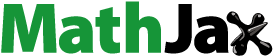
ABSTRACT
The COVID-19 pandemic has created an urgent need to utilize existing and develop new intervention technologies for SARS-CoV-2 inactivation on surfaces and in the air. Ultraviolet (UV) technology has been shown to be an effective antimicrobial intervention. Here a study was conducted to determine the efficacy of commercially available UV and blue light-based devices for inactivating HCoV-229E, a surrogate of SARS-CoV-2. The results indicate that two UV devices designed for surface disinfection, with doses of 8.07 µJ/cm2 for the 254 nm device and 20.61 µJ/cm2 for the 275 nm device, were efficient in inactivating 4.94 logs of surface inoculated HCoV-229E. Additionally, a 222 nm UV device with intended ceiling-based operation was effective in inactivating 1.7 logs of the virus inoculated on surface, with a dose of 6 mJ/cm2. A ceiling-based device designed to emit blue light at 405 nm was found to produce 89% reduction in HCoV-229E inoculated on a surface for a dose of 78 J/cm2. Finally, the UV based 222 nm device was found to produce a 90% reduction in the concentration of airborne HCoV-229E, at a 55 µJ/cm2 dose. These results are indicative of the great potential of using UV based technology for the control of SARS-CoV-2.
Implications: An important avenue of arresting COVID-19 and future pandemics caused by infectious pathogens is through environmental disinfection. To this effect, the study presented here evaluates commercially available UV and blue light based antimicrobial devices for their ability to kill the human coronavirus HCoV-229E, a surrogate of SARS-CoV-2, on surfaces and in air. The results indicate that two handheld UV devices produced complete inactivation of surface viral inoculum and a UVC ceiling based device produced 1 log reduction in HCoV-229E in air. These results imply the efficacy of UV technology as an antimicrobial tool, especially for rapid disinfection of indoor air.
Introduction
The recent coronavirus disease-19 (COVID-19) pandemic, caused by the sudden acute respiratory syndrome-coronavirus-2 (SARS-CoV-2) has highlighted the problem of rapid global spread of viral diseases. According to the CDC’s COVID tracker, there have been more than 80 million people infected with SARS-CoV-2 in the United States, with more than one million deaths (CDC Citation2022). Susceptible people such as the elderly and even children can be infected and develop serious diseases (Moschovis et al. Citation2021). The development of multiple vaccines has helped ease the disease burden on the U.S. economy and health care system, with restrictions being eased and case numbers reducing. However, the emergence of various mutant strains of SARS-CoV-2 and the lower-than-expected vaccination numbers due to vaccine hesitancy, have led to the recurrence of disease outbreaks. The current limitations of the vaccination approach underscore the need to approach the problem from another angle, by minimizing virus transmission using effective environmental intervention approaches including filtration and use of other emerging antimicrobial platforms (Huang et al. Citation2019, Citation2021; Mahase Citation2021; Pyrgiotakis et al. Citation2016; Vaze and Demokritou Citation2022; Vaze et al. Citation2018, Citation2019b, Citation2022).
To this effect, Ultraviolet (UV) light technology for disinfection purposes, also known as GUV (germicidal UV) has been utilized for sterilizing medical equipment, indoor microenvironments, as well as in the food and the water industry to inactivate microorganisms. UV acts primarily by producing DNA damage that the cell cannot overcome (Darnell et al. Citation2004; Stein et al. Citation1989). UV can also induce intracellular Reactive Oxygen Species (Reshi, Su, and Hong Citation2014), which have deleterious effects on the cell. The several types of UV utilized are Mercury-based lamps, light-emitting diodes (UV-C LED) and pulsed-xenon lamps that emit UV light across the entire UV spectrum with a peak emission near 230 nm (Mackenzie Citation2020). These UV systems have been shown to be effective against pathogens both on surfaces and in air (Reed Citation2010).
There has been a renewed focus on the applicability of the UV technology for inactivating coronaviruses, and specifically the SARS-CoV-2. An earlier study detailed the use of 254 nm UV source for the inactivation of the SARS-CoV-1 virus in culture with complete inactivation after 7 minutes of exposure (Darnell et al. Citation2004). Since the beginning of the pandemic, there have been a few studies regarding SARS-CoV-2 susceptibility to UV. In one study, pulsed xenon UV technology was shown to be effective in inactivating the SARS-CoV-2 virus inoculated on N95 masks by greater than 4 logs, after 5 minutes of exposure (Simmons et al. Citation2021). Another study reported 6 log reduction in SARS-CoV-2 in suspension by UVC with a 1 J/cm2 dose (Heilingloh et al. Citation2020). UV-C has been utilized by a few groups for efficacy testing against SARS-CoV-2 (Biasin et al. Citation2021; Storm et al. Citation2020). For UV-LED, a 3 log reduction was reported at 280 nm wavelength after 3mJ/cm2 dose (Minamikawa et al. Citation2021).
However, a 2021 review shows that most of the studies for determining the efficacy of UV and light emitting systems against coronaviruses utilize the virus in suspension and do not consider real world modalities of transmission, especially aerosol transmission (Chiappa et al. Citation2021). One of the few studies of such nature that have been conducted, indicates a strong effect of UV, especially 222 nm UVC against coronaviruses (Buonanno et al. Citation2020). Apart from the UV, short wavelength visible light with a spectrum from 380 to 500 nm that includes violet, indigo, blue, and some blue-green light systems, have also been used for disinfection purposes. More specifically, short wavelength visible light at 405 nm has been shown to inactivate pathogens such as Pseudomonas aeruginosa and Staphylococcus aureus, achieving as much as 95.1% and nearly 90% inactivation, respectively, with the microbes inoculated onto agar (Guffey and Wilborn Citation2006). An LED light array producing 405 nm was used to inactivate E. coli in solution, resulting in 0.4 log reduction by a dose of 117 J/cm2 (McKenzie et al. Citation2016). However, there is a sparsity of research on the application of blue light technology for specifically antiviral purposes.
To this effect, the present study was carried out, to assess the efficacy of four commercially available UV and blue light-based devices, for inactivating a surrogate of SARS-CoV-2 coronavirus on surfaces and in air. The specifications of the devices are detailed in the methods section. As the first tier of evaluation of device efficacy, all four devices were tested and their ability to inactivate SARS-CoV-2 surrogate inoculated on a surface was assessed. Having thus established the inactivation potential of the devices, as the second step, the ceiling mounted devices that were efficient in inactivating the virus on surfaces were assessed in terms of air disinfection.
The surrogate of SARS-CoV-2 used in this study was the Human Coronavirus HCoV-229E (Malik Citation2020). Although an alphacoronavirus and not a betacoronavirus like SARS-CoV-2, HCoV-229E is one of the other coronaviruses known to cause disease in humans, and has been one of the most extensively utilized as a surrogate for study of disinfection (Carraturo et al. Citation2020; Cimolai Citation2020; Meyers et al. Citation2021). The virus was challenged with UV and light based technologies on surfaces and in air, with the results being interpreted as validating the applicability of the tested devices.
Materials and methods
Description of devices tested
The description of devices tested in this study is summarized in .
Table 1. Details of devices tested in this study.
Device A
This is a rectangular device, handheld device intended for surface sterilization. The device contains a linear UV source of 53.5 cm length, with the low pressure mercury lamp producing UV-C light with a peak at 254 nm. The device has a wattage rating of 55W. The device has a handle with which it can be maneuvered over a surface.
Device B
This is also a handheld device. At the center of the device, there are 12 LED sources, placed in a linear fashion. The device has a wattage rating of 30W, producing UV-C light with a peak at 275 nm. A handle is utilized to guide the device over surfaces.
Device C
This is a disc shaped device with 7.2 inches diameter, with a 2 inch by 2-inch krypton chloride (KrCl) excimer lamp. The device is rated 15W with a 12-volt DC input. The UV unit produces UVC with a peak at 222 nm wavelength. This is a ceiling-mounted device, intended to be installed at a height of 8–11 feet. The major application of this device is for air disinfection,
Device D
This is a ceiling-mounted LED blue light-based device. It has a 6-inch diameter light, producing light in dual modality (Blue/White). Here, in this study, the blue light, produced at a peak wavelength of 405 nm was used. The power consumption of the device is 38W.
Viral strain
Human coronavirus HCoV-229E was used in the inactivation experiments. HCoV-229E is a member of the genus Alphacoronavirus and is one of the globally prevalent coronaviruses, a group that includes SARS-CoV-2, which is a betacoronavirus. HCoV-229E was acquired from ATCC (Strain no. VR-740, American type culture collection, Manassas VA). Viral aliquots of 107 pfu/mL were made and stored at −80°C. A HCoV-229E viral plaque assay was developed for the quantification of the virus in experimental samples.
HCoV-229E plaque assay
The Huh 7.5 cell line (provided courtesy of B. Lindenbach, Yale School of Medicine, New Haven, CT) was used in the viral quantification plaque assay (Vecchi, Montosi, and Pietrangelo Citation2010). The Huh 7.5 cells were plated in 12-well plates and incubated overnight at 37°C (5% CO2). The following day, i.e., the day of the experiment, after aspirating the media from each well, 100 µL of virus treatment and control samples were added to each well. The plates were incubated for 1 h at 37°C 5% CO2 with gentle rocking every 10 minutes. Following incubation, a mixture of 2 X DMEM (Dulbecco’s Modified Eagle Medium) and 2.4% Avicel (microcrystalline cellulose powder) was overlain on the cells to avoid any air bubbles. The cells were then incubated at 33°C (5% CO2) for 4 days. For visualizing plaques after the incubation, cells were fixed with 5% formaldehyde for 1 hour at room temperature. After removing the formaldehyde, the cells were stained with 2% crystal violet solution. The plaques were counted, and the pfu/mL was calculated using the following formula: Plaque count X virus dilution factor X 10 = pfu/mL sample.
Experimental setup for inactivation of HCoV-229E on surface
shows the experimental setup used in the study. The entire experiment was conducted inside a class II A2 biosafety cabinet. The device being evaluated was held above the surface of the cabinet floor, at a predetermined height. For the two handheld devices, this was one inch, which is the expected distance at which these devices would be operated in their intended operation. For the ceiling-based devices, ideally the devices would be installed at a height of 8–11 feet. However, due to the necessity of these experiments needing to be conducted inside a biosafety cabinet, a height of eight inches was chosen as the height at which device could feasibly be held above the surface of the cabinet floor.
Figure 1. Schematic of the surface inactivation efficacy testing of chosen UV/light-based intervention technologies against an inoculated SARS-CoV-2 surrogate.
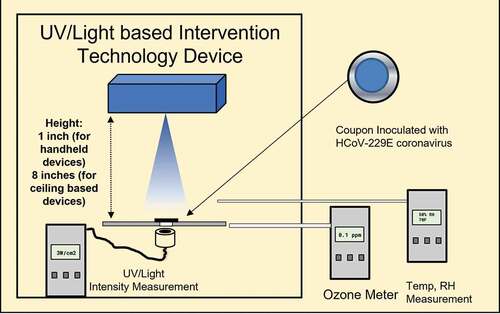
As shown in , each individual device was held above the virus-inoculated surface (circular stainless-steel coupon, 1.82 cm diameter). The device was turned on and the appropriate UV/light meter was placed directly underneath at the location of the exposure coupons. For all four devices, the measurement of intensity was performed at the peak wavelength of the device. For UV measurement, a X1–5 optometer (Gigahertz-Optik, Amesbury MA) was utilized. For the measurement of blue light, the CSS-45 remote spectral detector (Gigahertz-Optik, Amesbury MA) was used. The UV/blue light irradiance measurement was conducted in triplicate and averaged. The measurement devices utilized were calibrated by the manufacturer against the appropriate standards and verified before operation.
A treatment coupon was then placed at the treatment spot, and treatment was conducted for the specific timepoint. The treatment timepoints were chosen according to the intended application of the devices being assessed. For the two handheld devices, device A and device B, short timepoints of 1,3 and 10 seconds were selected. For the ceiling-based device C, intermediate timepoints were chosen, 30 seconds, 1 minute and 5 minutes. For the blue light-based device D, the longer time points chosen were 1,5 and 60 minutes.
Surface inoculation and recovery of HCoV-229E
10 µL of the 229E of the concentration 107 pfu/mL, was added to the center of a stainless-steel coupon (1.82 cm diameter) as the inoculum (105 pfu on each coupon) and spread gently across the face of the coupon, as shown in . The coupons were allowed to dry and then utilized for either UV treatment or held as control untreated samples. Post treatment, each coupon was added to a petri dish. 100 μL DMEM was gently pipetted onto the surface of the coupon. Once the layer of DMEM covered the entire coupon surface, gentle pipetting action was performed to remove the solution from the coupon, thus recovering the viral inoculum.
Experimental setup for inactivation of HCoV-229E in air
A “single-pass” experimental chamber was utilized to determine the efficacy of UV and blue light-based technologies on aerosolized virus; this apparatus has been used in earlier inactivation studies of various airborne microorganisms (Ko, First, and Burge Citation2000; McDevitt et al. Citation2007; McDevitt, Rudnick, and Radonovich Citation2012). The body of the chamber is constructed with stainless steel and consists of three parts: 1) the head (165×165×343 mm), 2) the main body (63×305×381 mm), and 3) the tail (42×305×25 mm). The head part of the chamber consisted of ports for the injection of the viral bioaerosol and supplemental HEPA-filtered air (see below for details). In the main body section of the chamber, there is a fused quartz UV exposure window (279×254 mm). The device to be evaluated was installed directly above this window at a height of 8 inches from the upper surface of the quartz window. Sampling of the UV-exposed bioaerosol and untreated control bioaerosol was performed through a sampling assembly connected at the end of the tail part. A schematic of the experimental setup is shown in . The entire experimental setup including the chamber and sampling apparatus was placed inside a biosafety cabinet.
Figure 2. Schematic of the air disinfection efficacy testing of UV based device C. The “single-pass” experimental chamber is shown, along with experimental airflows.
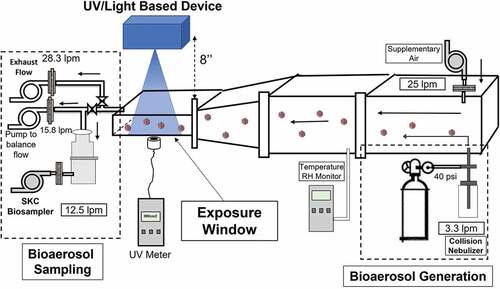
UV intensity measurement was performed by placing the UV sensor at a single 29 mm diameter circular section on the bottom of the exposure window directly underneath the UV device prior to sampling. Three measurements were recorded and averaged. The total UV exposure dose (mJ/cm2) for bioaerosol inactivation was calculated as UV intensity (mW/cm2) multiplied by time of treatment (seconds).
Generation of HCoV-229E bioaerosols
Briefly, a single-jet Collison nebulizer (CH Technologies, NJ) containing 1 × 107 pfu/mL HCoV-229E in PBSA (Phosphate Buffered Saline +0.1% Bovine Serum Albumin) was operated at 40 psig (pound-force per square inch) input pressure. The output of the nebulizer, producing viral bioaerosols at 3.3 lpm (liters per minute) was connected to an input port at the bottom of the head section of the chamber. Supplemental HEPA-filtered air (25 lpm) was injected through the second input port to ensure mixing of the bioaerosol with air in the chamber. The total input airflow into the chamber was 28.3 lpm which is equivalent to 1 cfm (1 cubic feet per minute). The UV exposure time, which was the residence time of the bioaerosol in the exposure window, was calculated to be 7.6 seconds.
Bioaerosol sampling
An SKC Biosampler (SKC Inc., Eighty Four, PA) was utilized for sampling of the UV exposed bioaerosol and the untreated bioaerosol (baseline). The biosampler had an operational flow rate of 12.5 lpm. To balance the 28.3 lpm flow rate of the chamber, a bypass pump operating at 15.8 lpm was connected to the sampling assembly. 20 mL of a viral sampling fluid was added to the biosampler. Sampling was performed for 20 min for each sample (baseline and UV-treated). Triplicate samples were first collected for the control baseline, followed by the UV-treated samples in triplicate.
Environmental parameters
The temperature and relative humidity were measured during experiments with a HOBO data logger (Grainger, Lake Forest IL). Ozone levels were measured with an Aeroqual series 200 ozone monitor (Gas Sensing, Inwood IA).
Statistical analysis
The inactivation experiments were performed in triplicates and the log reduction values at each timepoint were calculated, as detailed in earlier publications from this group (Huang et al. Citation2021; Vaze et al. Citation2019, Citation2019a). In summary, accounting for the natural decay of the microorganisms at time t, the log reduction was calculated according to the following equation where, CExp (0) is the microorganism concentration of the treatment coupon at time “0” while CExp (t) is the concentration of the exposed microorganisms at time “t”. The log reduction (LR) is defined as:
The aerosol inactivation results were used to determine the Z-value of the UV exposure. By definition, the Z-value is equivalent to the slope for the relationship between UV dose and logarithm of % survival (Walker and Ko Citation2007). The higher the Z-value, the more susceptible the organism. This is calculated as:
Where, No= baseline viral concentration averaged across the triplicate sampling runs, Nuv = UV-exposed viral concentration averaged across the triplicate sampling runs and D = effective UV dose (mJ/cm2), i.e., Intensity measured (mW/cm2) X time of exposure (seconds). For the aerosol inactivation results, a logarithmic fit of the survival fraction (NUV/N0) of the virus was plotted against the UV exposure dose.
Results and discussion
shows the summary of results of the tier one inactivation testing, performed on stainless steel surfaces inoculated with the virus. For device A (254 nm), the UV intensity measured at the treatment surface was 8.07 mW/cm2. The corresponding doses for the different treatment timepoints were calculated to be 8.07, 24.2 and 80.67 mJ/cm2 (for 1, 3 and 10 seconds respectively). The temperature measured near the surface of treatment was approximately 71° F. Relative humidity was 43.4%. There was no significant difference between these values and environmental measurements, taken away from the UV device. Ozone levels were below the limit of detection of 0.01 ppm. The control (baseline) samples did not show significant reduction in the viral concentration for the entire timecourse of the treatment. For control samples held for 1, 3 and 10 seconds, the concentration of the virus recovered was 8.83 × 104 (±4.71 × 103) pfu/mL, 8.83 × 104 (±1.43 × 104) pfu/mL, and 6 × 104 (±1.08 × 104) pfu/mL, respectively. The insignificant reduction in the control concentration of the virus can be attributed to the fact that HCoV-229E has been shown to be extremely resilient in its survivability on various surface materials (Bonny, Yezli, and Lednicky Citation2018). In the case of the UV treatment, complete inactivation was observed for all three time points, as shown in . No viable virus was recovered from the treatment coupons. This would translate to 4.94, 4.94 and 4.77 log reduction at UV doses of 8.07, 24.2 and 80.67 mJ/cm2 (for 1, 3 and 10 seconds of UV exposure respectively). Thus, it can be inferred that only momentary contact with the UV produced by this device (254 nm) can be effective in inactivating HCoV-229E on stainless steel surfaces.
Figure 3. Summary of the surface inactivation efficacy testing of UV/light based devices. The control (●) and treatment (■) concentration values (PFU/mL) of HCoV-229E are shown as a function of exposure time. The values are averages of triplicate runs and error bars are one standard deviation.
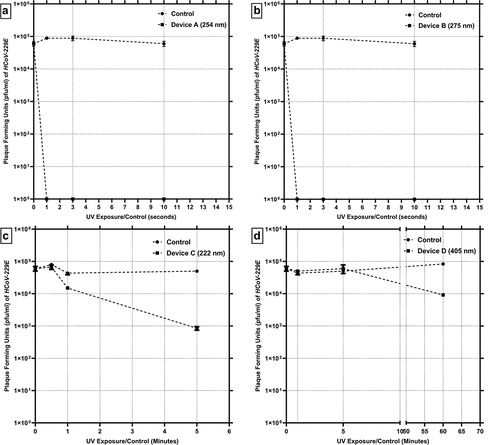
These HCoV-229E inactivation results are in line with published data on UV 254 nm with other microorganisms. As it is well known, the 254 nm light damages the viral deoxyribonucleic acid (DNA) or ribonucleic acid (RNA) so that the virus cannot reproduce (Beck et al. Citation2016a, Citation2016b; Mackenzie Citation2020). The susceptibility of HCoV-229E to UV radiation, especially 254 nm, has been well studied and one study reported a dose of 1.8 mJ/cm2 leading to one log reduction (Boegel et al. Citation2021). As the doses delivered in the present study are magnitude higher, the much higher inactivation observed is coherent. For SARS-CoV-2 itself, there are few studies assessing its susceptibility with 254 nm handheld devices. A recent study reported a 6 log inactivation of SARS-CoV-2 in liquid suspension exposed with a mercury UVC lamp (254 nm) at 5 cm (2 inches) distance with a 1048 mJ/cm2 dose (Heilingloh et al. Citation2020). Another study of note utilized handheld 254-nm UV devices evaluated against SARS-CoV-2 at a 5 cm (2 inches) distance. Results in that study indicate a complete inactivation (6 log reduction) after a UV dose of 800 mJ/cm2 (Ruetalo, Businger, and Schindler Citation2020). The highly efficient results presented in the current study further indicate that the device A will be an effective handheld surface sterilization device against SARS-CoV-2.
For the handheld device B (275 nm), the UV intensity measured at the treatment surface was 20.61 mW/cm2. The corresponding dose for each treatment timepoint was calculated to be 20.61, 61.83 and 206.1 mJ/cm2 (for 1, 3 and 10 seconds respectively). The temperature measured near the surface of treatment was 70.1° F and the relative humidity was 43.2%. Here, it is to be noted that, although there was no significant increase in the temperature in the surrounding area, the device itself became extremely hot to the touch. Ozone levels were below the limit of detection of 0.01 ppm.
summarizes the inactivation as a function of exposure time for device B. Here, similar values were observed for control, as the earlier handheld device with 8.83 × 104 (±4.71 × 103) pfu/mL for 1 second, 8.83 × 104 (±1.43 × 104) pfu/mL at 3 seconds, and 6 × 104 (±1.08 × 104) pfu/mL at 10 seconds. For the UV exposed surface, a complete inactivation was observed for all three time points. No viable virus was recovered from the treatment coupons. This would translate to 4.94, 4.94 and 4.77 log reduction at for doses of 20.61, 61.83 and 206.1 mJ/cm2 (for 1, 3 and 10 seconds respectively). The inactivation results are identical to the 254 nm device A. However, the dose values reported here for the 275 nm device B are three (3) times greater due to its higher intensity than the 254 nm device.
LED UVC disinfection is an emerging field in the application of UV for disinfection purposes, and there are few studies of the inactivation of coronaviruses at LED wavelengths. There has however, been renewed interest due to the current pandemic. A recent study discovered that across the UVC LED wavelength spectrum, 267 nm, which is similar to the 275 nm operation of this device, was the most effective wavelength in inactivating another surrogate of SARS-CoV-2, the HCoV-OC43 (Gerchman et al. Citation2020). In another study, a UV LED instrument was used to treat a clinical isolate of SARS-CoV-2 at a distance of 2 cm, which is similar to the distance utilized in the current study. Here a dose of 37.5 mJ/cm2 led to a 99.9% (3 log) inactivation (Inagaki et al. Citation2020). Thus, from the results obtained against HCoV-229E in this study, it can be inferred that the 275-nm device B is also likely well-suited to inactivate coronaviruses in relatively short contact times, validating its handheld operation.
The device C is intended for a ceiling-based operation. As this device is a 222 nm UVC device, it is considered to be safer for human exposure, as compared to 254 nm (Buonanno et al. Citation2017; Freeman et al. Citation2022). Here, the UV intensity was significantly lower than the other two UV based devices evaluated in this study. The UV intensity measured at the treatment surface was 0.02 mW/cm2. During the treatment, the temperature gradually increased near the treatment zone compared to surrounding one, with the initial temperature being 75.2° F and the final temperature measured at the end of the 5-minute treatment elevated to 80.2° F. Relative humidity remained constant and same as initial pretreatment levels, at 45.4%. Ozone levels were below the limit of detection of 0.01 ppm.
summarizes the surface inactivation results as a function of exposure time. Here, a gradual time course of inactivation was observed for the timepoints evaluated. The three timepoints utilized for treatment were 30 seconds, 1 minute and 5 minutes. The calculated UV dose for the three treatment timepoints was 0.6, 1.2 and 6 mJ/cm2, respectively. For the untreated controls, the concentrations of virus recovered were 8 × 104 (±4.08 × 103) pfu/mL, 4.33 × 104 (±4.71 × 103) pfu/mL and 5 × 104 (±4.08 × 103) pfu/mL, respectively. For the UV treatment, 6.66 × 104 (±1.01 × 104) pfu/mL were recovered after 30 seconds and 1.5 × 104 (±1.08 × 103) pfu/mL after 1 minute. The highest level of inactivation was observed for 5 minutes of treatment with 8.5 × 102 (±1.08 × 102) pfu/mL recovered. This constitutes a 1.77 log reduction (6 mJ/cm2 UV dose). Linear Curve fitting analysis for the inactivation timecourse led to a log reduction rate of 0.34 logs/minute (R2 = 0.738).
Far-UVC light (207–222 nm) is very strongly absorbed by proteins through peptide bonds, as well as by other biomolecules, leading to intracellular damage. However, the ability of far-UVC to penetrate biological materials is limited compared with conventional germicidal UV light (254 nm or greater), which can reach and damage internal nucleic acids (Coohill Citation1986; Goldfarb and Saidel Citation1951). Fewer studies of the efficacy of 222 nm UV against coronaviruses exist, as opposed to the more prevalent 254 nm. But recent studies have indeed pointed toward the applicability of 222 nm UV for inactivating coronaviruses. One study reported an inactivation of 99.95% (3.3 log reduction) after a 19.42 mJ/cm2 dose (Jones and Norton Citation2021). Another study utilized a 222 nm source placed 24 cm above a coupon inoculated with SARS-CoV-2, with a 2.35 log reduction at a dose of 3 mJ/cm2 (Kitagawa et al. Citation2021). The results from current study are congruous with these data.
For blue light-based device D, the light intensity measured at the treatment surface was 21.67 mW/cm2. When the device was operated for the longest timepoint of treatment, i.e., 1 hour, the temperature of the treatment zone increased from 75.1°F to 82.3°F. The relative humidity also decreased from 40% to 28.2%. Ozone levels were below the limit of detection of 0.01 ppm.
summarizes the inactivation produced by a light based device D as a function of exposure time. Here, no significant inactivation for the shorter timepoints tested was observed, namely 1 and 5 minutes. At 1- and 5-minute treatment, the controls at these timepoints were 4.33 × 104 (±4.71 × 103) pfu/mL for 1-minute and 5 × 104 (±4.08 × 103) pfu/mL for the 5 minutes. For the UV exposed samples, 5 × 104 (±4.08 × 103) pfu/mL were recovered for 1 minute of treatment and 6 × 104 (±1.78 × 103) pfu/mL for the 5-minute treatment. Hence, the treatment time was increased significantly, to 60 minutes. For this 60-minute treatment, the control samples contained 8.33 × 104 (±6.23 × 103) pfu/mL. The light treated samples contained 9.166 × 103 (±6.23 × 102) pfu/mL. This indicates an 89% (0.958 logs) reduction. The calculated light dose for the 1-, 5- and 60-minute timepoints was 1.3, 6.5 and 78 J/cm2. This product was advertised to disinfect over an extended period of exposure which resulted in the extended testing durations chosen here.
There are only a few studies in the literature regarding the use of blue light technology against viruses, especially coronaviruses. A recent study analyzed various wavelengths for their efficacy against HCoV-229E and it was observed that for 405 nm pulsed blue light, there was 44% reduction after a 130 J/cm2 dose (Enwemeka, Bumah, and Mokili Citation2021). Blue light was also recently evaluated in the context of SARS-CoV-2, with the results indicating that at the lowest tested irradiation dose of 0.035 mW/cm2, a reduction of 55.08% was seen after 4 hours of treatment, and the reduction after 24 hours was 90.17% (Rathnasinghe et al. Citation2021). Comparatively, device D has shown higher inactivation potential in this study. However, it should be noted that the increase in the temperature and substantial reduction in the relative humidity may have also contributed to the inactivation observed here. Given the notable enhancement in viral reduction observed here as a function of time compared to previous work, future investigation of the influence of temperature and humidity on this type of treatment would be valuable. Further, since a 1-h long exposure was required to produce 1 log reduction, it was surmised that studying inactivation of airborne HCoV-229E for this device with the current single-pass aerosol chamber setup, which has an exposure residence time of 7.6 seconds, would be infeasible as the delivered dose would be too small to lead to meaningful inactivation. For device D, a larger, room-sized chamber with longer residence and contact time would be more appropriate for evaluating the potential antiviral effect.
As the second tier of evaluation, we investigated the efficacy of device C in inactivating airborne HCoV-229E. As detailed in the methods section, the aerosolized virus was exposed to UV light at 222 nm from device C and inactivation was determined. denotes survival fraction of the HCoV-229E plotted against the UV dose. Here, the concentration of the virus sampled in the biosampling fluid during control runs (no UV exposure) was 9 × 103 (±2.58 × 103) pfu/mL. After 222 nm UV exposure, the concentration of the surviving virus was 8.17 × 102 (±1.34 × 102) pfu/mL, representing a 90.9% reduction (~1 log) in the concentration of aerosolized HCoV-229E. The intensity of UV that the airborne virus was exposed to was measured to be 7.32 µW/cm2. The effective UV dose delivered to the airborne viruses was calculated by multiplying this intensity with the residence time of the bioaerosol in the exposure zone of the chamber, which was 7.6 seconds. This came out to 55.63 µJ/cm2. During the experiment, the relative humidity and temperature inside the chamber were monitored. During control runs, this was 50% RH and 70°F and during the UV exposure runs, 54.33% RH and 70°F. Ozone levels were below the limit of detection of 0.01 ppm. The z value, as described in the methods section, was calculated, and found to be 0.043 cm2/µJ (4.31 cm2/µJ).
Figure 4. Inactivation of aerosolized HCoV-229E by device C as a function of UV exposure dose (uJ/cm2). the values are averages of triplicate runs and error bars represent one standard deviation. A logarithmic fit was performed assuming a survival fraction of 1 at the UV exposure dose of 0 uJ/cm2.
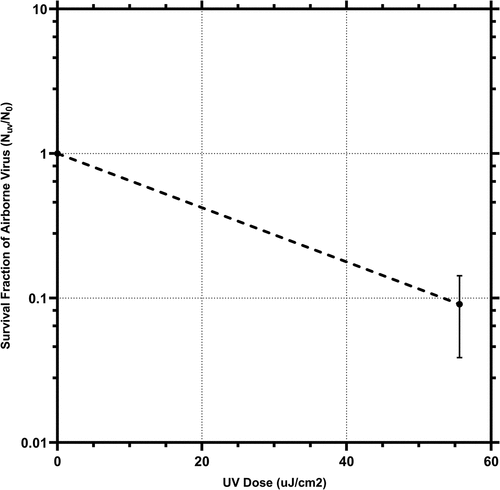
Only a few studies exist regarding the use of the 222 nm UV technology against airborne coronaviruses. In a recent study, a 222 nm UV device was utilized for inactivating airborne HCoV-229E and HCoV-OC43 with a chamber similar to the one utilized in present study. They report a k-value, which is calculated with the same formula as the z-value, of 0.41 mJ/cm2. This is significantly lower than the 4.31 mJ/cm2 value reported in this study (Buonanno et al. Citation2020). It is worth noting though that the exposure time utilized by them was significantly higher (approximately 20 seconds Vs 7.6 seconds) which makes it difficult to compare z values across studies given that the devices were different as well.
Furthermore, in another study by our group utilizing the same experimental setup and protocol using vaccinia virus, a surrogate of influenza virus, the reported Z-value was 2.54 mJ/cm2 for a 254 nm UV device, which is lower than the Z-value for the case of the coronavirus inactivation from device C. The higher Z-value reported here is indicative of the higher potential to inactivate coronavirus compared to influenza virus (McDevitt et al. Citation2007).
It is worth noting that the device C is to be installed in the ceiling of a room. Therefore, it was important to evaluate the inactivation of airborne coronavirus that this device will produce under real-world settings and dose. The region where there is the greatest chance of viral transmission in a room is where the human occupants of the room are breathing, coughing, sneezing etc., commonly referred to as the breathing zone. This zone is considered to be 6 feet from the floor per ASHRAE standard (Review of ANSI/ASHRAE Standard 62.1-Citation2004: Part 2 - Workplace Hygiene Citation2022). For a 10 ft ceiling height and a breathing zone of 6 ft above floor, we measured the UVC 222 nm intensity from Device C at the breathing zone and found it to be 0.771 µW/cm2 (below the occupational safety regulatory exposure limit of ~0.8 µW/cm2) (Buonanno et al. Citation2020). At this intensity and assuming the logarithmic fit of survival fraction as a function of exposure dose (; Z-value = 0.0431 cm2/µJ), we calculated that it would take Device C approximately 1.2 min to inactivate 90% of airborne coronavirus in the breathing zone, ~1.5 min for 95%, ~2.3 min for 99%, and ~3.5 min for 99.9% reduction, assuming no mixing of room air and absence of other sinks for the virus such as ventilation and air filtration units.
In conclusion, the results presented here affirm the efficacy of the 254 nm and 275 nm handheld devices in terms of their intended ability to inactivate viruses on surfaces, as well as demonstrating the efficacy of a ceiling based 22 nm UVC device for the inactivation of airborne viruses. These results indicate the promise of UV based technologies for the inactivation of viruses and should be considered as additional intervention tools for reducing the risk of infectious disease transmission. Thus, such studies are important not only for this, but future pandemic crises caused by viral disease transmission.
Acknowledgment
This work was made possible by a research grant from the Electric Power Research Institute (EPRI).
Disclosure statement
No potential conflict of interest was reported by the authors.
Data availability statement
The authors confirm that the data supporting the findings of this study are available within the article.
Additional information
Notes on contributors
Dilpreet Singh
Dilpreet Singh is senior health scientist at Exponent. He was previously a postdoctoral fellow at the Center for Nanotechnology and Nanotoxicology at Harvard University. His research focus is on understanding the environmental health and safety implications of industrially relevant nano-enabled products such as thermoplastics and coatings at their end-of-life, especially when they are incinerated in commercial waste incineration facilities or undergo thermal degradation during fire-related incidents in buildings. The ultimate objective of his nano-safety research is to inspire safer-by-design nano-enabled products that would minimize the nano-hazard and promote sustainable development of the nanotechnology industry.
Anand R. Soorneedi
Anand R. Soorneedi is a fifth year graduate student in the Food Science department at the University of Massachusetts, Amherst. Anand's research interests are in host-pathogen interactions, host factors that promote viral infection, developing novel concentration methods for difficult to cultivate viruses and disinfection strategies targeting emerging pathogens. He is currently developing a novel capture and concentration method for human norovirus using engineered E. coli. He is also currently assessing the potential of C. elegans as an animal model for human norovirus replication.
Nachiket Vaze
Nachiket Vaze is a research associate at the Environmental and Occupational Health Sciences Institute (EOHSI) of Rutgers University. Previously he was a postdoctoral fellow at the Center for Nanotechnology and Nanotoxicology at Harvard University. He received his Ph.D. in Biomedical Engineering from Drexel University. His research interests are nanotechnology based devices for antimicrobial applications, nano enabled materials for food safety and quality, and novel technologies for room air disinfection.
Ron Domitrovic
Ron Domitrovic is a program manager at the Electric Power Research Institute (EPRI).
Frank Sharp
Frank Sharp is a Principal Technical Leader at the Electric Power Research Institute (EPRI), an independent, non-profit research organization in the energy industry. Mr. Sharp has been a researcher at EPRI for over 12 years where he manages or co-manages projects relating to interior and exterior lighting, indoor agriculture, connected end use devices, and data centers. Frank’s research supports EPRI’s efforts related to energy efficiency, demand response, beneficial electrification, decarbonization, and emerging technologies. Recent topics of his research have included disinfection lighting, connected street lighting / smart city lighting, indoor food production, commercial robotics, and connected technologies.
Douglas Lindsey
Douglas Lindsey is a Sr. Project Manager at the Electric Power Research Institute (EPRI), an independent, non-profit research organization in the energy industry. Mr. Lindsey has been a researcher at EPRI for over 10 years where he manages projects relating to how end-use electric technologies can be used to support energy efficiency, decarbonization, and human health. Recent topics of research include disinfection lighting, indoor food production with LEDs, advances in heat pump water heating, and efficient window heat pumps.
Annette Rohr
Annette Rohr is a senior program manager at the Electric Power Research Institute (EPRI). After earning her doctorate from the Harvard T.H. Chan School of Public Health, she joined the world's leading independent, non-profit energy research and development organization where her scientific focus is on the intersection of energy and environmental health.
Matthew D. Moore
Matthew D. Moore is an assistant professor at the University of Massachusetts, Amherst. He received a B.S. in Food Science from Cornell University in 2010. He then received his Ph.D. in Food Science at North Carolina State University under the supervision of Dr. Lee-Ann Jaykus in 2016. After conducting research in the Jaykus lab as a postdoctoral scholar, Dr. Moore joined the National Antimicrobial Resistance Surveillance Team at the U.S. Centers for Disease Control and Prevention as an ORISE Postdoctoral Fellow in 2017. He then joined the Department of Food Science at the University of Massachusetts, Amherst in 2018.
Petros Koutrakis
Petros Koutrakis is a professor at the Harvard T.H. Chan School of Public Health. There, he is the Head of the Exposure, Epidemiology and Risk Program and the Director of the EPA/Harvard University Center for Ambient Particle Health Effects. His research activities focus on the development of human exposure measurement techniques and the investigation of sources, transport, and the fate of air pollutants.
Ed Nardell
Ed Nardell is a professor at Harvard Medical School and Harvard’s Chan School of Public Health. His research interests include tuberculosis control airborne transmission, and germicidal air disinfection. He has been principal investigator of a long-term human-to-animal airborne transmission facility near Pretoria, South Africa.
Philip Demokritou
Philip Demokritou is the founder of the Rutgers Nanoscience and Advanced Materials Research Center at Rutgers university. He is also the founding director of the Center for Nanotechnology and Nanotoxicology at Harvard university. His current nanoscience research includes synthesis of nanomaterials using flame spray pyrolysis, nature derived biopolymers for agri-food applications and environmental nanotechnology applications for pathogen inactivation, sustainable food package materials and agri-chemical delivery using nature derived biopolymers. He holds more than a dozen international/US patents and inventions. He is a co-author of two books, numerous book chapters, and more than two hundred articles in leading journals in nanoscience, particle health effect, and aerosol engineering fields.
References
- Beck, S. E., R. A. Rodriguez, M. A. Hawkins, T. M. Hargy, T. C. Larason, and K. G. Linden. 2016a. Comparison of UV-induced inactivation and RNA damage in MS2 phage across the germicidal UV spectrum. Appl. Environ. Microbiol. 82 (5):1468–74. American Society for Microbiology. doi:10.1128/AEM.02773-15.
- Beck, S. E., R. A. Rodriguez, M. A. Hawkins, T. M. Hargy, T. C. Larason, and K. G. Linden. 2016b. Comparison of UV-induced inactivation and RNA damage in MS2 phage across the germicidal UV spectrum. Appl. Environ. Microbiol. 82 (5):1468–74. American Society for Microbiology. doi:10.1128/AEM.02773-15.
- Biasin, M., A. Bianco, G. Pareschi, A. Cavalleri, C. Cavatorta, C. Fenizia, P. Galli, L. Lessio, M. Lualdi, E. Tombetti, et al. 2021. UV-C irradiation is highly effective in inactivating SARS-CoV-2 replication. Sci. Rep. 11 (1):1–7. Nature Publishing Group. doi:10.1038/s41598-021-85425-w.
- Boegel, S. J., M. Gabriel, M. Sasges, B. Petri, M. R. D’Agostino, A. Zhang, J. C. Ang, M. S. Miller, S. M. Meunier, and M. G. Aucoin. 2021. Robust evaluation of ultraviolet-C sensitivity for SARS-CoV-2 and surrogate coronaviruses. Microbiol. Spectr. 9(2). American Society for Microbiology. doi:10.1128/spectrum.00537-21.
- Bonny, T. S., S. Yezli, and J. A. Lednicky. 2018. Isolation and identification of human coronavirus 229E from frequently touched environmental surfaces of a university classroom that is cleaned daily. Am. J. Infect. Control 46 (1):105–07. Mosby. doi:10.1016/j.ajic.2017.07.014.
- Buonanno, M., B. Ponnaiya, D. Welch, M. Stanislauskas, G. Randers-Pehrson, L. Smilenov, F. D. Lowy, D. M. Owens, and D. J. Brenner. 2017. Germicidal efficacy and mammalian skin safety of 222-Nm UV light. Radiat. Res. 187 (4):483–91. Allen Press. doi:10.1667/RR0010CC.1.
- Buonanno, M., D. Welch, I. Shuryak, and D. J. Brenner. 2020. Far-UVC light (222 Nm) efficiently and safely inactivates airborne human coronaviruses. Sci. Rep. 10 (1):1–8. Nature Publishing Group. doi:10.1038/s41598-020-67211-2.
- Carraturo, F., C. Del Giudice, M. Morelli, V. Cerullo, G. Libralato, E. Galdiero, and M. Guida. 2020. Persistence of SARS-CoV-2 in the environment and COVID-19 transmission risk from environmental matrices and surfaces. Environ. Pollut. 265:115010. doi:10.1016/j.envpol.2020.115010.
- CDC. 2022. CDC COVID data tracker. Centers for Disease Control and Prevention. Accessed May 6, 2022. https://covid.cdc.gov/covid-data-tracker/#datatracker-home.
- Chiappa, F., B. Frascella, G. P. Vigezzi, M. Moro, L. Diamanti, L. Gentile, P. Lago, N. Clementi, C. Signorelli, N. Mancini, et al. 2021. The efficacy of ultraviolet light-emitting technology against coronaviruses: A systematic review. J. Hosp. Infect. 114:63–78. doi:10.1016/j.jhin.2021.05.005.
- Cimolai, N. 2020. Environmental and decontamination issues for human coronaviruses and their potential surrogates. J. Med. Virol. 92 (11):2498–510. doi:10.1002/jmv.26170.
- Coohill, T. P. 1986. Virus-cell interactions as probes for vacuum-ultraviolet radiation damage and repair. Photochem. Photobiol. 44 (3):359–63. John Wiley & Sons, Ltd. doi:10.1111/J.1751-1097.1986.TB04676.X.
- Darnell, M. E. R., K. Subbarao, S. M. Feinstone, and D. R. Taylor. 2004. Inactivation of the coronavirus that induces severe acute respiratory syndrome, SARS-CoV. J. Virol. Methods 121 (1):85–91. doi:10.1016/j.jviromet.2004.06.006.
- Enwemeka, C. S., V. V. Bumah, and J. L. Mokili. 2021. Pulsed blue light inactivates two strains of human coronavirus. J. Photochem. Photobiol. B Biol. 222 (September):112282. Elsevier. doi:10.1016/j.jphotobiol.2021.112282.
- Freeman, S., K. Kibler, Z. Lipsky, S. Jin, G. K. German, and K. Ye. 2022. Systematic evaluating and modeling of SARS-CoV-2 UVC disinfection. Sci. Rep. 12 (1):1–13. Nature Publishing Group. doi:10.1038/s41598-022-09930-2.
- Gerchman, Y., H. Mamane, N. Friedman, and M. Mandelboim. 2020. UV-LED disinfection of coronavirus: Wavelength effect. J. Photochem. Photobiol. B Biol. 212 (November):112044. Elsevier. doi: 10.1016/j.jphotobiol.2020.112044.
- Goldfarb, A. R., and L. J. Saidel. 1951. Ultraviolet absorption spectra of proteins. Science (80-) 114 (2954):156–57. American Association for the Advancement of Science. doi:10.1126/science.114.2954.156.
- Guffey, J. S., and J. Wilborn. 2006. In vitro bactericidal effects of 405-Nm and 470-Nm blue light. Photomed. Laser Surg. 24 (6):684–88. Photomed Laser Surg. doi:10.1089/pho.2006.24.684.
- Heilingloh, C. S., U. W. Aufderhorst, L. Schipper, U. Dittmer, O. Witzke, D. Yang, X. Zheng, K. Sutter, M. Trilling, M. Alt, et al. 2020. Susceptibility of SARS-CoV-2 to UV irradiation. Am. J. Infect. Control 48 (10):1273–75. Mosby Inc. doi:10.1016/j.ajic.2020.07.031.
- Huang, R., N. Vaze, A. Soorneedi, M. D. Moore, Y. Luo, E. Poverenov, V. Rodov, and P. Demokritou. 2021. A novel antimicrobial technology to enhance food safety and quality of leafy vegetables using engineered water nanostructures. Environ. Sci. Nano. 8 (2):514–26. Royal Society of Chemistry (RSC). doi:10.1039/d0en00814a.
- Huang, R., N. Vaze, A. Soorneedi, M. D. Moore, Y. Xue, D. Bello, and P. Demokritou. 2019. Inactivation of hand hygiene-related pathogens using engineered water nanostructures. ACS Sustain. Chem. Eng. 7 (24):19761–69. doi:10.1021/acssuschemeng.9b05057.
- Inagaki, H., A. Saito, H. Sugiyama, T. Okabayashi, and S. Fujimoto. 2020. Rapid inactivation of SARS-CoV-2 with deep-UV LED irradiation. Emerg. Microbes Infect. 9 (1):1744–47. Emerg Microbes Infect. doi:10.1080/22221751.2020.1796529.
- Jones, J. P., and K. Norton. 2021. 222-Nm ultraviolet light inactivates dried inocula of human rhinovirus and human coronavirus on a glass carrier. J. Hosp. Infect. 117 (November):190–91. Elsevier: 190. doi:10.1016/J.JHIN.2021.08.003.
- Kitagawa, H., T. Nomura, T. Nazmul, K. Omori, N. Shigemoto, T. Sakaguchi, and H. Ohge. 2021. Effectiveness of 222-Nm ultraviolet light on disinfecting SARS-CoV-2 surface contamination. Am. J. Infect. Control 49 (3):299–301. Mosby Inc. doi:10.1016/J.AJIC.2020.08.022.
- Ko, G., M. W. First, and H. A. Burge. 2000. Influence of relative humidity on particle size and UV sensitivity of serratia marcescens and mycobacterium bovis BCG aerosols. Tuber. Lung Dis. 80 (4–5):217–28. doi:10.1054/tuld.2000.0249.
- Mackenzie, D. 2020. Ultraviolet light fights new virus. Engineering 6 (8):851–53. Elsevier. doi:10.1016/j.eng.2020.06.009.
- Mahase, E. 2021. Covid-19: What new variants are emerging and how are they being investigated? BMJ 372 (January):n158. British Medical Journal Publishing Group. doi:10.1136/bmj.n158.
- Malik, Y. A. 2020. Properties of coronavirus and SARS-CoV-2. Malays. J. Pathol. 42 (1):3–11. Malaysian Society of Pathologists. https://europepmc.org/article/med/32342926.
- McDevitt, J. J., M. L. Ka, S. N. Rudnick, E. A. Houseman, M. W. First, and D. K. Milton. 2007. Characterization of UVC light sensitivity of vaccinia virus. Appl. Environ. Microbiol. 73 (18):5760–66. American Society for Microbiology. doi:10.1128/AEM.00110-07.
- McDevitt, J. J., S. N. Rudnick, and L. J. Radonovich. 2012. Aerosol susceptibility of influenza virus to UV-C light. Appl. Environ. Microbiol. 78 (6):1666–69. American Society for Microbiology. doi:10.1128/AEM.06960-11.
- McKenzie, K., M. Maclean, M. H. Grant, P. Ramakrishnan, S. J. MacGregor, and J. G. Anderson. 2016. The effects of 405 Nm light on bacterial membrane integrity determined by salt and bile tolerance assays, leakage of UV-Absorbing material and SYTOX green labelling. Microbiol. (United Kingdom) 162 (9):1680–88. Microbiology Society. doi:10.1099/mic.0.000350.
- Meyers, C., R. Robison, J. Milici, S. Alam, D. Quillen, D. Goldenberg, and R. Kass. 2021. Lowering the transmission and spread of human coronavirus. J. Med. Virol. 93 (3):1605–12. doi:10.1002/jmv.26514.
- Minamikawa, T., T. Koma, A. Suzuki, T. Mizuno, K. Nagamatsu, H. Arimochi, K. Tsuchiya, K. Matsuoka, T. Yasui, K. Yasutomo, et al. 2021. Quantitative evaluation of SARS-CoV-2 inactivation using a deep ultraviolet light-emitting diode. Sci. Rep. 11 (1):1–9. Nature Publishing Group. doi:10.1038/s41598-021-84592-0.
- Moschovis, P. P., L. M. Yonker, J. Shah, D. Singh, P. Demokritou, and T. B. Kinane. 2021. Aerosol transmission of SARS-CoV-2 by children and adults during the COVID-19 pandemic. Pediatr. Pulmonol. 56 (6):1389–94. doi:10.1002/ppul.25330.
- Pyrgiotakis, G., P. Vedantam, C. Cirenza, J. McDevitt, M. Eleftheriadou, S. S. Leonard, and P. Demokritou. 2016. Optimization of a nanotechnology based antimicrobial platform for food safety applications using engineered water nanostructures (EWNS). Sci. Rep. 6 (October 2015):21073. Nature Publishing Group. doi:10.1038/srep21073.
- Rathnasinghe, R., S. Jangra, L. Miorin, M. Schotsaert, C. Yahnke, and A. Garcίa-Sastre. 2021. The virucidal effects of 405 Nm visible light on SARS-CoV-2 and influenza a virus. Sci. Rep. 11 (1):1–10. Nature Publishing Group. doi:10.1038/s41598-021-97797-0.
- Reed, N. G. 2010. The history of ultraviolet germicidal irradiation for air disinfection. Public Health Rep. 125 (1):15–27. SAGE Publications. doi:10.1177/003335491012500105.
- Reshi, M. L., Y. C. Su, and J. R. Hong. 2014. RNA viruses: ROS-mediated cell death. Int. J. Cell Biol. 2014:1–16. doi:10.1155/2014/467452.
- Review of ANSI/ASHRAE standard 62.1-2004: Part 2 - Workplace hygiene. 2022. Accessed May 10, 2022. https://workplace-hygiene.com/review-ansiashrae-standard-62-1-2004-part-2/.
- Ruetalo, N., R. Businger, and M. Schindler. 2020. Rapid and efficient inactivation of surface dried SARS-CoV-2 by UV-C irradiation. BioRxiv, September. Cold Spring Harbor Laboratory, 2020.09.22.308098. doi:10.1101/2020.09.22.308098.
- Simmons, S. E., R. Carrion, K. J. Alfson, H. M. Staples, C. Jinadatha, W. R. Jarvis, P. Sampathkumar, R. F. Chemaly, F. Khawaja, M. Povroznik, et al. 2021. Deactivation of SARS-CoV-2 with pulsed-xenon ultraviolet light: Implications for environmental COVID-19 control. Infect. Control Hosp. Epidemiol. 42 (2):127–30. Cambridge University Press. doi:10.1017/ice.2020.399.
- Stein, B., H. J. Rahmsdorf, A. Steffen, M. Litfin, and P. Herrlich. 1989. UV-Induced DNA damage is an intermediate step in UV-induced expression of human immunodeficiency virus type 1, collagenase, c-Fos, and metallothionein. Mol. Cell. Biol. 9 (11):5169–81. doi:10.1128/mcb.9.11.5169-5181.1989.
- Storm, N., L. G. A. McKay, S. N. Downs, R. I. Johnson, D. Birru, M. de Samber, W. Willaert, G. Cennini, and A. Griffiths. 2020. Rapid and complete inactivation of SARS-CoV-2 by ultraviolet-C irradiation. Sci. Rep. 10(1). Nature Publishing Group. doi:10.1038/s41598-020-79600-8.
- Vaze, N., and P. Demokritou. 2022. Using engineered water nanostructures (EWNS) for wound disinfection: Case study of Acinetobacter baumannii inactivation on skin and the inhibition of biofilm formation. Nanomed. Nanotechnol. Biol. Med. 42 (June):102537. Elsevier. doi: 10.1016/j.nano.2022.102537.
- Vaze, N., Y. Jiang, L. Mena, Y. Zhang, D. Bello, S. S. Leonard, A. M. Morris, M. Eleftheriadou, G. Pyrgiotakis, and P. Demokritou. 2018. An integrated electrolysis – electrospray – ionization antimicrobial platform using engineered water nanostructures (EWNS) for food safety applications. Food Control 85 (March):151–60. Elsevier. doi:10.1016/j.foodcont.2017.09.034.
- Vaze, N., G. Pyrgiotakis, J. McDevitt, L. Mena, A. Melo, A. Bedugnis, L. Kobzik, M. Eleftheriadou, and P. Demokritou. 2019a. Inactivation of common hospital acquired pathogens on surfaces and in air utilizing engineered water nanostructures (EWNS) based nano-sanitizers. Nanomed. Nanotechnol. Biol. Med. 18:234–42. doi:10.1016/j.nano.2019.03.003.
- Vaze, N., G. Pyrgiotakis, J. McDevitt, L. Mena, A. Melo, A. Bedugnis, L. Kobzik, M. Eleftheriadou, and P. Demokritou. 2019b. Inactivation of common hospital acquired pathogens on surfaces and in air utilizing engineered water nanostructures (EWNS) based nano-sanitizers. Nanomed. Nanotechnol. Biol. Med. 18 (March):234–42. doi:10.1016/j.nano.2019.03.003.
- Vaze, N., G. Pyrgiotakis, L. Mena, R. Baumann, A. Demokritou, M. Ericsson, Y. Zhang, D. Bello, M. Eleftheriadou, and P. Demokritou. 2019. A nano-carrier platform for the targeted delivery of nature-inspired antimicrobials using engineered water nanostructures for food safety applications. Food Control 96:365–74. doi:10.1016/j.foodcont.2018.09.037.
- Vaze, N., A. R. Soorneedi, M. D. Moore, and P. Demokritou. 2022. Inactivating SARS-CoV-2 surrogates on surfaces using engineered water nanostructures incorporated with nature derived antimicrobials. Nanomaterials 12 (10):1735. Multidisciplinary Digital Publishing Institute. doi:10.3390/nano12101735.
- Vecchi, C., G. Montosi, and A. Pietrangelo. 2010. Huh-7: A human “Hemochromatotic” cell line. Hepatology 51 (2):654–59. doi:10.1002/hep.23410.
- Walker, C. M., and G. Ko. 2007. Effect of ultraviolet germicidal irradiation on viral aerosols. Environ. Sci. Technol. 41 (15):5460–65. American Chemical Society. doi:10.1021/es070056u.