ABSTRACT
The biochar derived from rice hull was evaluated for its abilities to remove hydrogen sulfide (H2S) from gas phase. The surface area and pH of the biochar were compared. The biochar derived from rice hull was evaluated for its abilities to remove hydrogen sulfide (H2S) from gas phase. The surface area and pH of the biochar were compared. The different pyrolysis temperature has great influence on the adsorption of H2S. At the different pyrolysis temperature, the H2S removal efficiency of rice hull-derived biochar was different. The adsorption capacities of biochar were 2.09 mg·g–1, 2.65 mg·g–1, 16.30 mg·g–1, 20.80 mg·g–1, and 382.70 mg·g–1, which their pyrolysis temperatures were 100 °C, 200 °C, 300 °C, 400 °C and 500 °C respectively. Based on the Yoon-Nelson model, it analyzed the mass transfer mechanism of hydrogen sulfide adsorption by biochar.
Implications: The paper focuses on the biochar derived from rice hull–removed hydrogen sulfide (H2S) from gas phase. The surface area and pH of the biochar were compared. The different pyrolysis temperatures have great influence on the adsorption of H2S. At the different pyrolysis temperatures, the H2S removal efficiency of rice hull–derived biohar was different. The adsorption capacities of biochar were 2.09, 2.65, 16.30, 20.80, and 382.70 mg·g−1, and their pyrolysis temperatures were 100, 200, 300, 400, and 500 °C, respectively. Based on the Yoon-Nelson model, the mass transfer mechanism of hydrogen sulfide adsorption by biochar was analyzed.
Introduction
Strict environmental regulations motivate scientists and engineers to seek efficient and cost-effective mechanisms by which to remove air pollutants. Hydrogen sulfide (H2S) is a malodorous gas that is harmful to human health and has detrimental effects on a large number of industrial catalysts. The H2S gas is an odiferous and environmentally hazardous material that can be released in wastewater treatment plants, landfill sites, and other oil or gas production processes (Latos et al., Citation2011; Lebrero et al., Citation2011). Any exposure to concentrations exceeding 500 ppm results in a loss of consciousness and can be fatal (Azargohar and Dalai, Citation2011). H2S removal from gas has been the subject of a great deal of research due to the restrictive emission standards that are implemented worldwide (Xiao et al., Citation2008).
Activated carbon is an effective carbonaceous sorbent for H2S removal due to its high surface area and porosity for sorption (Bagreev and Bandosz, Citation2000; Bashkova et al., Citation2009). To enhance its adsorption properties, the impregnation of activated carbon with sodium or potassium hydroxide or salts containing potassium is most commonly used. These impregnated carbons are often strongly alkaline and have a high dispersion of catalytic sites in the pore system. This favors efficient H2S oxidation. Bandosz (Citation2006) has extensively studied activated carbon-based materials as H2S adsorbents. Nevertheless, they point out that there are still some shortcomings in the applications of caustic impregnated carbon because the impregnation decreases the ignition temperature of the carbon and poses a self-ignition risk. Therefore, such limitation directs the attention of researchers toward virgin materials that have high sorption efficiency with improved safety profiles.
As a precursor of AC (activated carbon), biochars have received considerable attention in the past decades (Azargohar and Dalai, Citation2006; Hayes, Citation2006; Renner, Citation2007; Moussavi and Khosravi, Citation2012). Some of the biochars own a structured carbon matrix with the porosity and surface area comparable to the activated carbon; furthermore, most of the biochars are normally alkaline. Therefore, biochars could be a promising substitute for activated carbon as an adsorbent for H2S. Xu et al. (Citation2014) and Ros et al. (Citation2006) showed a good performance of sludge-based char for H2S removal. However, the underlying mass transfer mechanisms for the sorption of H2S are not yet fully elucidated.
The objective of this study is to investigate the performance and mass transfer mechanism of the rich hull–derived biochar as a sorbent for H2S removal.
Methods
Materials
The biochar called SR was produced from rice hull. The rice hull was cut into small pieces, washed, and baked in the oven at 60 °C for 48 hr. The pieces were then ground into small particles using a crusher (Shanghai, China). The sizes of the particles below 0.3 mm were obtained after screening. The particles were pyrolyzed at 100, 200, 300, 400, and 500 °C under an O2-free atmosphere in a ceramic fiber muffle furnace (Shanghai, China). The heating rate was 10 °C·min−1 up to the selected pyrolysis temperature, with a holding time of 5 hr at the final temperature. The samples were cooled by ventilation with nitrogen gas to 30 °C.
Measurement of H2S breakthrough curves
To evaluate the capacity of biochars derived from rice hull at different pyrolysis temperatures for H2S removal, H2S adsorption experiments were performed at a laboratory-scale apparatus at at room temperature (). The biochars were packed into quartz glass columns (12 mm in inner diameter, 300 mm in height). The height of the biochar bed was 150 mm; the bulk density of the biochar bed was 0.12 g·cm−3. The porosity of the whole biochar bed was 35%. The quartz sand was packed above and below the biochar bed. The source gas containing 50 µL·L−1 H2S and 500 mL·L−1 water vapor was passed through the column of adsorbent at 40 mL·min−1. The inlet H2S concentration was changed from 10 to 50 µL·L−1 by diluted compressed air. The load of H2S into the columns was changed by controlling the inlet concentration and/or space velocity. The outlet H2S was monitored using gas chromatography. The test was stopped at the breakthrough concentration of 50 µL·L−1. After every test, the excess of H2S was absorbed using a sodium hydroxide solution. The adsorption capacities of each biochar were calculated by integration of the area above the breakthrough curves, and from the H2S concentration in the inlet gas, flow rate, breakthrough time, and mass of biochar.
GC chromatography
The concentration of H2S in the exhaust gases from the biochar bed was monitored using a Shimadzu gas chromatograph (GC) (model GC2010, Shimadzu, Kyoto, Japan). The separation was conducted on a CNW (Mainz, Germany) CD-1 column (bonded 100% dimethyl siloxane, 30 m long, 0.32 mm internal diameter, and 5.00 µm dimension of film thickness). The GC oven heating program was as follows: (1) 80 °C for 2 min, (2) heating from 80 to 150 °C at 20 °C·min−1, and (3) holding at 150 °C for 18 min. The injector temperature was 80 °C. A flame photometric detector (FPD), with a sulfur filter and an opening temperature of 220 °C, was used.
H2S adsorption capacity calculation
According to Cal et al. (Citation2000), the adsorption capacity of biochar on H2S with x/M, the calculation formula is as follows:
where Q is gas flow rate (m3·s−1), w is the quality of biochar in the fixed bed, MW is the mole mass of H2S (34 mg·mmol−1), VM is molar volume of gas (22.4mL·mmol−1), C0 is the inlet concentration of H2S (mg·m−3), C(t) is the outlet concentration of H2S (mg·m−3), and t is the adsorption saturation time in the fixed bed.
Results and discussion
Characterization of the biochar
The physicochemical characteristics of the different biochars derived from rice hull used in this experiment are shown in . The pyrolysis temperature significantly affects pH and SA (surface area). All biochars were alkaline. The highest pH was 10.54 for SR500, and the lowest was 7.25 for SR100. These values are typical for most biochars generated at high temperatures (Lehmann and Joseph, Citation2009). Higher pH suggests potential to adsorb acidic H2S (Dudka and Adriano, Citation1997). Among the biochars, the highest SA (in cm2) was 115.34 for SR500, which was 25.5 times higher than that of SR100. With the increase of the pyrolysis temperature, the composition of the biomass is decomposed, which leads to the decrease of the carbon content of the biochar. The difference of the N contents among the five biochars is small. The pH and SA are key factors for carbon material to absorb H2S; however, no evidence showed that the change of elemental composition affected the H2S adsorption capacity (Hung et al., Citation2000).
Table 1. Physicochemical characteristics of the three different biochars and activated carbon.
Breakthrough curves
Breakthrough curves represent the time profile for saturation of a given amount of an adsorbent. Such adsorbent is structured as a fixed bed with a given solution of an adsorbate, forced through this bed at a constant rate and fixed temperature. Despite being fully empirical and dependent on almost all variables involved, breakthrough curves serve two purposes: (a) to validate if the adsorbent is efficient for the required separation and (b) to establish the breakthrough time (process interruption), based on a criterion, which is either technical, economic, or legal (Knaebel, Citation1999). shows the breakthrough curves of H2S on different biochars with different pyrolysis temperatures.
Figure 2. Breakthrough curves of H2S on rice hull–derived biochars pyrolyzed at different temperatures.
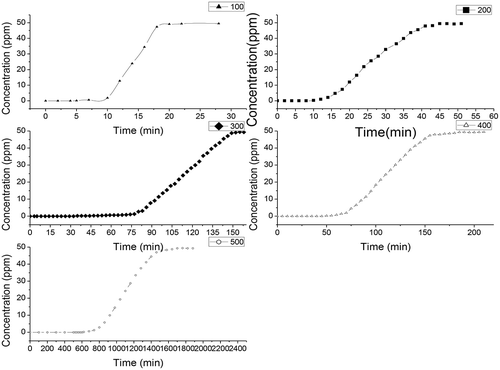
Various factors affect the breakthrough curves, such as the nature of the adsorbate and adsorbent, the column geometry, and operating conditions. Favorable adsorption isotherms and extremely high adsorption rates would result in a virtually coinciding breakthrough time and exhaustion point. Thus, the breakthrough curves become sharp. The breakthrough time is the point on the breakthrough curve where the effluent adsorbate reaches its maximum allowable concentration, which often corresponds to the treatment goal. shows that the breakthrough time is dependent on pyrolysis temperature. The breakthrough time for different biochar increased with increasing pyrolysis temperature. When the pyrolysis temperature increased from 100 to 500 °C, the breakthrough time for different biochars gradually increased. The breakthrough time increased from 5 to 620 min (shown in ). Hossain et al. (Citation2011) and Peng et al. (Citation2011) reported that pyrolysis temperature has a significant effect on the chemical, physical, morphological, and spectral properties of biochars. The scanning electron microscope image presents evidence that the surface area of biochar can be increased with pyrolysis temperature (Brodowski et al., Citation2005; Chun et al., Citation2004; Keiluweit et al., 2010). The increase in surface area provides a greater number of adsorption sites and space for H2S.
Table 2. H2S breakthrough time, saturation time, and H2S breakthrough capacity for the different biochars.
Breakthrough capacity is defined as the mass of the absorbate removed by the adsorbent at breakthrough concentration or the maximum acceptable (desired) concentration. When the effluent concentration reaches this value, the adsorbent requires replacement. summarizes the capacity measured for a breakthrough concentration of 50 ppm. The capacity of the H2S breakthrough ranges from 2.09 to 382.7 mg/g (the data shown in ). Among all samples, SR500 has the strongest capacity of H2S breakthrough. The H2S breakthrough capacity of SR500 is remarkably superior to all other samples reported by Bagreev et al. (Citation2001), the strongest capacity for which was 104.5 mg/g. The saturation time of H2S adsorption is also listed in . The change in the saturation time of samples is similar to the change in the breakthrough time.
The mass transfer analysis of adsorption by biochars
The Yoon-Nelson (Citation1984) model is a theoretical model based on gas adsorption kinetics; when the air flows through the biochars fixed bed, a portion of the gas molecule is adsorbed at the active sites of the microporous structure, and other gas molecules flow through the bed. Q is defined as the adsorption probability; P is defined as the transmission probability. If the 70% gas molecules is fixed in the biochar fixed bed, the 30% is across the fixed bed, then Q = 0.70, P = 0.30.
C1 is the inlet gas concentration in the biochar fixed bed, and Ct is the outlet gas concentration (such as the outlet concentration of 30% shown in ). With the adsorption going on, the concentration eventually will reach the saturation state. Finally, the inlet concentration and the outlet concentration will be equal. Assuming the adsorption capacity by biochars is proportional to the adsorption probability (Q) and penetration probability (P), we can obtain:
This assumption is based on the observation of the changes of velocity of penetration concentration (dCt/dt). The experiments show that the number of the biochar active centers is directly proportional to Ct. In additional, the experimental data show that the biochar bed reduction rate is proportional to the gas concentration (C) and velocity (F), and is inversely proportional to the quality of biochar (Wc). Therefore,
Because the active center number of the biochar in the fixed bed is proportional to the quality of the biochar, therefore,
Introducing the dimensionless proportional coefficient, k, we obtained:
or
The dimension of the proportional coefficient k’ is the reciprocal of time.
To obtain accurate expression of adsorption, eq 6 is required for the solution of differential. Because of P = 1 − Q, we can obtain the following expression:
And k’ is independent of time, we can obtain:
where λ is the adsorption time when the breakthrough concentration is half of the initial concentration, so when Q = P = ½, t = λ. We can obtain:
Integrating eq 9, we obtain the expressions for Q and P:
Integrate eq 11, we can obtain:
Because P = Ct/C1, and setting C = C1, C0 = Ct, we obtain:
where k’ is rate constant (min−1); λ is 50% breakthrough time (min); t is breakthrough time (min); C is outlet concentration (mg·m−3); C0 is inlet concentration (mg·m−3); and k is proportionality constant.
To calculate the theoretical breakthrough curves of the single-component system, it is necessary to determine the two parameters, k’ and λ. The values of these two parameters can be obtained from the experimental data. Using SR500 as test sample to verify the Yoo-Nelson model shows that the experimental data can be fitted well. The results are shown in .
It be seen from , the ln(C/(C0 − C) maintained a good linear relationship with t; the correlation coefficient R = 0.9909. From the fitted straight-line equation, y = 119.9x + 1149.2, the values of k’ and λ can be obtained: k’ = 0.0083, λ = 1149.2. According to the data, integrating eq 13, we can obtain:
Using eq 14, the adsorption breakthrough fitting curves can be obtained (shown in ). When the outlet gas concentration reaches 5% of the inlet concentration, it is beginning to penetrate the biochars fixed bed (this penetration point time is marked as t1). When the outlet gas concentration reaches 95% of the inlet concentration, the adsorption of H2S by biochars reaches the equilibrium state (this adsorption balance point is marked as t2). According to eq 14, under gas flow of 40 mLmin−1, C0 = 50 mg·m−3, t1 = 612 min, t2 = 1580 min are predicted; these values are very close to the experimental data: t1 = 620 min, t2 = 1645 min.
Conclusions
The main conclusion from this study is that biochar derived from rice hull is a promising adsorbent of H2S. The SR500 was superior to other biochars for H2S adsorption, which its breakthrough capacity for adsorbing H2S was 382.7 mg/g. The Yoon-Nelson model can explain the mass transfer mechanism of the adsorption H2S by biochars.
Funding
This study was supported by the Postdoctoral Foundation of China (14Z102060010) and the Innovation of Science and Technology Special-Postdoctoral Research Fund (14X100030005).
Additional information
Funding
Notes on contributors
Guofeng Shang
Guofeng Shang, postdoctor, Department of Environment and Resource, School of Agriculture and Biology, Shanghai Jiao Tong University, Shanghai, People’s Republic of China.
Liang Liu
Liang Liu, doctor, Department of Environment and Resource, School of Agriculture and Biology, Shanghai Jiao Tong University, Shanghai, People’s Republic of China.
Ping Chen
Ping Chen, doctor, Department of Environment and Resource, School of Agriculture and Biology, Shanghai Jiao Tong University, Shanghai, People’s Republic of China.
Guoqing Shen
Guoqing Shen, Professor, Department of Environment and Resource, School of Agriculture and Biology, Shanghai Jiao Tong University, Shanghai, People’s Republic of China.
Qiwu Li
Qiwu Li, engineer, Hunan Environmental Monitoring Center, Changsha, Hunan Changsha, Hunan, People’s Republic of China.
References
- Azargohar, R., and A.K. Dalai. 2006. Biochar as a precursor of activated carbon. Appl. Biochem. Biotechnol. 131:762–773. doi:10.1007/978-1-59745-268-7_62
- Azargohar, R., and A.K. Dalai. 2011. The direct oxidation of hydrogen sulphide over activated carbons prepared from lignite coal and biochar. Can. J. Chem. Eng. 89:844–853. doi:10.1002/cjce.v89.4
- Bagreev, A., and T.J. Bandosz. 2000. Study of hydrogen sulfide adsorption on activated carbons using inverse gas chromatography at infinite dilution. J. Phys. Chem. B 104:8841–8847. doi:10.1021/jp0011037
- Bagreev, A., S. Bashkova, D.C. Locke, and T.J. Bandosz. 2001. Sewage sludge-derived materials as efficient adsorbents for removal of hydrogen sulfide. Environ. Sci. Technol. 35:1537–1543. doi:10.1021/es001678h
- Bandosz, T.J. 2006. Carbonaceous materials as desulfurization media. In Combined and Hybrid Adsorbents: Fundamentals and Applications, ed. J.M. Loureiro and M.T. Kartel, 145–164. Berlin, Germany: Springer Netherlands.
- Bashkova, S., T.R. Armstrong, and V. Schwartz. 2009. Selective catalytic oxidation of hydrogen sulfide on activated carbons impregnated with sodium hydroxide. Energy Fuels 23:1647–1682. doi:10.1021/ef800711c
- Brodowski, S., W. Amelung, L. Haumaier, C. Abetz, and W. Zech. 2005. Morphological and chemical properties of black carbon in physical soil fractions as revealed by scanning electron microscopy and energy-dispersive x-ray spectroscopy. Geoderma 128:116–129. doi:10.1016/j.geoderma.2004.12.019
- Cal, M.P., B.W. Strickle, and A.A. Lizzio. 2000. High temperature hydrogen sulfide adsorption on activated carbon I. Effects of gas composition and metal addition. Carbon 38:1757–1765. doi:10.1016/S0008-6223(00)00010-5
- Chun, Y., G.Y. Sheng, C.T. Chiou, and B.S. Xing. 2004. Compositions and sorptive properties of crop residue-derived chars. Environ. Sci. Technol. 38:4649–4655. doi:10.1021/es035034w
- Dudka, S., and D.C. Adriano. 1997. Environmental impacts of metal ore mining and processing: A review. J. Environ. Qual. 26:590–602. doi:10.2134/jeq1997.00472425002600030003x
- Hayes, M.H.B. 2006. Biochar and biofuels for a brighter future. Nature 443:144–144. doi:10.1038/443144c
- Hossain, M.K., V. Strezov, K.Y. Chan, A. Ziolkowski, and P.F. Nelson. 2011. Influence of pyrolysis temperature on production and nutrient properties of wastewater sludge biochar. J. Environ. Manage. 92:223–228. doi:10.1016/j.jenvman.2010.09.008
- Hung, L.C., H.T. Jiun, L.T. Chien, and C.H. Yi. 2000. Adsorption characteristics of alkaline activated carbon exemplified by water vapor, H2S, and CH3SH gas. Sep. Sci. Technol. 35:903–918.
- Knaebel, K. 1999. The basics of adsorber design. Chem. Eng. 106:92–101.
- Keiluweit, M., P.S. Nico, M.G. Johnson, and M. Kleber. 2011. Dynamic mole-cular structure of plant biomass-derived black carbon (biochar). Environ. Sci. Technol. 44:1247–1253.
- Latos, M., P. Karageorgos, N. Kalogerakis, and M. Lazaridis. 2011. Dispersion of odorous gaseous compounds emitted from wastewater treatment plants. Water Air Soil Pollut. 215:677–679. doi:10.1007/s11270-010-0508-8
- Lebrero, R., L. Bouchy, R. Stuetz, and R. Murioz. 2011. Odor assessment and management in wastewater treatment plants: A review. Crit. Rev. Environ. Sci. Technol. 41:915–950. doi:10.1080/10643380903300000
- Lehmann, J., and S. Joseph. 2009. Biochar for environmental management: An introduction. In Biochar for Environmental Management, Science and Technology, ed. J. Lehmann and S. Joseph, 1–12. London: Earthscan.
- Moussavi, G., and R. Khosravi. 2012. Preparation and characterization of a biochar from pistachio hull biomass and its catalytic potential for ozonation of water recalcitrant contaminants. Bioresour. Technol. 119:66–71. doi:10.1016/j.biortech.2012.05.101
- Peng, X., L. Ye, C. Wang, H. Zhou, and B. Sun. 2011. Temperature- and duration-dependent rice straw-derived biochar: Characteristics and its effects on soil properties of an ultisol in southern China. Soil Tillage Res. 112:159–166. doi:10.1016/j.still.2011.01.002
- Renner, R. 2007. Rethinking biochar. Environ. Sci. Technol. 41:5932–5933. doi:10.1021/es0726097
- Ros, A., M.A. Montes-Moran, E. Fuente, D.M. Nevskaia, and M.J. Martin. 2006. Dried sludges and sludge-based chars for H2S removal at low temperature: Influence of sewage sludge characteristics. Environ. Sci. Technol. 40:302–309. doi:10.1021/es050996j
- Xiao, Y., S. Wang, D. Wu, and Q. Yuan. 2008. Catalytic oxidation of hydrogen sulfide over unmodified and impregnated activated carbon. Sep. Purif. Technol. 59:326–332. doi:10.1016/j.seppur.2007.07.042
- Xu, X.Y., X.D. Cao, L. Zhao, and T.H. Sun. 2014. Comparison of sewage sludge-and pig manure-derived biochars for hydrogen sulfide removal. Chemosphere 111:296–303. doi:10.1016/j.chemosphere.2014.04.014
- Yoon, Y.H., and J.H. Nelson. 1984. Application of gas adsorption kinetics: I. A theoretical model for respirator cartridge service life. Am. Ind. Hyg. Assoc. J. 45:509–511. doi:10.1080/15298668491400197