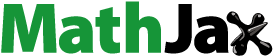
ABSTRACT
Fugitive dust is an important source of particulate matters (PM) emission in the air. Vegetation barriers (VBs) can be an effective way to mitigate PM from fugitive dust sources. It is meaningful to choose appropriate plants to establish VBs that can efficiently capture PM from various sources. This study was conducted to establish comparable and repeatable conditions to evaluate the capability of different VB species in mitigating PM emission from certain fugitive dust source. The airflow around two VBs and their PM interception mechanism was studied in a wind tunnel with simulated PM emission source of animal feeding operations. The species used for the two VBs were conifers represented by Pinus Sylvestris var. mongolica Litv. (PS) and the broad-leaved species represented by Syringa Oblate Lindl. (SOL). The results showed that the interception efficiency of the PS vegetation barrier was only slightly lower than that of SOL vegetation, while the PS had a lower effect on the wind speed at the similar leaf surface area. On the other hand, there were a large number of disordered “ridged” stripes on the microscopic structure of the hydrophilic leaves of SOL and PS, and a large amount of fine particles deposited on the leaves were observed, indicating that the microscopic geometric surface structure increased the deposition efficiency of the particles. These findings help to better understand the potential of tree species to reduce PM in environments.
Implications: To evaluate the capability of different VB species in mitigating PM emission from certain fugitive dust source, airflow around the two VBs and their PM interception mechanisms were studied in wind tunnels with comparable and repeatable conditions. The results showed that the interception efficiency of the Pinus Sylvestris var. mongolica Litv. (PS) was only slightly lower than that of the Syringa Oblate Lindl. (SOL) vegetation, while the PS had a lowering effect on the wind speed at the same low leaf surface area. Microscopic analysis of leaves surface indicated that the microscopic characteristics increased the deposition efficiency of the particles.
Introduction
Particulate matters (PM) pollution has already become one of the major environmental problems around the world (Nowak et al. Citation2013; Xie et al. Citation2018), especially in China (Zhang and Cao Citation2015), which affected air quality, regional and global climates, and human health (Brook and Rajagopalan Citation2012; Huang et al. Citation2014; Pascal et al. Citation2016; Wong et al. Citation2008). Fugitive dust (FD) is one of major contributors to the PM pollution (Zhan and Qian Citation2018). Common sources of FD include road (Amato et al. Citation2009; Zhao et al. Citation2017), agricultural operations (Bonifacio et al. Citation2015; Cambra-Lopez et al. Citation2010; Ni Citation2015), building construction (Yan et al. Citation2019), and many other natural and anthropogenic activities. Emitted dust in general can cause illnesses or death from respiratory, heart, and lung disease, especially for small particles (Anderson, Thundiyil, and Stolbach Citation2012; Colson et al. Citation2017). Implementing long-term FD mitigation plans and management strategies is needed for policy makers to improve air quality and protect environment for maximum ecological benefit.
Currently, vegetation barriers (VBs) have been proved to be an effective approach used to mitigate FD pollution (Guo et al. Citation2019; Wu et al. Citation2018; Wuyts et al. Citation2018). The VBs, with aboveground organs (especially leaves/needles) of trees and shrubs, have potential dust retaining capacity to control atmospheric dustiness (Kretinin and Selyanina Citation2006). VB, as a natural porous barrier, can reduce wind speed and change the direction of airflow by the flexible elements such as leaf, branch and twig, which can adapt to fluid fluctuations (Guo Citation2011; Lin Citation2006). Studies have also shown that VBs have a good effect on accommodating microclimate and adhering particulate matter with advantages of high efficiency and economical and facilitating management (Albayrak et al., Citation2014; Gosselin and de Langre Citation2011; Tyndall and Colletti Citation2006; Weber, Kowarik, and Saumel Citation2014). Therefore, VBs have become one of the important methods in PM pollution control, which has been widely used in various situations, such as urban streets (Doygun and Doygun Citation2018; Li et al., Citation2016a; Tong et al. Citation2016), highways (Lee et al. Citation2018; Ranasinghe et al. Citation2019), and livestock and poultry farms (Guo Citation2011). However, the mechanism of VBs effecting on the air improvement is not yet fully understood.
There are great differences in the interception efficiency of PM between different VBs (Guo Citation2011). Studies have shown that the dust retention capacity of the VBs depends on several factors, including canopy type, leaf and branch density, and leaf micromorphology (Rai et al. Citation2009; Rasanen et al. Citation2013; Saebo et al. Citation2012; Sgrigna et al. Citation2015). Available studies mostly focused on the dust on leaves exposed to general air pollution in the city, and lacked overall evaluation from the aspect of wind sheltering, PM concentration reduction in the air, and particle retention within the VBs. Moreover, due to the timeframe required to implement mature vegetation in the same location and under same meteorological conditions, for the on-site measurement experiment, the studies have been restricted by their inability to directly and precisely compare the findings with replicable conditions., such as the measurement methods, territorial environment, PM emission sources, and so on (Qi et al. Citation2013; Saebo et al. Citation2012; Terzaghi et al. Citation2013). Due to the specific characterizes of various vegetation, the efficiency and mechanism of VBs intercepting particulate pollutants from different sources still need to be further studied. It was suggested that future study may aim at the species selecting of local vegetation and VB configuration design for specified particulate emission sources in comparable experimental conditions, making effect on intercepting smaller particles (Qi et al. Citation2013; Terzaghi et al. Citation2013).
The purpose of this study was to systematically evaluate and compare the capability of two types of VBs in mitigating simulated FD from certain source (concentration animal feeding operations) in a wind tunnel. Specific objectives of this study were to 1) explore controllable experimental conditions for comparing conifers and broad-leaved species of VBs in reducing FD and 2) evaluate the PM interception efficiency of VB both from the aspect of PM concentration in the air and particles retained on the leaves of VB. This study had practical significance for reducing FDs in the atmosphere and providing a new method for the study of VBs.
Materials and methods
In this study, the experiment was carried out in an indoor low-speed wind tunnel. Two types of VBs were established and evaluated at three levels of incoming air speed. A total of 6 groups of experiments were repeated for 3 times. During the tests, the average indoor temperature was 22 ± 2 °C and the relative humidity was 16 ± 2%.
Experimental set up
The wind tunnel had a test section of 2 m in length, 1.5 m in height, and 1.2 m in width as shown in . Powder particles were dispersed at height of 0.9 m of central interface of contraction and stable section, and entered the test section with the airflow. The wind speed at the inlet of the test section was set at 0.9 m/s (v1), 1.8 m/s (v2), and 2.7 m/s (v3), which was the reference speed and measured at the center point 0 of the entrance plane. In the test section, the VBs were set perpendicular to the windward direction, and the distance between the entrance plane of the test section and the adjacent edge of VBs was 0.6 m. Taken the vertical plane at this edge as the x = 0 plane and the bottom surface of the test section as z = 0 plane, the origin (0, 0, 0) was set at the middle point of the intersection line of these two planes, and the vertical plane along the wind direction at the origin was considered as y = 0 plane.
Powder particles for dispersion
To simulate the PM emitted from concentrated animal feeding operations, a mixture of surface soil layer (include surface dust, manure, feed, and soil) from a cattle feedlot was collected and pulverized into powder particles for dispersion in the air (Tong et al. Citation2017; Uzoma et al. Citation2011).
The processing process of these samples was shown in . The collected mixture was dried at 110°C for 20 h in a constant temperature drying oven (ZK-35S, Tianjin Sanshui Scientific Instruments Co., Ltd., China), and the dried sample was cooled to room temperature in the dryer (Rogge, Medeiros, and Simoneit Citation2006). It was then pulverized with a planetary ball mill (XQM-2 L, Nanjing Laboratory of Scientific Analysis and Research, China) with a rotational speed of 500 r·min−1 and a grinding time of 3 h. Using deionized water as the medium, a small portion of the granulated powder obtained with above method was dissolved in water and ultrasonic dispersed, and the particle size distribution was tested by laser particle size distribution instrument (BT-9300ST, Dandong Bate instruments Co., Ltd., China) through wet injection system. The geometric mean particle size and middle diameter of particle powder were obtained. The total average geometric mean diameter and standard deviation of particulate matter source for the wind tunnel tests were calculated as shown in . The generated powder particles were dispersed to the wind tunnel by a particle generator (BT-901 restructuring, Dandong Bate Instruments Co., Ltd., China), continuously providing stable and uniform suspended particles for the wind tunnel tests.
Table 1. Particle size distribution of powder particles used for the dispersion for PS and SOL tests (Mean ± Standard Error)
Vegetative barriers
The young PS trees were selected from the Experimental Forest Farm of Jingyuetan, Changchun, and the SOL VBs were established with mature branches and leaves taken from the SOL trees at the campus of Jilin University. The two PS trees and the branches and leaves of SOL were transplanted and fixed in two soil boxes and floral foam bricks, respectively. Each PS tree with bundled needles was considered as cylinder shape with diameter (D) of 0.4 m and height (H) of 0.7 m. The SOL barrier was considered as rectangular with length (L) of 0.5 m, width (W) of 0.2 m, and height of 0.7 m. The leaf surface area density of PS and SOL were set closely about 6.63 m−1 and 6.56 m−1 (estimated by the following calculation method), respectively, to compare their interception efficiency for PM. During the experiment, the VBs were watered properly every day to keep them fresh. After each experiment, the VBs were washed with deionized water and used again after natural evaporation.
The estimation of PS and SOL surface area density: the total surface area (m2) of each element (leaf/needle, twig, stem and branch) of each VB was added together, divided by the volume (m3) of the VB, and the surface area density (m−1) of the VB was obtained (Tiwary, Morvan, and Colls Citation2006). Firstly, the mean surface area of each element was obtained through 100 leaves/needles and 20 branches randomly chosen from clove hedges and the same with PS plant, as shown in . The surface area of SOL leaves was calculated through their profiles in a coordinate paper (Tiwary, Morvan, and Colls Citation2006). The needles, twigs, and stems were assumed as cylinders, and the surface area was calculated with the diameter and length measured by vernier caliper (Hwang, Yook, and Ahn Citation2011). Then, the total number of each element was counted or estimated (needles) in each VB. Meanwhile, the total surface area of each element was estimated by multiplying the mean surface area and the total numbers.
Table 2. The parameters used for the calculation of leaf surface area of PS and SOL
Monitoring of air velocity and PM concentration
As a carrier of PM, air flow has a significant effect on the diffusion and dispersion of particulate matter. The velocity distribution around the barriers was measured by using a hot-wire anemometers (D8880, CEM Machinery Industry Co., Ltd., China). The wind speed at 4 points was measured in the experiment as shown , which were in the y = 0 plane. The monitored point 1 and point 3, as the closest monitor points from upwind and downwind side of VBs, respectively, were set at 0.1 m (about 0.1 H) away from VBs at height of 0.5 m (0.75 H) (Tiwary, Morvan, and Colls Citation2006). The point 2 was set up above the middle of the VBs at height of 0.9 m. The point 4 was set further downwind of the VBs at 0.9 m away from the origin at height of 0.5 m. Using two aerosol monitors (DustTrak DRX 8533, TSI, USA), the mass concentration of PM1, PM2.5, and PM10 (particulate matter with an aerodynamic diameter of 1 μm, 2.5 μm, and 10 μm or less, respectively) at point 1 and point 3 were monitored. The sampling time for each test was 4 min with 1 s interval. Before each test, the instruments were calibrated.
Microstructure of leaves and elements of particles
The microstructure of PS and SOL leaves was observed by scanning electron microscopy (SEM, EVO18, ZEISS). To detect element composition of PM, Energy-dispersive X-ray spectra (S2 PUMA, Shanghai Casting Analysis Instrument Co., Ltd., Germany) was adopted. The static contact angle of the leaf was measured using the Optical Contact Angle Meter (JC2000A, Shanghai Optoelectronics Co., China).
Data analysis
According to the real-time measured values of wind speed at two points (1 and 3), the wind speed reduction efficiency θ (%) was calculated as shown in Equation 1:
where v1 and v3 are the average wind speed at monitoring point 1 and point 3, respectively. Each test was repeated three times, and the test results were mean ± standard error (Mean ± SE). The wind speed at monitor points 1–4 were reported with normalized speed to the reference point 0.
The efficiency of the VBs on intercepting particulate matter is η (%) as shown in Equation 2:
where C1 and C3 are the average value of particulate matters concentration within 2 to 4 minutes at the monitoring point 1 and point 3, and C′1 and C′3 are background concentration within 1 min of the monitoring point 1 and point 3. The test results were mean ± standard error (Mean ± SE).
Shapiro Paired t-test was performed on the differences of PM1, PM2.5, and PM10 concentrations with significance levels less than 0.05.
Results and discussion
Distribution of air flow around VBs
Airflow distribution around Pinus Sylvestris var. mongolica Litv. (PS) and Syringa Oblate Lindl. (SOL) was reflected by varied wind speeds (). Under three wind speed condition settings, the relative wind speed at point 1 of PS and SOL VBs was 0.88, 0.89, 0.96 and 0.72, 0.74, 0.77, respectively. The wind speed of the PS barrier reached the highest velocity at the monitoring point 2, while the wind speed decreased at the other three points (). For the SOL barrier, the wind speed at point 3 reached its highest under the same conditions ().
Figure 3. Normalized velocity around the vegetation barriers of PS (a) and SOL (b) at three levels of incoming wind settings
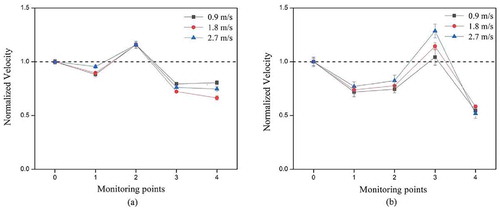
Since airflow is the carrier of the PM in the dispersing process, it is important to understand how air flows through the vegetation (Koch, Samson, and Denys Citation2019). The potential to reduce air pollution depends on the effect of vegetation barriers on airflow (Viippola et al. Citation2018). When the airflow approached the VBs, PS barrier presented greater resistance to airflow than that of SOL barrier; thus the highest wind speed occurred right above the PS barrier while it was lagged for the SOL barrier (). This might be caused by the shape and structure difference of these two VBs established in this study. Compared to PS, the SOL branches were suppler to bend with the wind, and the broad leaves easily crimping itself with airflow and leading the branches to swing (Albayrak et al., Citation2014; Alben, Shelley, and Zhang Citation2002). It was observed during the tests that the sway amplitudes of SOL top branches were great and the SOL vegetation barrier presented an inclined angle, especially in the highest wind speed. Therefore, the large deformability of plants caused large amplitudes of motion, which caused the highest speed region to lag (Langre and Emmanuel Citation2008). In addition, with similar surface area density, the SOL with larger leaf surface area had fewer leaves compared with that of PS, which became sparser and made it easier for airflow to pass through the SOL (Středová et al. Citation2012). Commonly, the wind speed above the crown is increased and the wake is tilted downwards and extends far behind a full-scale tree (Dellwik et al. Citation2019). The uncommon phenomenon occurred in the case of SOL barrier might have been caused by the lighter and more flexible structure of branches compared to that of the full-scale rigid tree with trunks and stems. The measurement of the motion of plants and the dynamics of wind, over large sets of points, and for plant systems of various sizes need to be further studied to more accurately represent the airflow distribution.
Interception efficiency of particulate matter
The interception efficiency of VBs for all particle sizes decreased with the increase of wind speed (). For PM1, PM2.5, and PM10, the interception efficiencies of PS barrier varied from 15.9% to 41.6%, 10.5% to 26.4%, and 11.6% to 36.9% (), respectively, with the wind speed varied from high to low. For the SOL barrier, those values ranged 17.5%--35.9%, 12.8%--33%, and 13.6%--31.8% (), respectively.
Figure 4. Interception efficiency of different size particles by PS (a) and SOL (b) at different wind speeds
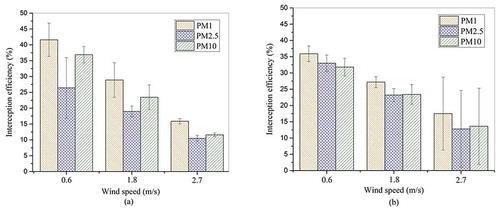
At each wind speed for PS barrier, the interception efficiency of PM1 was significantly higher than that of PM2.5 and PM10, while the interception efficiency of PM10 was higher than that of PM2.5. The PS barrier had better interception effect on PM1 maybe because a large number of needles disturbed the airflow into small vortices, which increased the turbulence intensity near the needles. This was consistent with the published study, where ultrafine particles were primarily driven by Brownian diffusion and turbulent transport (Lin and Khlystov Citation2012). As the airflow was disturbed by the PS barrier, the air flow speed was decreased and some of particles collided with the branches and needles. Because PM10 has larger particle size and larger mass, they were easy to settle due to its own gravity, resulting in higher interception efficiency of PM10 than PM2.5. In addition, the variation of PM2.5 was broader at the lowest wind speed. This might be caused by the unstable capability of PS on intercepting PM2.5 at low wind speed or the uncertainties of the experiments.
As for SOL barriers, although the interception efficiency for PM1 was higher than that of PM2.5 and PM10, the differences of the interception efficiency of the three PM size fraction were not significant (p > 0.05). The mean differences of PM concentrations between PS and SOL species were not essentially different. At the highest speed of v3, the variance of the interception efficiency of the SOL was higher. It might be caused by the great sway amplitude of SOL top leaves based on the observation, which resulted in the relatively unstable movement of the particles and the disturbance of flow on the instrument sampling.
In addition, wind speed had significant impact on the interception efficiency of PM. With the increase of wind speed, the efficiency of VB intercepting PM showed a decreasing trend. This may be due to the fact that the leaves and stems of the VB can flexibly adjust their direction as the wind speed changed, thus changing the local structure and porosity of the VB, resulting in the particles to pass through the VB quickly.
Influences of leaf microstructure on the reduction of particulate matter
The microscopic structure of PS needles showed a well-organized “ridged” stripe with 8–10 μm width and an ordered array of stomata-strips distributed with a number of stomata evenly. Distribution of villi was observed in the area between the stomata, and the stoma diameter was 16 μm with spacing of 43 to 61 μm (). A large number of particles were deposited between the stomata-strips () and between striped bands () of the needles. The needle was more likely to accumulate fine particles with particle size less than 10 μm (). This was due to the large mass of wrinkles and the villus structure distributed in the area of the stomata zone. Also, the size of the villus was nanometer-scale, resulting in increased surface area and surface roughness, which was more conducive to the deposition of intercepting particles. However, for the needles, it can be found that the striped bands accumulated fewer particles than on the stomata strips, which might be because the surface of the striped strip had a smoother surface.
Figure 5. Representative scanning electron micrographs showing the particles on the stomata strips (a) and striped bands (c) of PS needles; (b) and (d) correspond to the square areas on (a) and (c), respectively, with higher magnification
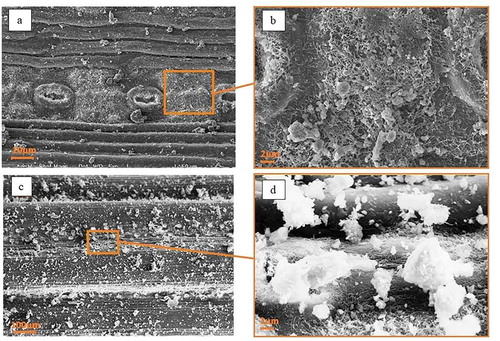
The SOL leaves were symmetrical in shape with a main vein. There were a large number of disordered “ridged” stripes distributed around the main and secondary main veins (). A tree-like hierarchical structure was formed, which is a very typical structure with a large extended area surface in biological research fields (Li et al., Citation2016b). Plenty of deposited fine particles (less than 10 μm) were observed in the vicinity of the main vein (), and many coarse particles (more than 10 μm) deposited in the area between the main vein and the leaf edge (). The microscopic structure of the SOL leaves significantly affected the deposition efficiency and particle size, which is consistent with the previous research conclusions (Tong et al. Citation2017; Tsutsui et al. Citation2016). The stripes and gullies of the SOL leaves could increase the deposition efficiency of the particles, since the dense micro-nano secondary structure facilitated the capture of PM due to its ability to provide a large contact surface area and high roughness to block airflow.
Figure 6. Representative scanning electron micrographs showing the particles on the main vein of leaves (a) and between the main vein and the leaf edge of SOL leaves (c); (b) and (d) correspond to the square areas on (a) and (c), respectively, with higher magnification
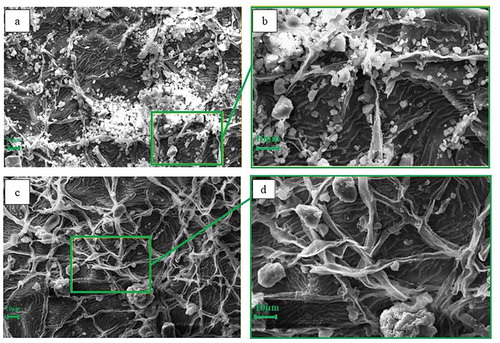
The microstructure of SOL surface was more complicated than that of PS, and the roughness of SOL was higher on high magnification. However, the particles above the needles were more than that on the SOL leaves, which may be caused by other reasons. Therefore, a leaf with a rough leaf surface is not the only indicator when capturing PM because it is affected by multiple factors.
Elemental analysis of particulate matter
To identify the PM composition affecting on particulate collection, Energy-dispersive X-ray spectra (EDX) was adopted to detect element composition of PM. As shown in , the atom content of C, N, O, Si, Al, Na and S was confirmed with 85.65%, 0.31%, 11.28%, 2.33%, 0.21%, 0.21%, and 0.01%, respectively. Herein, the C, N, O were mainly from organics in cattle manure and feed, and the elements of Si, Al, Na, S were mainly from oxides and mineral salts in soil (Rogge, Medeiros, and Simoneit Citation2006). In general, more soil consisting of oxides and mineral salts were contained in PM compared to organic manure and feed (Suriadikusumah et al. Citation2013). The results suggested that PM werer mainly composites of mineral salts, oxides, and organics.
Table 3. Percentage of element concentration in the leaf surface of the deposited particulate matter
Above all, PM prepared in this work tend to be hydrophilic as they consist of lots of mineral salts, and thus the surfaces of VB with hydrophily should be more attractive to collecting PM (Cambra-Lopez et al. Citation2010). On the contrary, PM from concentrated animal operations such as hen houses with more organics and lipoids tend to be lipophilic, leading to higher collecting efficiency by VB with lipophilic surfaces (Wei et al. Citation2015). Therefore, composition of PM was one of the factors affected the interception efficiency of VB, and the characteristics of VB leaf surfaces should be taken into account for PM mitigation from varied emission sources.
Surface wettability analysis
The average contact angle of the front side of the PS needle was 51.6°±1.9°, and the contact angle of the SOL leaves with an average value of 58.9 ° ± 4.0 °. They were between 40° and 90° belonging to hydrophilic surfaces.
The surface wettability of the leaves has a significant effect on its dust retention ability. Wettable leaves have strong dust retention ability (Fogg Citation1947; Jia, Huixia, and Binze Citation2015). The contact angle and the amount of dust retention presented negative correlation, so the hydrophilic surface is more conducive to the deposition of particulate matter. Although the surface contact angles of PS and SOL were between 40° and 90°, the contact angle of PS was smaller than that of SOL, indicating PS was more hydrophilic and deposited more particles (Fogg Citation1947).
In general, the VBs of PS and SOL presented similar capacity of intercepting particles, and both of them had greater interception efficiency at relatively lower wind speed. With the repeatable and comparable conditions in the wind tunnel, the VBs with different species can be evaluated from the aspect of wind speed reduction, PM concentration in the air, and particles deposited on the leaves/needles. However, the limitation of plant size in the wind tunnel may increase some experiment uncertainties and cause some differences with the real plant applied on site. It also needs more test cases and experimental samples to obtain reliable speculations.
Conclusion
In this study, the experiment of VBs intercepting particles was carried out in an indoor low-speed wind tunnel, which provided repeatable and comparable conditions. The air distribution, interception efficiency, and leaf surface microscopic analysis of conifers represented by PS and broad-leaved species represented by SOL were systematically studied. The following conclusions could be drawn:
The PS had stronger impact on the airflow in the case of low leaf surface area density, because the larger leaves of SOL were more likely to change the flow field around the VB under the wind. The interaction of wind and the plant organ need to be further studied to better understand the effects of wind on plants.
With the increase of wind speed, the interception efficiency of VBs for all particle sizes was reduced; the two VBs had higher interception efficiency for fine particles; the interception efficiency of PS was higher than that of SOL for PM1 and PM10.
The surface of PS and SOL leaves were rough with more “ridge” stripes, which had a significant impact on intercepting PM. The PS and SOL leaves were hydrophilic, which made the particles easier to deposit on the leaves.
The PM collected from the cattle farm was mainly a composite of mineral salts, oxides, and organics, which were hydrophilic, and thus the surfaces of VB with hydrophily should be more attractive to collecting the PM.
Additional information
Funding
Notes on contributors
Li Guo
Li Guo is an associate professor at Jilin University, People’s Republic of China.
Bo Zhao
Bo Zhao and Dongsen Zhao are master students at Jilin University, People’s Republic of China.
Jin Li
Jin Li is an undergraduate student at Jilin University, People’s Republic of China.
Jin Tong
Jin Tong is a professor at Jilin University, People’s Republic of China.
Zhiyong Chang
Zhiyong Chang is a senior engineer at Jilin University, People’s Republic of China.
Xin Liu
Xin Liu is an assistant professor at Jilin University, People’s Republic of China.
References
- Albayrak, I., V. Nikora, O. Miler, and M. T. O’Hare. 2014. Flow–plant interactions at leaf, stem and shoot scales: Drag, turbulence, and biomechanics. Aquat. Sci. 76 (2):269–94. doi:10.1007/s00027-013-0335-2.
- Alben, S., M. Shelley, and J. Zhang. 2002. Drag reduction through self-similar bending of a flexible body. Nature 420 (6915):479–81. doi:10.1038/nature01232.
- Amato, F., M. Pandolfi, M. Viana, X. Querol, A. Alastuey, and T. Moreno. 2009. Spatial and chemical patterns of PM10 in road dust deposited in urban environment. Atmos. Environ. 43 (9):1650–59. doi:10.1016/j.atmosenv.2008.12.009.
- Anderson, J. O., J. G. Thundiyil, and A. Stolbach. 2012. Clearing the air: A review of the effects of particulate matter air pollution on human health. J. Med. Toxicol. 8:166–75. doi:10.1007/s13181-011-0203-1.
- Bonifacio, H. F., R. G. Maghirang, S. L. Trabue, L. L. McConnell, J. H. Prueger, and E. R. Bonifacio. 2015. TSP, PM10, and PM2.5 emissions from a beef cattle feedlot using the flux-gradient technique. Atmos. Environ. 101:49–57. doi:10.1016/j.atmosenv.2014.11.017.
- Brook, R. D., and S. Rajagopalan. 2012. Chronic air pollution exposure and endothelial dysfunction what you can’t see-can harm you. J. Am. Coll. Cardiol. 60 (21):2167–69. doi:10.1016/j.jacc.2012.08.974.
- Cambra-Lopez, M., A. J. Aarnink, Y. Zhao, S. Calvet, and A. G. Torres. 2010. Airborne particulate matter from livestock production systems: A review of an air pollution problem. Environ. Pollut. 158:1–17. doi:10.1016/j.envpol.2009.07.011.
- Colson, A. J., L. Vredenburgh, R. E. Guevara, N. P. Rangel, C. T. Kloock, and A. Lauer. 2017. Large-scale land development, fugitive dust, and increased coccidioidomycosis incidence in the antelope valley of California, 1999-2014. Mycopathologia 182:439–58. doi:10.1007/s11046-016-0105-5.
- Dellwik, E., M. P. van der Laan, N. Angelou, J. Mann, and A. Sogachev. 2019. Obersved and modeled near-wake flow behind a solitary tree. Agr. And Forest Meteorol. 265:78–87. doi:10.1016/j.agrformet.2018.10.015.
- Doygun, N., and H. Doygun. 2018. A research on the use of vegetation barriers in traffic noise control. Ksu Tarim Ve Doga Dergisi-Ksu J. Agr. Nature 21:599–606. doi:10.18016/ksudobil.369519.
- Fogg, G. E. 1947. Quantitative studies on the wetting of leaves by water. Proc. R.Soc Med. 134:503–22. doi:10.1098/rspb.1947.0028.
- Gosselin, F. P., and E. de Langre. 2011. Drag reduction by reconfiguration of a poroelastic system. J. Fluid Struct. 27:1111–23. doi:10.1016/j.jfluidstructs.2011.05.007.
- Guo, L. 2011. Measurement and control of particulate emission from cattle feedlots in Kansas, ProQuest Dissertations Publishing.
- Guo, L., S. Ma, D. Zhao, B. Zhao, B. Xu, J. Wu, J. Tong, D. Chen, Y. Ma, M. Li, et al. 2019. Experimental investigation of vegetative environment buffers in reducing particulate matters emitted from ventilated poultry house. J. Air Waste Manag. Assoc. 69 (8):934–43. doi:10.1080/10962247.2019.1598518.
- Huang, R. J., Y. Zhang, C. Bozzetti, K. F. Ho, J. J. Cao, Y. Han, K. R. Daellenbach, J. G. Slowik, S. M. Platt, F. Canonaco, et al. 2014. High secondary aerosol contribution to particulate pollution during haze events in China. Nature 514 (7521):218–22. doi:10.1038/nature13774.
- Hwang, H. J., S. J. Yook, and K. H. Ahn. 2011. Experimental investigation of submicron and ultrafine soot particle removal by tree leaves. Atmos. Environ. 45 (38):6987–94. doi:10.1016/j.atmosenv.2011.09.019.
- Jia, Y., W. Huixia, and X. Binze. 2015. Accumulation of particulate matter on leaves of nine urban greening plant species with different micromorphological structures in Beijing. Res. Environ. Sci. 28 (3):384–92. doi:10.13198/j.1001-6929.2015.03.08.
- Koch, K., R. Samson, and S. Denys. 2019. Aerodynamic characterisation of green wall vegetation based on plant morphology: An experimental and computational fluid dynamics approach. Biosyst. Eng. 178:34–51. doi:10.1016/j.biosystemseng.2018.10.019.
- Kretinin, V. M., and Z. M. Selyanina. 2006. Dust retention by tree and shrub leaves and its accumulation in light chestnut soils under forest shelterbelts. Eurasian Soil Sci. 39 (3):334–38. doi:10.1134/s1064229306030136.
- Langre, D., and Emmanuel. 2008. Effects of wind on plants. Annu Rev Fluid Mech. 40 (1):141–68. doi:10.1146/annurev.fluid.40.111406.102135.
- Lee, E. S., D. R. Ranasinghe, F. E. Ahangar, S. Amini, S. Mara, W. Choi, S. Paulson, and Y. F. Zhu. 2018. Field evaluation of vegetation and noise barriers for mitigation of near freeway air pollution under variable wind conditions. Atmos. Environ. 175:92–99. doi:10.1016/j.atmosenv.2017.11.060.
- Li, X. B., Q. C. Lu, S. J. Lu, H. D. He, Z. R. Peng, Y. Gao, and Z. Y. Wang. 2016a. The impacts of roadside vegetation barriers on the dispersion of gaseous traffic pollution in urban street canyons. Urban For. Urban Gree. 17:80–91. doi:10.1016/j.ufug.2016.03.006.
- Li, Y., J. Krahn, and C. Menon. 2016b. Bioinspired dry adhesive materials and their application in robotics: A review. J. Bionic Eng. 13:181–99. doi:0.1016/S1672-6529(16)60293-7.
- Lin, M. Y., and A. Khlystov. 2012. Investigation of ultrafine particle deposition to vegetation branches in a wind tunnel. Aerosol Sci. Technol. 46:465–72. doi:10.1080/02786826.2011.638346.
- Lin, X. 2006. Simulation of odor dispersion around natural windbreaks. PhD diss. Ste-Anne-deBellevue, Quebec: McGill University.
- Ni, J. Q. 2015. Research and demonstration to improve air quality for the U.S. animal feeding operations in the 21st century - a critical review. Environ. Pollut. 200:105–19. doi:10.1016/j.envpol.2015.02.003.
- Nowak, D. J., S. Hirabayashi, A. Bodine, and R. Hoehn. 2013. Modeled PM2.5 removal by trees in ten U.S. cities and associated health effects. Environ. Pollut. 178:395–402. doi:10.1016/j.envpol.2013.03.050.
- Pascal, M., P. de Crouy Chanel, V. Wagner, M. Corso, C. Tillier, M. Bentayeb, M. Blanchard, A. Cochet, L. Pascal, S. Host, et al. 2016. The mortality impacts of fine particles in France. Sci. Total Environ. 571:416–25. doi:10.1016/j.scitotenv.2016.06.213.
- Qi, J., J. Wei, Z. Qian, and R. Liu. 2013. Problems and contermeasures in research on urban trees’ dust-retaining ability. World For Res. 26:52–57. In Chinese.
- Rai, A., K. Kulshreshtha, P. K. Srivastava, and C. S. Mohanty. 2009. Leaf surface structure alterations due to particulate pollution in some common plants. The Environmentalist 30 (1):18–23. doi:10.1007/s10669-009-9238-0.
- Ranasinghe, D., E. S. Lee, Y. F. Zhu, I. Frausto-Vicencio, W. Choi, W. Sun, S. Mara, U. Seibt, and S. E. Paulson. 2019. Effectiveness of vegetation and sound wall-vegetation combination barriers on pollution dispersion from freeways under early morning conditions. Sci. Total Environ. 658:1549–58. doi:10.1016/j.scitotenv.2018.12.159.
- Rasanen, J. V., T. Holopainen, J. Joutsensaari, C. Ndam, P. Pasanen, A. Rinnan, and M. Kivimaenpaa. 2013. Effects of species-specific leaf characteristics and reduced water availability on fine particle capture efficiency of trees. Environ. Pollut. 183:64–70. doi:10.1016/j.envpol.2013.05.015.
- Rogge, W. F., P. M. Medeiros, and B. R. T. Simoneit. 2006. Organic marker compounds for surface soil and fugitive dust from open lot dairies and cattle feedlots. Atmos. Environ. 40:27–49. doi:10.1016/j.atmosenv.2005.07.076.
- Saebo, A., R. Popek, B. Nawrot, H. M. Hanslin, H. Gawronska, and S. W. Gawronski. 2012. Plant species differences in particulate matter accumulation on leaf surfaces. Sci. Total Environ. 427-428:347–54. doi:10.1016/j.scitotenv.2012.03.084.
- Sgrigna, G., A. Saebo, S. Gawronski, R. Popek, and C. Calfapietra. 2015. Particulate matter deposition on Quercus ilex leaves in an industrial city of central Italy. Environ. Pollut. 197:187–94. doi:10.1016/j.envpol.2014.11.030.
- Středová, H., J. Podhrázská, T. Litschmann, T. Středa, and J. Rožnovský. 2012. Aerodynamic parameters of windbreak based on its optical porosity. Contrib. Geophys Geodesy. 42:213–26. doi:10.2478/v10126-012-0008-5.
- Suriadikusumah, A., W. Nugraha, N. Nurlaeny, and R. Devnita. 2013. Effect of different mixed media (merapi volcanic ash, cow manure and mineral soil) on chemical properties of soil and growth of maize (Zea mays L.). J. Agr. Sci. 5. doi:10.5539/jas.v5n2p188.
- Terzaghi, E., E. Wild, G. Zacchello, B. E. L. Cerabolini, K. C. Jones, and A. Di Guardo. 2013. Forest filter effect: Role of leaves in capturing/releasing air particulate matter and its associated PAHs. Atmos. Environ. 74:378–84. doi:10.1016/j.atmosenv.2013.04.013.
- Tiwary, A., H. P. Morvan, and J. J. Colls. 2006. Modelling the size-dependent collection efficiency of hedgerows for ambient aerosols. Aerosol Sci. 37 (8):990–1015. doi:10.1016/j.jaerosci.2005.07.004.
- Tong, J., X. Liu, R. Maghirang, K. Wei, L. Liu, C. Wang, Y. Ma, D. Chen, H. Yan, and L. Guo. 2017. Investigation of the potential and mechanism of clove for mitigating airborne particulate matter emission from stationary sources. J. Bionic Eng. 14 (2):390–400. doi:10.1016/s1672-6529(16)60407-9.
- Tong, Z. M., R. W. Baldauf, V. Isakov, P. Deshmukh, and K. M. Zhang. 2016. Roadside vegetation barrier designs to mitigate near-road air pollution impacts. Sci. Total Environ. 541:920–27. doi:10.1016/j.scitotenv.2015.09.067.
- Tsutsui, O., R. Sakamoto, M. Obayashi, S. Yamakawa, T. Handa, D. Nishio-Hamane, and I. Matsuda. 2016. Light and SEM observation of opal phytoliths in the mulberry leaf. Flora - Morpho, Distrib, Func. Ecol. Plants 218:44–50. doi:10.1016/j.flora.2015.11.006.
- Tyndall, J., and J. Colletti. 2006. Mitigating swine odor with strategically designed shelterbelt systems: A review. Agroforestry Syst. 69 (1):45–65. doi:10.1007/s10457-006-9017-6.
- Uzoma, K. C., M. Inoue, H. Andry, H. Fujimaki, A. Zahoor, and E. Nishihara. 2011. Effect of cow manure biochar on maize productivity under sandy soil condition. Soil Use Manage 27 (2):205–12. doi:10.1111/j.1475-2743.2011.00340.x.
- Viippola, V., T. H. Whitlow, W. Zhao, V. Yli-Pelkonen, J. Mikola, R. Pouyat, and H. Setälä. 2018. The effects of trees on air pollutant levels in peri-urban near-road environments. Urban For. Urban Gree. 30:62–71. doi:10.1016/j.ufug.2018.01.014.
- Weber, F., I. Kowarik, and I. Saumel. 2014. Herbaceous plants as filters: Immobilization of particulates along urban street corridors. Environ. Pollut. 186:234–40. doi:10.1016/j.envpol.2013.12.011.
- Wei, J., X. Liu, X. Zhang, C. Wang, X. Chen, X. Liang, Y. Hou, and H. Li. 2015. Influences of hydrosoluble and lipophilic rhizodeposits on pyrene sorption in soil. CLEAN - Soil, Air, Water 43:1401–08. doi:10.1002/clen.201400751.
- Wong, C. M., N. Vichit-Vadakan, H. Kan, and Z. Qian. 2008. Public health and air pollution in Asia (PAPA)_ A multicity study of short-term effects of air pollution on mortality. Environ. Health Persp. doi:10.1289/ehp.11257.
- Wu, Y., W. Ma, J. Liu, L. Zhu, L. Cong, J. Zhai, Y. Wang, and Z. Zhang. 2018. Sabina chinensis and Liriodendron chinense improve air quality in Beijing, China. PLoS One 13 (1):e0189640. doi:10.1371/journal.pone.0189640.
- Wuyts, K., J. Hofman, S. Van Wittenberghe, G. Nuyts, K. De Wael, and R. Samson. 2018. A new opportunity for biomagnetic monitoring of particulate pollution in an urban environment using tree branches. Atmos. Environ. 190:177–87. doi:10.1016/j.atmosenv.2018.07.014.
- Xie, C., L. Kan, J. Guo, S. Jin, Z. Li, D. Chen, X. Li, and S. Che. 2018. A dynamic processes study of PM retention by trees under different wind conditions. Environ. Pollut. 233:315–22. doi:10.1016/j.envpol.2017.10.073.
- Yan, H., G. Ding, H. Li, Y. Wang, L. Zhang, Q. Shen, and K. Feng. 2019. Field evaluation of the dust impacts from construction sites on surrounding areas: A city case study in China. Sustainability 11. doi:10.3390/su11071906.
- Zhan, Q. W., and C. X. Qian. 2018. Mineralization and cementation of fugitive dust based on the utilization of carbon dioxide and its characterization. J. Wuhan Univ. Technol. 33 (2):263–67. doi:10.1007/s11595-018-1815-x.
- Zhang, Y. L., and F. Cao. 2015. Fine particulate matter (PM 2.5) in China at a city level. Sci Rep. 5:14884. doi:10.1038/srep14884.
- Zhao, G., Y. Chen, P. K. Hopke, T. M. Holsen, and S. Dhaniyala. 2017. Characteristics of traffic-induced fugitive dust from unpaved roads. Aerosol Sci. Technol. 51:1324–31. doi:10.1080/02786826.2017.1347251.