuum in eq 10) |
Acknowledgment
This work was supported by the Consortium for Clean Coal Utilization. The authors thank Dr. Brent Williams and Dhruv Mitroo for helping measure the ozone concentration, and Dr. James Ballard for careful reading and editing of the manuscript.
Supplemental Material
Supplemental data for this article can be accessed at http://dx.doi.org/10.1080/10962247.2014.998352.
Supplemental Materials
Download MS Word (550.4 KB)Additional information
Notes on contributors
He Jing
He Jing and Xiaofei Wang are doctoral students, Wei-Ning Wang is a research assistant professor, and Pratim Biswas is the department chair and Lucy & Stanley Lopata Professor at the Department of Energy, Environmental and Chemical Engineering, Washington University in St. Louis, St. Louis, MO, USA.
References
- Byun, Y., K.B. Ko, M. Cho, W. Namkung, D.N. Shin, J.W. Lee, D.J. Koh, and K.T. Kim. 2008. Oxidation of elemental mercury using atmospheric pressure non-thermal plasma. Chemosphere 72:652–658. doi:10.1016/j.chemosphere.2008.02.021
- Byun, Y., D.J. Koh, and D.N. Shin. 2011a. Removal mechanism of elemental mercury by using non-thermal plasma. Chemosphere 83:69–75. doi:10.1016/j.chemosphere.2010.12.003
- Byun, Y., D.J. Koh, D.N. Shin, M. Cho, and W. Namkung. 2011b. Polarity effect of pulsed corona discharge for the oxidation of gaseous elemental mercury. Chemosphere 84:1285–1289. doi:10.1016/j.chemosphere.2011.05.044
- Chen, J. 2002. Direct-current corona enhanced chemical reactions. Ph.D. thesis, University of Minnesota, Minneapolis, Minnesota, USA.
- Chen, J., and J.H. Davidson. 2002. Ozone production in the positive DC corona discharge: Model and comparison to experiments. Plasma Chem. Plasma Process. 22:495–522. doi:10.1023/A:1021315412208
- Chen, X., and S.S. Mao. 2007. Titanium dioxide nanomaterials: Synthesis, properties, modifications, and applications. Chem. Rev. 107:2891–2959. doi:10.1021/cr0500535
- Chen, Z., D.P. Mannava, and V.K. Mathur. 2006. Mercury oxidization in dielectric barrier discharge plasma system. Ind. Eng. Chem. Res. 45:6050–6055. doi:10.1021/ie0603666
- Feeley, T.J., L.A. Brickett, and J.T. Murphy. 2003. Evaluation of the effect of SCR NOx control technology on mercury speciation. http://www.netl.doe.gov/technologies/coalpower/ewr/pubs/SCRHgPaperFinal030503.pdf ( accessed December 21, 2013).
- Fujiwara, N., Y. Fujita, K. Tomura, H. Moritomi, T. Tuji, S. Takasu, S. Niksa. 2002. Mercury transformations in the exhausts from lab-scale coal flames. Fuel 81:2045–2052. doi:10.1016/S0016-2361(02)00156-4
- Gao, Y., Z. Zhang, J. Wu, L. Duan, A. Umar, L. Sun, Z. Guo, and Q. Wang. 2013. A critical review on the heterogeneous catalytic oxidation of elemental mercury in flue gases. Environ. Sci. Technol. 47:10813–10823. doi:10.1021/es402495h
- Hall, B. 1995. The gas phase oxidation of elemental mercury by ozone. Water Air Soil Pollut. 80:301–315. doi:10.1007/BF01189680
- Hedrick, E., T.G. Lee, P. Biswas, and Y. Zhuang. 2001. The development of iodine based impinger solutions for the efficient capture of Hg0 using direct injection nebulization-inductively coupled plasma mass spectrometry analysis. Environ. Sci. Eng. 35:3764–3773. doi:10.1021/es010648r
- Hogan, C.J., Jr., M.H. Lee, and P. Biswas. 2004. Capture of viral particles in soft X-ray–enhanced corona systems: Charge distribution and transport characteristics. Aerosol Sci. Technol. 38:475–486. doi:10.1080/02786820490462183
- Hu, C.X., J.S. Zhou, S. He, L. Zhang, J.M. Zhang, Z.Y. Luo, K.F. Cen. 2009. Influence and control of electrostatic precipitators and wet flue gas desulfurization systems on the speciation of mercury in flue gas. J. Power Eng. 29:400–404.
- Jeong, J., and J. Jurng. 2007. Removal of gaseous elemental mercury by dielectric barrier discharge. Chemosphere 68:2007–2010. doi:10.1016/j.chemosphere.2007.01.044
- Jiang, Y., J. An, K. Shang, D. Jiang, J. Li, N. Wu, and Y. Lu. 2013. Oxidation efficiency of elemental mercury in two DBD plasma reactors. J. Phys. Conf. Ser. 418:012118. doi:10.1088/1742-6596/418/1/012118
- Kettleson, E.M., B. Ramaswami, C.J. Hogan Jr., M.H. Lee, G.A. Statyukha, P. Biswas, and L.T. Angenent. 2009. Airborne virus capture and inactivation by an electrostatic particle collector. Environ. Sci. Eng. 43: 5940–5946. doi:10.1021/es803289w
- Kettleson, E.M., J.M. Schriewer, R.M.L. Buller, and P. Biswas. 2013. Soft-X-ray-enhanced electrostatic precipitation for protection against inhalable allergens, ultrafine particles, and microbial infections. Appl. Environ. Microbiol. 79:1333–1341. doi:10.1128/AEM.02897-12
- Ko, B.K., Y. Byun, M. Cho, W. Namkung, I.P. Hamilton, D.N. Shin, D.J. Koh, and K.T. Kim. 2008a. Pulsed corona discharge for oxidation of gaseous elemental mercury. Appl. Phys. Lett. 92:251503. doi:10.1063/1.2952496
- Ko, B.K., Y. Byun, M. Cho, W. Namkung, I.P. Hamilton, D.N. Shin, D.J. Koh, and K.T. Kim. 2008b. Influence of gas components on the oxidation of elemental mercury by positive pulsed corona discharge. Main Group Chem. 7:191–204. doi:10.1080/10241220802601064
- Ko, B.K., Y. Byun, M. Cho, W. Namkung, D.N. Shin, D.J. Koh, and K.T. Kim. 2008c. Influence of HCl on oxidation of gaseous elemental mercury by dielectric barrier discharge process. Chemosphere 71:1674–1682. doi:10.1016/j.chemosphere.2008.01.015
- Korpiel, J.A., and R.D. Vidic. 1997. Effect of sulfur impregnation method on activated carbon uptake of gas-phase mercury. Environ. Sci. Eng. 31:2319–2325. doi:10.1021/es9609260
- Kuikarni, P., N. Namiki, Y. Otani, and P. Biswas. 2002. Charging of particles in unipolar coronas irradiated by in-situ soft X-rays: Enhancement of capture efficiency of ultrafine particles. J. Aerosol Sci. 33:1279–1296. doi:10.1016/S0021-8502(02)00067-8
- Li, J., W. Sun, B. Pashaie, and S.K. Dhali. 1995. Streamer discharge simulation in flue gas. IEEE Trans. Plasma Sci. 23:672–678. doi:10.1109/27.467989
- Li, Y., M. Daukoru, A. Suriyawong, and P. Biswas. 2009a. Mercury emissions control in coal combustion systems using potassium iodide: Bench-scale and pilot-scale studies. Energy Fuels 23:236–243. doi:10.1021/ef800656v
- Li, Y., A. Suriyawong, M. Daukoru, Y. Zhuang, and P. Biswas. 2009b. Measurement and capture of fine and ultrafine particles from a pilot-scale pulverized coal combustor with an electrostatic precipitator. J. Air Waste Manage. Assoc. 59:553–559. doi:10.3155/1047-3289.59.5.553
- Liang, X., S. Jayaram, P. Looy, A. Berezin, and J.S. Chang. 1998. Pulse corona application for the collection of mercury from flue gas. Paper presented at 1998 IEEE International Symposium on Electrical Insulation, Arlington, VA, June 7–10, 1998.
- Liang, X., P.C. Looy, S. Jayaram, A.A. Berezin, M.S. Mozes, and J.S. Chang. 2002. Mercury and other trace elements removal characteristics of DC and pulse-energized electrostatic precipitator. IEEE Trans. Ind. Appl. 38:69–76. doi:10.1109/28.980355
- Lin, W., B. Zhang, W. Hou, Q. Zhou, and H. Yang. 2010. Enhanced oxidation of elemental mercury in simulated flue gas by non-thermal plasma. Proc. CSEE 30:72–76.
- Namiki, N., K. Cho, P. Fraundorf, and P. Biswas. 2005. Tubular reactor synthesis of doped nanostructured titanium dioxide and its enhanced activation by coronas and soft X-rays. Ind. Eng. Chem. Res. 44:5213–5220. doi:10.1021/ie049247l
- Pal, B., and P.A. Ariya. 2004. Studies of ozone initiated reactions of gaseous mercury: Kinetics, product studies, and atmospheric implications. Phys. Chem. Chem. Phys. 6:572–579. doi:10.1039/B311150D
- Parker, K.R. 2003. Electrical Operation of Electrostatic Precipitators. London: The Institution of Electrical Engineers.
- Qunn, R.J., M. Neville, M. Janghorbani, C.A. Mims, and A.F. Sarofim. 1982. Mineral matter and trace-element vaporization in a laboratory-pulverized coal combustion system. Environ. Sci. Eng. 16:776–781. doi:10.1021/es00105a009
- Richardson, C., T. Machalek, B. Marsh, S. Miller, M. Richardson, R. Chang, M. Strohfus, S. Smokey, T. Hagley, G. Juip, and R. Rosvold. 2003. Chemical addition for mercury control in flue gas derived from western coals. Presented at the Joint EPRI DOE EPA Combined Utility Air Pollution Control Symposium, The Mega Symposium, Washington, DC, May 19–22, 2003.
- Sibata, A., M. Takahasi, H. Mikuni, H. Horiguchi, and S. Tsuchiya. 1979. Chemi-ionization of excited mercury atom with 253.7 nm irradiation in the presence of N2 and CH4. Bull. Chem. Soc. Jpn. 52:15–20. doi:10.1246/bcsj.52.15
- Sjostrom, S., M. Durham, C.J. Bustard, and C. Martin. 2010. Activated carbon injection for mercury control: Overview. Fuel 89:1320–1322. doi:10.1016/j.fuel.2009.11.016
- Skalny, J.D., S. Matejcik, J. Orszagh, R. Vladoiu, and N.J. Mason. 2008a. A study of the physical and chemical processes active in corona discharges fed by carbon dioxide. Ozone Sci. Eng. 30:145–151. doi:10.1080/01919510701863058
- Skalny, J.D., A. Stoica, J. Orszagh, R. Vladoiu, and N.J. Mason. 2008b. Positive and negative corona discharges in flowing carbon dioxide. J. Phys. D Appl. Phys. 41:175211. doi:10.1088/0022-3727/41/17/175211
- Suriyawong, A., M. Gamble, M.H. Lee, R. Axelbaum, and P. Biswas. 2006. Submicrometer particle formation and mercury speciation under O2-CO2 coal combustion. Energy Fuels 20:2357–2363. doi:10.1021/ef060178s
- Suriyawong, A., C.J. Hogan Jr., J. Jiang, and P. Biswas. 2008. Charged fraction and electrostatic collection of ultrafine and submicrometer particles formed during O2–CO2 coal combustion. Fuel 87: 673–682. doi:10.1016/j.fuel.2007.07.024
- Suriyawong, A., M. Smallwood, Y. Li, Y. Zhuang, and P. Biswas. 2009. Mercury capture by nano-structured titanium dioxide sorbent during coal combustion: Lab-scale to pilot-scale studies. Aerosol Air Qual. Res. 9: 394–403. doi:10.4209/aaqr.2009.02.0012
- Urabe, T., Y. Wu, T. Nagawa, and S. Masuda. 1988. Study on Hg, NOx, SOx behavior in municipal refuse incinerator furnace and removal of those by pulse corona discharge. Seiso Giho 13:12–29.
- U.S. Environmental Protection Agency. 2011. Cleaner power plants. http://www.epa.gov/mats/powerplants.html ( accessed July 2013).
- Van Veldhuizen, E.M. 2000. Electrical Discharges for Environmental Purpose: Fundamentals and Applications. New York: Nova Science Publishers.
- Wang, M., Y. Sun, and T. Zhu. 2013a. Removal of NOx, SO2, and Hg from simulated flue gas by plasma–absorption hybrid system. IEEE Trans. Plasma Sci. 41:312–318. doi:10.1109/TPS.2012.2234483
- Wang, M., T. Zhu, H. Luo, P. Tang, and H. Li. 2009. Oxidation of gaseous elemental mercury in a high voltage discharge reactor. J. Environ. Sci. China 21:1652–1657. doi:10.1016/S1001-0742(08)62469-9
- Wang, M., T. Zhu, H. Luo, H. Wang, and W. Fan. 2011a. Effects of reaction conditions on elemental mercury oxidation in simulated flue gas by DC nonthermal plasma. Ind. Eng. Chem. Res. 50:5914–5919. doi:10.1021/ie200130m
- Wang, W.N., W.J. An, B. Ramalingam, S. Mukherjee, D.M. Niedzwiedzki, S. Gangopadhyay, and P. Biswas. 2012. Size and structure matter: Enhanced CO2 photoreduction efficiency by size-resolved ultrafine Pt nanoparticles on TiO2 single crystals. J. Am. Chem. Soc. 134:11276–11281. doi:10.1021/ja304075b
- Wang, W.N., J. Park, and P. Biswas. 2011b. Rapid synthesis of nanostructured Cu-TiO2-SiO2 composites for CO2 photoreduction by evaporation driven self-assembly. Catal. Sci. Technol. 1:593–600. doi:10.1039/C0CY00091D
- Wang, X., S.M. Daukoru, S. Torkamani, W.N. Wang, and P. Biswas. 2013b. Role of exhaust gas recycle on submicrometer particle formation during oxy-coal combustion. P. Combust. Inst. 34:3479–3487. doi:10.1016/j.proci.2012.07.049
- Wang, Y.J., Y.F. Duan, L.G. Yang, S.L. Meng, Z.J. Huang, C.J. Wu, and Q. Wang. 2008. Experimental study on mercury removal by combined wet flue gas desulphurization with electrostatic precipitator. Proc. CSEE 28:64–69.
- Wang, Z.H., S.D. Jiang, Y.Q. Zhu, J.S. Zhou, J.H. Zhou, Z.S. Li, and K.F. Cen. 2010. Investigation on elemental mercury oxidation mechanism by non-thermal plasma treament. Fuel Process. Technol. 91:1395–1400. doi:10.1016/j.fuproc.2010.05.012
- Wold, A. 1993. Photocatalytic properties of TiO2. Chem. Mater. 5:280–283. doi:10.1021/cm00027a008
- Worathanakul, P., P. Kongkachuichay, J.D. Noel, A. Suriyawong, D.E. Giammar, and P. Biswas. 2008. Evaluation of nanostructured sorbents in differential bed reactors for elemental mercury capture. Environ. Eng. Sci. 25:1061–1070. doi:10.1089/ees.2007.0130
- Wu, C.Y., T.G. Lee, G. Tyree, E. Arar, and P. Biswas. 1998. Capture of mercury in combustion systems by in situ-generated titania particles with UV irradiation. Environ. Eng. Sci. 15:137–148. doi:10.1089/ees.1998.15.137
- Xie, R., Y. Duan, Y. Cao, L.C. Li, S. Kellie, W.P. Pan, J.T. Riley, K. Ho, P. Chu, and A. Metha. 2002. Mercury speciation and concentration at ESP in a 100 MWe coal-fired power plant. Abstr. Pap. Am. Chem. Soc. 224:U574.
- Xu, F., Z. Luo, W. Cao, P. Wang, B. Wei, X. Gao, M. Fang, and K. Cen. 2009. Simultaneous oxidation of NO, SO2 and Hg0 from flue gas by pulsed corona discharge. J. Environ. Sci. China 21:328–332. doi:10.1016/S1001-0742(08)62272-X
- Yang, H., W. Hou, H. Zhang, and L. Zhou. 2012a. Oxidation of elemental mercury with non-thermal plasma coupled with photocatalyst. J. Adv. Oxid. Technol. 15:321–327.
- Yang, H., H. Liu, H. Wu, and M. Wang. 2012b. Photochemical removal of gaseous elemental mercury in a dielectric barrier discharge plasma reactor. Plasma Chem. Plasma Process. 32:969–977. doi:10.1007/s11090-012-9393-9
- Yang, L., X. Fan, D. Feng, and Y. Wang. 2013. Mercury removal characteristics of coal-fired power plants. Paper presented at 2013 Third International Conference on Intelligent System Design and Engineering Applications, Hong Kong, China, January 16–18, 2013.
- Yates, J.T., Jr. 2009. Photochemistry on TiO2: Mechanisms behind the surface chemistry. Surf. Sci. 609:1605–1612. doi:10.1016/j.susc.2008.11.052
- Zhuang, Y., and P. Biswas. 2001. Submicrometer particle formation and control in a bench-scale pulverized coal combustor. Energy Fuels 15:510–516. doi:10.1021/ef000080s