ABSTRACT
Bacillus subtilis subsp. niger spores are a commonly used biological indicator to evaluate the disinfection of an enclosed space. In the present study, chlorine dioxide (ClO2) gas was applied to inactivate B. subtilis subsp. niger spores in an enclosed space. The effects of the ClO2 gas concentration (1-3 mg/l), relative humidity (RH, 30-70%) and exposure time (30-90 min) were investigated using a response surface methodology (RSM). A three-factor Box-Behnken experimental design was used. The obtained data were adequately fitted to a second-order polynomial model with an R2adj of 0.992. The ClO2 gas concentration, RH and exposure time all significantly (P<0.05) and positively correlated with the inactivation of B. subtilis subsp. niger spores. The interaction between the ClO2 gas concentration and RH as well as that between the exposure time and RH indicated significant and synergistic effects (P<0.05). The predictive model was validated by additional eight experiments and proven to be with good accuracy. Overall, this model established by the RSM could show the trend of the inactivation of spores, indicate the interactions between important factors, and provide a reference to determine effective conditions for the disinfection in different enclosed spaces by ClO2 gas.
Implications: The inactivation of indoor biological contaminants plays an important role in preventing the transmission of pathogens and ensuring human safety. The predictive model using response surface methodology indicates the influence and interaction of the main factors on the inactivation of Bacillus subtilis subsp. niger spores by ClO2 gas, and can predict a ClO2 gas treatment condition to achieve an effective sterilization of enclosed spaces. The results in this paper will provide a reference for the application of ClO2 gas treatments for indoor disinfection.
Introduction
Infectious disease epidemics, such as those of the Ebola virus, Middle East respiratory syndrome coronavirus (MERS-CoV), and severe acute respiratory syndrome (SARS), have attracted increasing attention worldwide (Althaus et al., Citation2015; Petersen et al., Citation2015; Smith, Citation2006). The inactivation of indoor biological contaminants has been shown to play an important role in preventing the transmission of pathogens and ensuring human safety (Aygun et al., Citation2002). Therefore, a high-efficiency sterilization technique is required to effectively decontaminate indoor environments. Compared with liquid sterilization chemicals, fumigating decontaminants feature several advantages such as a high efficiency, large disinfection volume, good penetration ability, and simple operation and have been widely employed to sterilize enclosed spaces (Rogers et al., Citation2007; Goyal et al., Citation2014; Hudson et al., Citation2007).
Chlorine dioxide (ClO2) is a strong oxidizing decontaminant with a broad and high biocidal effectiveness (Aieta and Berg, Citation1986). It has been approved as a disinfectant for agricultural, commercial, industrial, medical, and residential use (U.S. Environmental Protection Agency [EPA], Citation2006). As a promising fumigating decontaminant, ClO2 gas is more effective, less toxic, and less time-consuming than traditional fumigants, such as ethylene oxide and formaldehyde, and it more effectively inactivates microorganisms than aqueous ClO2 (Han et al., Citation2001; Vaid et al., Citation2010). Several studies have shown that ClO2 gas can inactivate all bacteria and spores to completely decontaminate enclosed spaces such as hospital rooms (Lowe et al., Citation2013; Luftman et al., Citation2006), ambulances (Lowe et al., Citation2013), Biosafety Level 3 laboratories and associated ductwork (Lowe et al., Citation2012), cafeterias (Hsu et al., Citation2014), exposure chambers (Pottage et al., Citation2012), and entire buildings (Wood and Martin, Citation2009).
The ClO2 gas concentration, relative humidity (RH), and exposure time have been shown to affect the sterilization effect of ClO2 gas. Lowe et al. (Citation2013) reported that the decontamination of an ambulance could achieve a complete inactivation of B. anthracis and B. atrophaeus spores by ClO2 gas, and decrease in gas concentration, exposure time, and RH would reduce log reductions of spores. Morino et al. (Citation2009) showed that the log reduction of feline calicivirus in the dry state was less than 1.0 in 45–55% RH but 6.1 in 75–85% RH when the organism was treated with 0.26 ppm ClO2 gas for 24 hr. These previous studies mainly focused on the evaluation to the inactivation of different microorganisms by ClO2 gas and demonstrated the significant effect of these parameters. However, the effect tendency and interactions of gas concentration, RH, and exposure time are little researched using multifactor experimental design and mathematical modeling. Additional studies are needed to elucidate the mechanisms underlying this effect as well as the interaction between these parameters.
Biological indicators are typically used to evaluate the sterilizing effect of different environments. Bacillus subtilis subsp. niger is one of the most common spore-forming strains and has the strongest resistance to the sanitizer among different types of pathogens. This strain has been used as a biological indicator in several evaluations of disinfection efficiency (Li et al., Citation2012; Jeng and Woodworth, Citation1990; Pottage et al., Citation2012). Furthermore, B. subtilis subsp. niger spores also can be used as an avirulent surrogate for B. anthracis spores because of the similar resistance to decontaminants. (Rogers et al., Citation2005, Citation2007).
Response surface methodology (RSM) is a collection of statistical and methodology techniques useful for developing, improving, and optimizing processes in which a response of interest is influenced by several variables (Baş and Boyacı, Citation2007). A predictive model for the inactivation of E. coli O157:H7 on green peppers by ClO2 gas using RSM was built in a previous study (Han et al., Citation2001), but this model was primarily applied to foodborne pathogens. Furthermore, the inactivation of spores in an enclosed space has not yet been described by a mathematical model.
Therefore, the objectives of this study were to investigate the individual effects and interactions of the ClO2 gas concentration (1–3 mg/L), RH (30–70%), and exposure time (30–90 min) on the inactivation of B. subtilis subsp. niger spores in an enclosed space and to develop a predictive model using RSM for determining appropriate treatment conditions to inactivate spores. The predictive model could illustrate the effect tendency of important factors in the ClO2 gas treatment and provide a reference to apply ClO2 gas to decontaminate environments.
Materials and methods
Spore preparation and coupon inoculation
Stock cultures of B. subtilis subsp. niger (ATCC 9372) were obtained from the China General Microbiological Culture Collection Center (Beijing, China) for this study. Spores were prepared according to the slightly modified standard method (Aouadhi et al., Citation2013). Cultures were grown in tryptic soy broth on a rotary shaker (HNY-2102C; Honour, Tianjin, China) at 37 °C for 18–24 hr to obtain a working culture. A aliquot (1 mL) of working culture was spread on sporulation agar plates (18 g/L nutrient broth, 15 g/L agar, 8 mg/L MnSO4·H2O, and 1 g/L CaCl2·H2O) and incubated at 37 °C for 7 days. Spores were gently dislodged from the plate using a sterile L-shape spreader and collected in a sterile centrifuge tube. The spores were washed three times with sterile distilled water and treated at 65 °C for 30 min to kill the vegetative cells. A spore population of >90% was verified via a spore stain and microscopic analysis. The spore stock was diluted to a final concentration of approximately 1 × 109/mL and was stored at 4 °C.
As required, the samples of spore stock (2 mL) were diluted in a measured volume of sterile distilled water to obtain a spore suspension with a concentration of 5 × 108/mL. Each sterile filter paper coupon (1 cm × 1 cm; type 103; Jiaojie, Shenyang, China) was inoculated with 10 μL of this suspension. After inoculation, the coupons were dried in a laminar flow biosafety hood for 1 hr at 23 °C. The prepared coupons were used within 24 hr of inoculation.
Test chamber
The inactivation experiments were conducted in a chamber (length × width × height, 1.25 m × 0.8 m × 1 m) equipped with a door, gloves, injection ports, and connection for electricity. A pass box was connected to the chamber. Four electric fans (HSL-2; Emicorcom, Leqing, China) were installed in the corner of the chamber to mix the fumigant. To maintain the desired RH during the exposure time, water vapor was injected into the chamber via an ultrasonic humidifier (PW103; Povos, Shanghai, China). A sensor (CHT3W1TLD; Honeywell, Morris Plains, NJ, USA) was placed inside the chamber to measure the temperature and RH. The structure of the test chamber is shown in .
ClO2 gas generation and measurement
Because ClO2 gas is difficult to store and transport, it should be generated and used on site. In this study, ClO2 gas was generated and injected into the test chamber using a ClO2 gas generating system (Lab-01; National Bio-Protection Engineering Center, Tianjin, China) to obtain the desired gas concentration. A diaphragm pump (N86KTE; KNF Inc., Freiburg, Germany) was used to circulate the ClO2 gas and air mixture inside the chamber, and a concentration sensor of ClO2 gas (AF26F; Optex Inc., Tokyo, Japan) in the recirculation line continuously monitored the concentration of ClO2 gas.
ClO2 gas treatment
All experiments were repeated three times using three samples per experiment for a total of nine data points per inactivation treatment. In each treatment, three coupons were individual placed into different sealed and sterile centrifugal tubes (50 mL; Corning Inc., Corning, NY, USA). Another coupon was retained as positive control outside the chamber. The sealed centrifugal tubes and three sterile plates were placed inside the chamber within reach of the glove ports. The B. subtilis subsp. niger spores was then inactivated at varying gas concentrations (1–3 mg/L), RH (30–70%), and exposure times (30–90 min). The temperature in the test chamber was constant at 22–24 °C.
To achieve the expected experimental conditions, the humidifier and generator were used to transfer water vapor and ClO2 gas into the chamber. When the desirable RH, ClO2 gas concentration and temperature were stabilized as designed, the centrifugal tubes were opened, exposing the coupons and starting the exposure period. Because there was a slow decay of the ClO2 gas during the exposure period, the ClO2 gas was intermittently added to ensure that the gas concentration kept constant in the treatment. At the predetermined time point, the coupons were placed into sterile plates using sterile forceps, and the plates were then removed via a pass box to a biological safety cabinet in order to extract the spores. The chamber was aerated with air after all of the coupons had been removed.
Enumeration of viable spores
The number of viable spores in the untreated and ClO2 gas–treated coupons was determined using the pour plate method. The test coupons and the untreated control coupons were aseptically placed into sterile tubes containing 10 mL of phosphate-buffered saline (PBS), 0.1% Tween 80, and a magnetic stir bar. The tubes were stirred (3 min) on a magnetic stirrer (RH basic; IKA Inc., Staufen, Germany) until the filter paper had disintegrated into pulp to aid the removal of spores from the coupons. The tubes containing the coupons were then vortexed (1 min) at a speed of 1000 r/min (MS1 Minishaker; IKA).
Serial dilutions (1 in 10) to 10−4 were prepared using sterile water. Aliquots (1 mL) of the appropriate dilution were pipetted and spread on nutrient broth agar plates in duplicate. These plates were incubated for 24 hr at 37 °C, and the colonies were then enumerated. Inactivation was expressed as a logarithmic viability reduction, log(N0/N), where N and N0 are the colony counts after sterilization and in the untreated coupons, respectively. The average number of colony-forming units (CFU) from positive controls was measured to be 5.13 × 106 per coupon.
Design of experiments
The results of previous experiments showed that the ClO2 gas concentration, RH, and exposure time were the most significant factors to influence the inactivation of microorganisms by ClO2 gas (Han et al., Citation2001, Citation1999). In this study, single-factor experiments were designed to express the individual effects of the ClO2 gas concentration (1–3 mg/L), RH (30–70%), and exposure time (30–90 min) on the log reduction of B. subtilis subsp. niger spores, respectively. Each single-factor experiment consisted of five levels of the experimental factor, and other nonexperimental factors were held at the middle value of the range studied. Based on the single-factor experiments, a Box-Behnken experimental design (Ferreira et al., Citation2007) with three independent factors was used to study the inactivation of B. subtilis subsp. niger spores by ClO2 gas. Specifically, the factors investigated were the ClO2 gas concentration, RH, and exposure time, which were designated as X1, X2, and X3, respectively. Each factor was examined at three coded levels, which are indicated by −1, 0, and +1, as shown in . The real values of the independent variables were coded according to eq 1:
Table 1. Codes and levels of variables selected for the trials.
where Xi is the real value of the independent variable; xi is the coded value of the variable Xi; X0 is the value of Xi at the center point, and ΔX is the step change. X and x values are shown in .
In the next step, the inactivation behavior of spores is explained by the second-order polynomial equation as follows:
where Y is the predicted response, which is the log reduction of B. subtilis subsp. niger spores (log(N0/N)); B0, Bi, Bij, and Bii are constant coefficients of intercept, linear, interaction, and quadratic terms, respectively; xi and xj are coded independent variables.
The software Design Expert (version 8.0.6; Stat-ease Inc., Minneapolis, MN, USA) was used for the analysis of variance (ANOVA) and estimation of the response surface with a multiple linear regression. The fit of the predictive model was assessed based on the adjusted coefficient of determination (R2adj), and its statistical significance was checked by the Fisher’s F test in the same program. The three-dimensional graphical representation of the system behavior, called the response surface, was used to describe the interaction effects of the variables on the log reduction of spores.
Results
Single-factor experiments
The susceptibility of B. subtilis subsp. niger spores in the test chamber was tested by varying the ClO2 gas concentration, RH, and exposure time according to the design of single-factor experiments. The spore viability decreased (log reduction increased) as the ClO2 gas concentration increased from 1 to 3 mg/L at a constant RH of 50% and exposure time of 60 min, as shown in . This finding suggested a positive correlation between the degree of inactivation and the gas concentration. Specifically, the highest log reduction of 5.06 was observed at 3 mg/L.
Figure 2. Individual effect of ClO2 gas concentration on the inactivation of B. subtilis subsp. niger spores using ClO2 gas. RH = 50%; exposure time = 60 min.
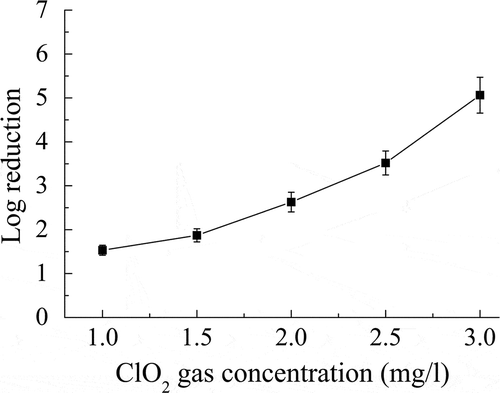
As shown in , the inactivation of B. subtilis subsp. niger spores increased as the RH was increased from 30% to 70% at a ClO2 gas concentration of 2 mg/L and an exposure time of 60 min. The maximum log reduction of 4.86 was achieved at 70% RH.
Figure 3. Individual effect of RH on the inactivation of B. subtilis subsp. niger spores using ClO2 gas. Gas concentration = 2 mg/L; exposure time = 60 min.
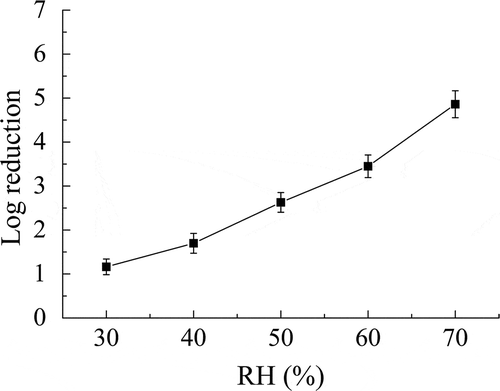
shows that the exposure time of the spores to the ClO2 gas affected the spore viability. In this study, exposure times of 30–90 min were examined, and the highest log reduction value of 4.42 of B. subtilis subsp. niger spores was obtained for an exposure time of 90 min at a gas concentration of 2 mg/L and RH of 50%.
Predictive model
presents the results of varying the ClO2 gas concentration, RH, and exposure time on the inactivation of B. subtilis subsp. niger spores in enclosed space by ClO2 gas, using RSM. The response (Y) was the log reduction of this organism.
Table 2. Box-Behnken experimental design and data for inactivation of B. subtilis subsp. niger spores by ClO2 gas using RSM.
Using a multiple regression analysis of the experimental data (), the response variables and test variables were related by the following second-order polynomial equation:
where Y is the predicted log reduction of spores, x1, x2, and x3 are coded terms for three independent variables (viz., the ClO2 gas concentration, RH, and exposure time).
The regression coefficients in eq 3 were calculated and tested for their significance using Design Expert, and are shown in . The positive signs of regression coefficients indicate the positive effect on the predicted response Y. The P value at the confidence level of α = 0.05 is used as a standard to assess the significance of each coefficient for being different from zero. The linear coefficients (B1, B2, and B3), quadratic coefficients (B11), and interaction coefficients (B12 and B23) were significant, as indicated by small P values (P < 0.05). The other term coefficients (B13, B22, and B33) were not significant.
Table 3. Regression coefficients of the predictive model.
presents a summary of the ANOVA for the predictive model. The results show that the predictive model was highly significant, as was evident from the Fisher’s F test (F = 230.84) with a very low probability value (P < 0.0001). The goodness of fit of the model was checked by the adjusted determination coefficient (R2adj). The value was 0.992, which was close to 1, indicating a satisfactory adjustment of the model to the experimental data. The large P value for lack of fit (>0.05) shows that the the F test was insignificant, implying significant model correlation between the variables and responses.
Table 4. Analysis of variance (ANOVA) for the predictive model.
Response surfaces
The graphical representations of the regression eq 3 were obtained using the Design Expert. In –, the response surfaces and contour plots show the effect of the ClO2 gas concentration, RH, and exposure time on the inactivation of B. subtilis subsp. niger spores in an enclosed space, and the contour lines in contour plots expressed the values of log reduction. In each figure, one factor remains constant at its optimal value determined by the design of experiments. A 6-log reduction of spores, which could reach the recommended sterility assurance level, was regarded as a criterion of the effective sterilization in this study (Bozkurt et al., Citation2014).
Figure 5. Interaction effects of ClO2 gas concentration and RH on the inactivation of B. subtilis subsp. niger spores using ClO2 gas while the exposure time was 60 min. (a) Contour plots of log reduction; (b) response surface.
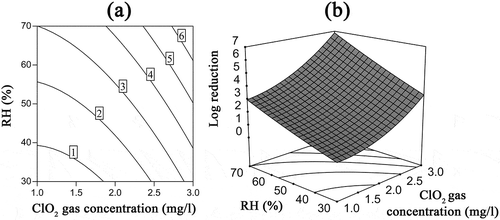
Figure 6. Interaction effects of ClO2 gas concentration and exposure time on the inactivation of B. subtilis subsp. niger spores using ClO2 gas while the RH was 50%. (a) Contour plots of log reduction; (b) response surface.
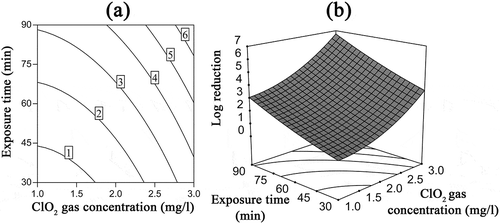
Figure 7. Interaction effects of exposure time and RH on the inactivation of B. subtilis subsp. niger spores using ClO2 gas while the ClO2 gas concentration was 2 mg/L. (a) Contour plots of log reduction; (b) response surface.
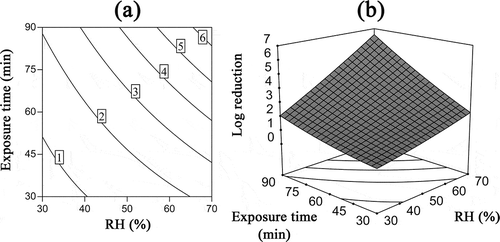
shows the effect of the ClO2 gas concentration and RH for a median exposure time (60 min, 0 level). Specifically, the log reduction of B. subtilis subsp. niger spores directly correlated with the ClO2 gas concentration and RH. The model predicted a 6-log reduction when the gas concentration was in the range of 2.7–3 mg/L, and the RH was in the range of 60–70%.
The interaction of the ClO2 gas concentration with the exposure time at a constant RH (50%, 0 level) is shown in . The contour plot indicates that the reduction of B. subtilis subsp. niger spores increases with the increase in ClO2 gas concentration and time. Meanwhile, the treatment at concentration of 2.75–3 mg/L and exposure time of 75–90 min was estimated to reach a 6-log reduction based on the model.
shows the log reduction of spores for varying exposure times and RH values at fixed ClO2 gas concentration (2 mg/L, 0 level). Specifically, exposure times of 82–90 min and RH of 65–70% were predicted to result in a 6-log reduction of spores.
Validation of the model
To validate the accuracy of the predictive model, eight additional experimental parameters were tested in the range of the Box-Behnken design. The conditions and results of the experiments are shown in . The experimental values were found to be significantly in agreement with the predicted values (average absolute relative deviation = 5.43%). Therefore, the model reliably predicted the inactivation of B. subtilis subsp. niger spores by ClO2 gas in an enclosed space for gas concentrations of 1–3 mg/L, RH of 30–70%, and exposure times of 30–90 min.
Table 5. Verified results of the predictive model.
Discussion
In this study, the inactivation of B. subtilis subsp. niger spores by ClO2 gas in an enclosed spaces was examined as a function of controlled ClO2 gas concentration, RH value, and exposure time, and a predictive model using RSM was developed based on these experimental data.
An analysis of the individual effect of the ClO2 gas concentration on the inactivation of spores () demonstrated that the log reduction of spores increased as the concentration increased from 1 to 3 mg/L. This finding is consistent with the previous work (Jeng and Woodworth, Citation1990), in which the log reduction of B. subtilis subsp. niger spores were 6.2, 5.9, and 4.0 when exposed to ClO2 gas concentrations of 30, 15, and 7 mg/L for 30 min, respectively. The indoor disinfection by ClO2 gas requires an appropriate range of concentration, considering the safety, operability, and efficiency. Low concentration leads to a slow inactivation rate and long treatment time, whereas excessive concentration brings strong causticity and high risk of operation. The range of concentration in this study is moderate and in accordance with the practical application in various enclosed spaces (Pottage et al., Citation2012; Lowe et al., Citation2013).
RH plays an important role in the inactivation of microorganisms by ClO2 gas. The inactivation tendency of ClO2 gas according to different levels of RH is shown in . High level of RH leads to a high log reduction of B. subtilis subsp. niger spores. Several studies also obtained an efficient inactivation under conditions of high RH (>70%) (Wood and Martin, Citation2009; Luftman et al., Citation2006; Bhagat et al., Citation2010), whereas the disinfection effect of ClO2 gas under low RH (<50%) is less mentioned. In the present study, different levels of RH were used to reflect the influence on the spore inactivation, and there was a nonlinear increase of the log reduction as the RH values increased from 30% to 70%. The rate of killing under high RH was faster than under low RH. Therefore, humidifying the environment to a high level of RH will accelerate the disinfection process by ClO2 gas.
Exposure time is a common factor that can influence the degree of inactivation. In , the increase of exposure time also reduced the number of B. subtilis subsp. niger spores. The disinfection by ClO2 gas is a complex process that is affected by several factors. The single-factor experiments in which the ClO2 gas concentration, RH, and exposure time were varied did not demonstrate interactions between different factors and predict a treatment condition that results in complete inactivation of B. subtilis subsp. niger spores. Therefore, RSM, an empirical modeling technique used to describe the individual effects of variables and the mutual interactions between variables, was employed in this study.
The RSM analysis (eq 3; – and –) demonstrated the influence and interaction of the three controlled parameters on the inactivation of B. subtilis subsp. niger spores using ClO2 gas. All the linear coefficients, B1, B2, and B3, of the predictive model were significant (P < 0.001) and greater than zero in , confirming that these parameters all significantly and positively correlated with the inactivation of spores. Because of the descending order of the linear coefficients: B1 (1.67) > B2 (1.51) > B3 (1.42), the ClO2 gas concentration most significantly affected the inactivation, followed by the RH and exposure time in the respective ranges studied.
The interaction between the ClO2 gas concentration and RH indicated a synergistic effect on the inactivation of spores due to the significance (P < 0.05) and positive sign of the interaction coefficient B12 in . This phonemonon means that the combination of the gas concentration and RH has an additional and positive effect on the log reduction of spores except the individual effects of experimental factors. This considerable interaction could also verified by the nonlinear nature of the response surface () and the respective contour plots () (Yetilmezsoy et al., Citation2009). Several studies have also indicated that the combination of the ClO2 gas concentration and RH represents a synergistic effect (Park and Kang, Citation2015; Han et al., Citation2001). This synergistic effect may be attributed to the following: Water vapor may condense onto the surface to surround the spores under high RH conditions (Pottage et al., Citation2012). Due to the high solubility of ClO2 gas in water, the condensed water likely acts as the carrier of ClO2 gas. Thus, the combination of high gas concentration and high RH will allow more ClO2 act on the spores and lead to a rapid improvement in the inactivation efficiency.
The analysis of the model also shows that the RH and exposure time exert a synergistic effect on the inactivation of spores. The interaction coefficient B23 (0.72) in was significantly different from zero (P < 0.05) and greater than the coefficient B12 (0.26), indicating a strong interaction between the RH and exposure time. When both the RH and exposure time increased, the huge increase in the log reduction in the response surface () and the decrease in the spacing of the contour lines () also verified the synergistic effect. The reason for this result may be as follows: Bacterial spores are highly resistant to disinfectants because of thick and firm spore coats, which protect the contents of spores from adverse environmental conditions (McKenney and Eichenberger, Citation2012). Increases in RH and time leads to spores swell and the expansion of the channels used by gases to access the spores (Westphal et al., Citation2003). Therefore, the combination of high RH and long exposure time will provide more effective exposure of ClO2 gas to spores and exert a positive effect on the inactivation of B. subtilis subsp. niger spores.
The predictive model based on the RSM in this study can be used to illustrate the effect tendency and interactions of these experimental factors as well as predict a condition of ClO2 gas treatment to achieve a 6-log reduction of B. subtilis subsp. niger spores according to the response surfaces and contour plots in –. Filter paper strip is one of the most common carrier types and is widely used for the validation of space decontamination (Jeng and Woodworth, Citation1990; Jia et al., Citation2013). However, materials of the spore carrier greatly affected the inactivation efficiency (Li et al., Citation2012; Rogers et al., Citation2007). It is important to note that the predicted results are based on a certain carrier of spores and that use of different indoor surface materials and/or treatment conditions may result in altered inactivation characteristics. Therefore, the predictive model only provided a reference for the ClO2 gas treatment and further validation of calculated treatment conditions must be carried out before actually applying a treatment to the indoor disinfection.
Conclusions
An RSM involving experimental design and regression analysis was used to reveal the influence and interaction of the ClO2 gas concentration, RH, and exposure time on the inactivation of B. subtilis subsp. niger spores in an enclosed space by ClO2 gas. A predictive model was established and verified by the experiment data. The model could be used to describe the individual effect and interaction of important factors, and to predict a ClO2 gas treatment condition to achieve a 6-log reduction of spores, which may aid the application of ClO2 gas treatments for indoor disinfection.
Funding
This work was supported by the National Science and Technology Major Project of China (No. 2012ZX10004801) and the National High Technology Research and Development Program of China (No. 2014AA021405).
Additional information
Funding
Notes on contributors
Jiancheng Qi
Tao Wang is a doctoral candidate, Jiancheng Qi is a research professor, Jinhui Wu is an associate professor, Limei Hao, Ying Yi, Song Lin, and Zongxing Zhang are research assistants at the Institute of Medical Equipment, Academy of Military Medical Sciences, and the National Bio-protection Engineering Center in Tianjin, People’s Republic of China.
References
- Aieta, E.M., and J.D. Berg. 1986. Review of chlorine dioxide in drinking water treatment. J. Am. Water Works Assoc. 78:62–72.
- Althaus, C.L., N. Low, E.O. Musa, F. Shuaib, and S. Gsteiger. 2015. Ebola virus disease outbreak in Nigeria: Transmission dynamics and rapid control. Epidemics 11:80–84. doi:10.1016/j.epidem.2015.03.001
- Aouadhi, C., H. Simonin, H. Prévost, M. Lamballerie, A. Maaroufi, and S. Mejri. 2013. Inactivation of Bacillus sporothermodurans LTIS27 spores by high hydrostatic pressure and moderate heat studied by response surface methodology. LWT Food Sci. Technol. 50:50–56. doi:10.1016/j.lwt.2012.07.015
- Aygun, G., O. Demirkiran, T. Utku, B. Mete, S. Urkmez, M. Yilmaz, H. Yasar, Y. Dikmen, and R. Ozturk. 2002. Environmental contamination during a carbapenem-resistant Acinetobacter baumannii outbreak in an intensive care unit. J. Hosp. Infect. 52:259–262. doi:10.1053/jhin.2002.1300
- Baş, D., and İ.H. Boyacı. 2007. Modeling and optimization I: Usability of response surface methodology. J. Food Eng. 78:836–845. doi:10.1016/j.jfoodeng.2005.11.024
- Bhagat, A., B.S. Mahmoud, and R.H. Linton. 2010. Inactivation of Salmonella enterica and Listeria monocytogenes inoculated on hydroponic tomatoes using chlorine dioxide gas. Foodborne Pathog. Dis. 7:677–685. doi:10.1089/fpd.2009.0466
- Bozkurt, H., D.H. D’Souza, and P.M. Davidson. 2014. A comparison of the thermal inactivation kinetics of human norovirus surrogates and hepatitis A virus in buffered cell culture medium. Food Microbiol. 42:212–217. doi:10.1016/j.fm.2014.04.002
- Ferreira, S.L., R.E. Bruns, H.S. Ferreira, G.D. Matos, J.M. David, G.C. Brandao, E.G. Silva, L.A. Portugal, P.S. Reis, A.S. Souza, and W.N. Santos. 2007. Box-Behnken design: An alternative for the optimization of analytical methods. Anal. Chim. Acta. 597:179–186. doi:10.1016/j.aca.2007.07.011
- Goyal, S.M., Y. Chander, S. Yezli, and J.A. Otter. 2014. Evaluating the virucidal efficacy of hydrogen peroxide vapour. J. Hosp. Infect. 86:255–259. doi:10.1016/j.jhin.2014.02.003
- Han, Y., J.D. Floros, R.H. Linton, S.S. Nielsen, and P.E. Nelson. 2001. Response surface modeling for the inactivation of Escherichia coli O157: H7on green peppers (Capsicum annuum L.) by chlorine dioxide gas treatments. J. Food Prot. 64:1128–1133.
- Han, Y, A.M. Guentert, R.S. Smith, R.H. Linton, and P.E. Nelson. 1999. Efficacy of chlorine dioxide gas as a sanitizer for tanks used for aseptic juice storage. Food Microbiol. 16:53–61. doi:10.1006/fmic.1998.0211
- Han, Y., R.H. Linton, S.S. Nielsen, and P.E. Nelson. 2001. Reduction of Listeria monocytogenes on green peppers (Capsicum annuum L.) by gaseous and aqueous chlorine dioxide and water washing and its growth at 7 degrees C. J. Food Prot. 64:1730–1738.
- Hsu, C.S., M.C. Lu, and D.J. Huang. 2014. Effect of gaseous chlorine dioxide on student cafeteria bioaerosols. Clean Soil Air Water 42:12–19. doi:10.1002/clen.201100293
- Hudson, J.B., M. Sharma, and M. Petric. 2007. Inactivation of Norovirus by ozone gas in conditions relevant to healthcare. J. Hosp. Infect. 66:40–45. doi:10.1016/j.jhin.2006.12.021
- Jeng, D.K., and A.G. Woodworth. 1990. Chlorine dioxide gas sterilization under square-wave conditions. Appl. Environ. Microbiol. 5:514–519. doi:10.1111/aor.1990.14.issue-5
- Jia, H.Q., Y.J. Li, B. Sun, S.Q. Zhao, Y. Yi, M. Zhao, Z.X. Zhang, X. Pan, and J.C. Qi. 2013. Evaluation of vaporized hydrogen peroxide fumigation as a method for the bio-decontamination of the high efficiency particulate air filter unit. Biomed. Environ. Sci. 26:110–117. doi:10.3967/0895-3988.2013.02.005
- Li, Y.J., N. Zhu, H.Q. Jia, J.H. Wu, Y.Yi, and J.C. Qi. 2012. Decontamination of Bacillus subtilis var. niger spores on selected surfaces by chlorine dioxide gas. J. Zhejiang Univ. Sci. B 13:254–260. doi:10.1631/jzus.B1100289
- Lowe, J.J., S.G. Gibbs, P.C. Iwen, and P.W. Smith. 2012. A case study on decontamination of a Biosafety Level-3 laboratory and associated ductwork within an operational building using gaseous chlorine dioxide. J. Occup. Environ. Hyg. 9:196–205. doi:10.1080/15459624.2012.733592
- Lowe, J.J., S.G. Gibbs, P.C. Iwen, P.W. Smith, and A.L. Hewlett. 2013. Impact of chlorine dioxide gas sterilization on nosocomial organism viability in a hospital room. Int. J. Environ. Res. Public Health 10:2596–605. doi:10.3390/ijerph10062596
- Lowe, J.J., A.L. Hewlett, P.C. Iwen, P.W. Smith, and S.G. Gibbs. 2013. Evaluation of ambulance decontamination using gaseous chlorine dioxide. Prehosp. Emerg. Care 17:401–408. doi:10.3109/10903127.2013.792889
- Luftman, H.S., M.A. Regits, P. Lorcheim, M.A. Czarneski, T. Boyle, H. Aceto, B. Dallap, D. Munro, and K. Faylor. 2006. Chlorine dioxide gas decontamination of large animal hospital intensive and neonatal care units. Appl. Biosaf. 11:144–154. doi:10.1177/153567600601100306
- McKenney, P.T., and P. Eichenberger. 2012. Dynamics of spore coat morphogenesis in Bacillus subtilis. Mol. Microbiol. 83:245–260. doi:10.1111/j.1365-2958.2011.07936.x
- Morino, H., T. Fukuda, T. Miura, C. Lee, T. Shibata, and T. Sanekata. 2009. Inactivation of feline calicivirus, a norovirus surrogate, by chlorine dioxide gas. Biocontrol Sci. 14:147–153. doi:10.4265/bio.14.147
- Park, S.H., and D.H. Kang. 2015. Antimicrobial effect of chlorine dioxide gas against foodborne pathogens under differing conditions of relative humidity. LWT Food Sci. Technol. 60:186–191. doi:10.1016/j.lwt.2014.09.031
- Petersen, E., D.S. Hui, S. Perlman, and A. Zumla. 2015. Middle East respiratory syndrome—Advancing the public health and research agenda on MERS—Lessons from the South Korea outbreak. Int. J. Infect. Dis. 36:54–55. doi:10.1016/j.ijid.2015.06.004
- Pottage, T., S. Macken, K. Giri, J.T. Walker, and A.M. Bennett. 2012. Low-temperature decontamination with hydrogen peroxide or chlorine dioxide for space applications. Appl. Environ. Microbiol. 78:4169–4174. doi: 10.1128/AEM.07948-11.
- Rogers, J.V., Y.W. Choi, W.R. Richter, D.C. Rudnicki, D.W. Joseph, C.L. Sabourin, M.L. Taylor, and J.C. Chang. 2007. Formaldehyde gas inactivation of Bacillus anthracis, Bacillus subtilis, and Geobacillus stearothermophilus spores on indoor surface materials. J. Appl. Microbiol. 103:1104–1112. doi:10.1111/j.1365-2672.2007.03332.x
- Rogers, J.V., C.L. Sabourin, Y.W. Choi, W.R. Richter, D.C. Rudnicki, K.B. Riggs, M.L. Taylor, and J.C. Chang. 2005. Decontamination assessment of Bacillus anthracis, Bacillus subtilis, and Geobacillus stearothermophilus spores on indoor surfaces using a hydrogen peroxide gas generator. J. Appl. Microbiol. 99:739–748. doi:10.1111/j.1365-2672.2005.02686.x
- Smith, R.D. 2006. Responding to global infectious disease outbreaks: Lessons from SARS on the role of risk perception, communication and management. Soc. Sci. Med. 63:3113–3123. doi:10.1016/j.socscimed.2006.08.004
- U.S. Environmental Protection Agency. 2006. Pesticides—Reregistration Eligibility Decision (RED) for chlorine dioxide (including sodium chlorite). http://www3.epa.gov/pesticides/chem_search/reg_actions/reregistration/red_PC-020503_3-Aug-06.pdf ( accessed September 16, 2015).
- Vaid, R., R.H. Linton, and M.T. Morgan. 2010. Comparison of inactivation of Listeria monocytogenes within a biofilm matrix using chlorine dioxide gas, aqueous chlorine dioxide and sodium hypochlorite treatments. Food Microbiol. 27:979–984. doi:10.1016/j.fm.2010.05.024
- Westphal, A.J., P. B. Price, T.J. Leighton, and K.E. Wheeler. 2003. Kinetics of size changes of individual Bacillus thuringiensis spores in response to changes in relative humidity. Proc. Natl. Acad. Sci. U. S. A. 100:3461–3466. doi:10.1073/pnas.232710999
- Wood, J.P., and G.B. Martin. 2009. Development and field testing of a mobile chlorine dioxide generation system for the decontamination of buildings contaminated with Bacillus anthracis. J. Hazard. Mater. 164:1460–1467. doi:10.1016/j.jhazmat.2008.09.062
- Yetilmezsoy, K., S. Demirel, and R.J. Vanderbei. 2009. Response surface modeling of Pb(II) removal from aqueous solution by Pistacia vera L.: Box-Behnken experimental design. J. Hazard. Mater. 171:551–562. doi:10.1016/j.jhazmat.2009.06.035