ABSTRACT
Acute kidney injury (AKI) necessitating renal-replacement therapy has been associated with high mortality rates in critically ill patients. Usual methods to study AKI encompass the assessment of serum and urine biomarkers. Hypoxia is a major pathophysiological feature of AKI, which necessitates continuous bedside monitoring of renal tissue oxygenation in intensive care unit (ICU) patients. Research has made continuous bladder urine oxygen pressure (PuO2) monitoring possible in humans. Although the value of bladder PuO2 does not represent an absolute value of medullary tissue oxygen pressure (Po2), bladder PuO2 can be considered a window into the renal medullary oxygenation. Bladder PuO2 can be monitored by using probes with oxygen sensors inserted into the urinary bladder. Additionally, PuO2 can be measured manually by using a blood gas analyzer machine. PuO2 monitoring can be potentially helpful in early diagnosis and/or prevention of AKI and guide therapeutic interventions aimed at improving renal oxygen delivery in those patients.
1. Introduction
AKI is a common finding in hospitalized patients, particularly in septic cohorts. Angus et al. reviewed the diagnostic codes of 192,980 patients with serious sepsis from seven US states; AKI was found in 22% of the patients and had a mortality rate of 38.2%[Citation1]. In the acutely ill patients (SOAP) study, patients recruited from Europe and admitted to 198 ICUs, sepsis was reported among 37% of 3147 patients. In 51% of the cases, AKI occurred and was associated with 41% ICU mortality [Citation2]. In the FINNAKI study, 2901 seriously ill patients have been studied across 17 Finnish ICUs. Among the most critical 918 serious sepsis patients, 53% of them met the KDIGO guidelines for AKI [Citation3].
In septic AKI experimental research, endotoxin administration had decreased global renal blood flow (RBF), which was connected to a hypodynamic systemic circulation. A conclusion was made that septic human AKI is attributed to renal vasoconstriction and ischemia [Citation4]. On the other hand, in a study conducted on 160 original animal models, RBF was found either preserved or increased if the animal model had high cardiac output (CO). However, oliguria and AKI progressed within hours and were marked despite such global renal hyperemia. A phenomenon in which RBF is distinguished from glomerular filtration rate (GFR) was revealed [Citation5]. Ischemia can still occur in spite of increased RBF. This was explained by experimental research which proved a blood flow redistribution towards the renal cortex at the expense of renal medullary blood flow [Citation6–8]. Additionally, changes in regional distribution of blood flow imply the activation of intrarenal shunting pathways and, consequently, renal medullary hypoxia ensues [Citation9].
In postmortem human and experimental septic AKI, acute tubular necrosis (ATN) was found to be rare [Citation10,Citation11]. However, in septic kidneys, mild tubular damage, leucocyte infiltration, and apoptosis were reported in postmortem autopsy [Citation12].
The diagnosis of AKI has been based primarily on serum creatinine over the last 50 years [Citation13]. It is well known that a significant decrease in GFR leads to an increased serum creatinine level. In the last 10 years, a lot has been done to find specific biomarkers to identify acute damage in the renal tubular epithelium [Citation14,Citation15]. The majority of studies seek to discover and confirm biomarkers in large cohorts of patients using highly efficient techniques. Very few studies have reviewed the AKI biomarker clinical application to improve AKI treatment and patients’ outcome. In both blood and urine, biomarkers can be detected. Urine biomarkers have nevertheless been examined most thoroughly as the urine is closest to the injury site.
Neutrophil gelatinase-associated lipocalin (NGAL) was investigated most extensively. Diverse patient populations were analyzed by various investigators, and NGAL measurements for different indications were determined. It is obvious that NGAL is expressed in AKI in a severity-related way, such as after contrast, after cardiac surgery, and after renal transplantation. Dehydration alone does not cause the expression of NGAL. Notably, the cut-off values for NGAL in AKI are uncertain due to the variety of test kits on the market [Citation16–23].
AKI biomarkers discovery is concerned with the desire for early diagnosis to provide early prevention and treatment. Biomarkers can supply AKI pathophysiology insights and provide additional functional testing [Citation24]. The ideal biomarkers should detect renal stress before functional damage is apparent or also in the absence of preclinical AKI. The levels of biomarkers should also help in diagnosing the cause of oliguria. The scope of research should include a transition from monitoring physiological biomarkers of adequate renal perfusion to pathophysiologic biomarkers of renal hypoperfusion and finally biomarkers of kidney cell structural injury or damage [Citation25].
The main goal of this review is to address the question as to whether minimally invasive, bedside continuous bladder PuO2 monitoring would be worthwhile in patients at high risk of developing AKI.
2. The role of using continuous bladder PuO2 measurements in early detection of AKI
Hypoxia in the renal medulla is a hallmark of AKI of diverse etiologies. The kidneys are vulnerable to hypoxia due to their role as an oxygen sensor designed to sense the decrease in renal tissue Po2 in case of hypoxemia, which stimulates erythropoietin production for increased erythropoiesis. However, the absence of innate renal feedback mechanisms capable of increasing renal oxygen delivery or decreasing renal oxygen consumption makes the kidney highly susceptible to hypoxia [Citation26]. The kidney is also susceptible to hypoxia due to a large metabolic demand imposed by active reabsorption of sodium, which ultimately increases oxygen consumption. Limitations on oxygen delivery to cortical tissue are imposed by the density of peritubular capillaries. Moreover, oxygen is shunted between arteries and nearby veins in the renal cortex as well as between the descending and ascending vasa recta in the renal medulla () [Citation27,Citation28].
Figure 1. Diffusion of oxygen inside the renal parenchyma (O2: oxygen, Po2: oxygen pressure, and PuO2: urinary oxygen pressure). A: oxygen diffusion through Bowman’s capsule, B: oxygen shunting between arteries and veins in the cortex, C: oxygen shunting between descending and ascending vasa recta in the medulla, and D: oxygen diffusion between the renal medulla and renal pelvis
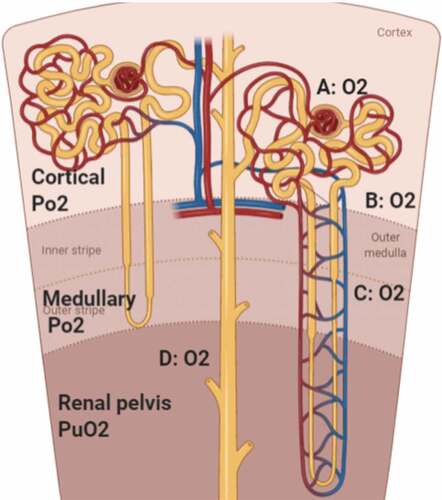
Renal medullary mean Po2 reflects the balance between renal medullary oxygen delivery and oxygen consumption [Citation29,Citation30]. Medullary Po2 is equal to PuO2 in the renal pelvis (); however, measurement of pelvic PuO2 is technically difficult as it requires the insertion of a nephrostomy tube for collecting urine from the renal pelvis [Citation31]. Pelvic PuO2 decreases depending on the distance from the pelvis to the bladder [Citation32] (). The difference between pelvic and bladder PuO2 may be significant during diuresis and in the presence of some pathological conditions, or with various oxygen concentrations of inspired gas [Citation33] (). Although the value of bladder PuO2 does not represent an absolute value of medullary Po2, variation over time in bladder PuO2 measurements can unmask changes in medullary tissue Po2. A decrease in bladder PuO2 measurements over time may reflect decreased oxygen delivery to the renal medullary tissue or increased oxygen consumption of the renal medullary tissue.
Figure 2. Oxygen flow through the urinary tract starting from renal medulla down to the urinary bladder; 1: renal medullary tissue Po2, 2: pelvic PuO2, 3: upper ureteric PuO2, 4: lower ureteric PuO2, and 5: bladder PuO2. (PuO2: urinary oxygen pressure.)
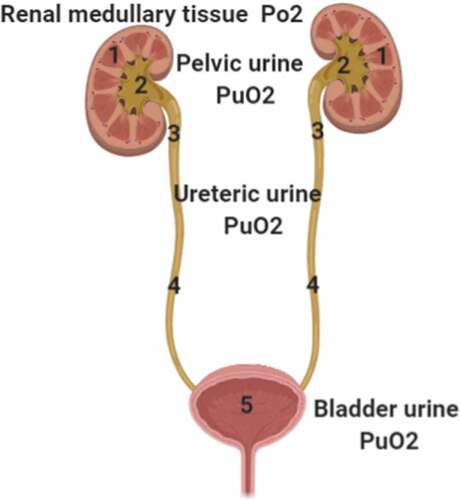
Continuous monitoring of the bladder PuO2 may potentially serve as a significant clinical tool for monitoring the adequacy of renal tissue oxygenation in critically ill patients who are at risk of developing AKI. Moreover, continuous monitoring of bladder PuO2 is a relatively noninvasive technique, which could provide potentially important real-time data regarding renal medullary tissue oxygenation in ICU patients [Citation32,Citation34,Citation35].
Our suggested method for continuous monitoring of bladder PuO2 encompasses the insertion of an oxygen-sensing probe into the urinary bladder through a urinary catheter. The sensing probe should be kept in contact with urine while trying to avoid contact with the walls of the urinary bladder, so that it measures bladder PuO2 and not urinary bladder wall Po2 (). The measuring probe can then be interfaced with a monitor screen, which displays bladder PuO2 measurements continuously, thus allowing clinicians to follow the trend and anticipate the changes in renal medullary oxygenation over time. The probe method has been used previously by Morelli et al., Osawa et al., and Zhu et al. () [Citation33,Citation36,Citation37]. The measuring probe should ideally be sensitive, easily calibrated, not affected by acidic urine, and not fragile as to be easily broken by kinking.
Table 1. Summary of previous studies that monitored urinary oxygen pressure (PuO2). ICU: intensive care unit, PuO2: urinary oxygen pressure, AKI: acute kidney injury, GFR: glomerular filtration rate, RBF: renal blood flow, Po2: oxygen pressure, Hct: hematocrit, Hb: hemoglobin, PRBC: packed red blood concentrate, and CaO2: arterial oxygen content
Figure 3. Oxygen flow from the ureters to the urinary bladder and oxygen-sensing probe passing through the urinary catheter with its tip located inside the urinary bladder to measure bladder PuO2
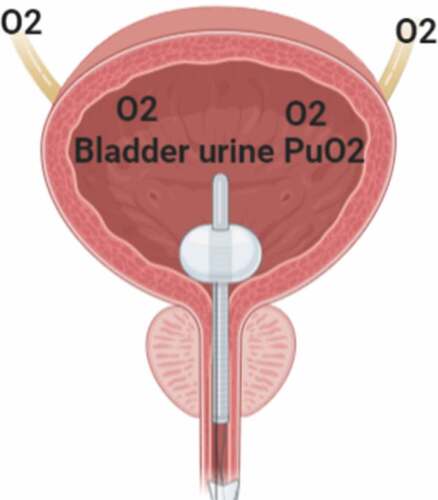
Bladder PuO2 monitoring can be confounded by multiple factors, including systemic oxygenation, perfusion, diuretics, urinary tract infections, chronic renal impairments, local diseases of the urinary tract, renal metabolic state, oxygenation within the ureteric wall, and urine flow. At low urine flow, the signal may be lost [Citation38,Citation39]; consequently, measurement of bladder PuO2 will have little or no utility in patients who have already developed AKI ().
Alternatively, bladder PuO2 can also be measured by collecting urine samples manually from the urinary catheter, which can then be measured by a gas analyzer. This method was used by Kitashiro et al [Citation30] . and Valente et al [Citation40] . (). This manual method is easy and inexpensive, can be done in every ICU, and does not require special equipment. However, the possible air entrainment into the sampling syringe and calibration of the gas analyzer machine may influence the accuracy of PuO2 measurements.
3. A summary of previous studies employing PuO2
Studies in experimental hyperdynamic septic AKI have shown that, even in the presence of increased global RBF and oxygen supply, the renal medulla is especially vulnerable to hypoxia during early sepsis [Citation41]. Progressive renal medullary hypoxia leads to oxidative stress and inflammation, which can initiate renal cellular injury and finally AKI [Citation8,Citation41,Citation42].
In an animal study of bovine sepsis with AKI [Citation8], resuscitation of septic shock with norepinephrine improved arterial blood pressure and resulted in transient improvement in renal function. However, the use of norepinephrine was associated with further worsening of kidney function due to a decrease in renal medullary tissue perfusion and medullary tissue Po2 independent of changes in RBF and renal oxygen delivery, indicating that whole kidney measures of oxygenation cannot be used to predict the changes in medullary perfusion and oxygenation. Interestingly, measured PuO2 was closely related to medullary tissue Po28.
Moreover, in animal studies with septic AKI, distinct effects on renal medullary tissue Po2 were demonstrated by using the following therapies: fluids, norepinephrine, vasopressin, angiotensin II, and furosemide [Citation41].
Similarly, PuO2 was found to increase after the administration of fenoldopam to stable critically ill patients, which was not related to increases in systemic perfusion and cardiac function [Citation33] (Table1). Comparably, furosemide administration to patients with septic shock was associated with greater diuresis and an increase in bladder PuO236 (Table1).
Surprisingly, in an experimental study on septic AKI in conscious sheep, the decrease in medullary tissue Po2 and PuO2 was detected several hours before the increase in urinary NGAL and serum creatinine. Additionally, intravenous infusion of angiotensin II could restore arterial pressure and improve creatinine clearance without exacerbating medullary or urinary hypoxia [Citation43].
In a recent experimental study [Citation42] on conscious sheep model with septic AKI, fluid bolus therapy with 500 mL of Hartmann’s solution over 15 min was associated with increased blood pressure, central venous pressure, CO, medullary Po2, PuO2, and creatinine clearance at 30 minutes. Unanticipatedly, the improvement in medullary oxygenation had disappeared thereafter, and the studied animals had sodium and volume retention after two boluses [Citation42] ().
Consequently, the optimal choice of therapeutic intervention should aim to restore and maintain adequate renal medullary microcirculation without worsening medullary hypoxia [Citation41].
Continuous monitoring of bladder PuO2 is found to be a useful tool for evaluating the balance between renal oxygen supply and demand in stable critically ill patients [Citation33] (). Moreover, bladder PuO2 was found to be low when measured in ICU patients with septic shock [Citation36] (). Thus, continuous PuO2 monitoring could be a perfect monitoring tool during the treatment of septic AKI [Citation41].
As initial serum creatinine lags behind the onset of renal tubular injury [Citation44], bladder PuO2 may initially aid in the adjustment of fluid intake by ensuring appropriate volume resuscitation to the patients and avoiding volume overload, which may further compromise CO and worsen the acute lung injury. As such, bladder PuO2 should be incorporated in future randomized clinical studies to investigate its value in AKI prediction and management.
4. Conclusion
Continuous bladder PuO2 monitoring is a minimally invasive and potentially useful tool for early detection, prevention, and management of AKI. Therefore, its use in patients with early stages of AKI could help in elucidating the pathogenesis of AKI in at-risk patients, as well as aiding in the early diagnosis of acute renal failure and establishing the most appropriate therapeutic interventions.
Disclosure statement
The authors declare no potential conflicts of interest with respect to the research, authorship, and publication of this article.
Additional information
Funding
References
- Angus DC, et al. Epidemiology of severe sepsis in the United States: analysis of incidence, outcome, and associated costs of care. Read Online Crit. Care Med. Soc. Crit. Care Med. 2001;29:1303–1310.
- Vincent J-L, et al. Sepsis in European intensive care units: results of the SOAP study. Crit. Care Med. 2006;34(2):344–353.
- Poukkanen M, et al. Acute kidney injury in patients with severe sepsis in F innish I ntensive C are U nits. Acta Anaesthesiol. Scand. 2013;57(7):863–872.
- Schrier RW, Wang W. Acute renal failure and sepsis. N. Engl. J. Med. 2004;351(2):159–169.
- Di Giantomasso D, May CN, Bellomo R. Vital organ blood flow during hyperdynamic sepsis. Chest. 2003;124(3):1053–1059.
- Post EH, Kellum JA, Bellomo R, et al. Renal perfusion in sepsis: from macro-to microcirculation. Kidney Int. 2017;91(1):45–60.
- Calzavacca P, Evans RG, Bailey M, et al. Cortical and medullary tissue perfusion and oxygenation in experimental septic acute kidney injury. Crit. Care Med. 2015;43(10):e431–e439.
- Lankadeva YR, et al. Intrarenal and urinary oxygenation during norepinephrine resuscitation in ovine septic acute kidney injury. Kidney Int. 2016;90(1):100–108.
- Casellas D, Mimran A. Aglomerular pathways in intrarenal microvasculature of aged rats. American Journal of Anatomy. 1979;156(2):293–299.
- Lerolle N, et al. Histopathology of septic shock induced acute kidney injury: apoptosis and leukocytic infiltration. Intensive Care Med. 2010;36(3):471–478.
- Takasu O, et al. Mechanisms of cardiac and renal dysfunction in patients dying of sepsis. Am. J. Respir. Crit. Care Med. 2013;187(5):509–517.
- Langenberg C, Gobe G, Hood S, et al. Renal histopathology during experimental septic acute kidney injury and recovery. Crit. Care Med. 2014;42(1):e58–e67.
- Kellum JA, et al. Kidney disease: improving global outcomes (KDIGO) acute kidney injury work group. KDIGO clinical practice guideline for acute kidney injury. Kidney Int Suppl. 2012;2:1–138.
- Schrezenmeier EV, Barasch J, Budde K, et al. Biomarkers in acute kidney injury–pathophysiological basis and clinical performance. Acta Physiol. 2017;219(3):556–574.
- Alge JL, Arthur JM. Biomarkers of AKI: a review of mechanistic relevance and potential therapeutic implications. Clin. J. Am. Soc. Nephrol. 2015;10(1):147–155.
- Parikh CR, et al. Postoperative biomarkers predict acute kidney injury and poor outcomes after adult cardiac surgery. J. Am. Soc. Nephrol. 2011;22(9):1748–1757.
- Dent CL, et al. Plasma neutrophil gelatinase-associated lipocalin predicts acute kidney injury, morbidity and mortality after pediatric cardiac surgery: a prospective uncontrolled cohort study. Crit Care. 2007;11(6):R127.
- Bennett M, et al. Urine NGAL predicts severity of acute kidney injury after cardiac surgery: a prospective study. Clin. J. Am. Soc. Nephrol. 2008;3(3):665–673.
- Haase M, et al. Accuracy of neutrophil gelatinase-associated lipocalin (NGAL) in diagnosis and prognosis in acute kidney injury: a systematic review and meta-analysis. Am. J. Kidney Dis. 2009;54(6):1012–1024.
- Ho J, et al. Urinary, plasma, and serum biomarkers’ utility for predicting acute kidney injury associated with cardiac surgery in adults: a meta-analysis. Am. J. Kidney Dis. 2015;66(6):993–1005.
- Pons B, et al. Diagnostic accuracy of early urinary index changes in differentiating transient frompersistent acute kidney injury in critically ill patients: multicenter cohortstudy. Crit Care. 2013;17(2):R56.
- Darmon M, et al. Diagnostic performance of fractional excretion of urea in the evaluation of critically ill patients with acute kidney injury: a multicenter cohort study. Crit Care. 2011;15(4):R178.
- Srisawat N, Kellum JA. The role of biomarkers in acute kidney injury. Crit. Care Clin. 2020;36(1):125–140.
- McCullough PA, et al. Diagnosis of acute kidney injury using functional and injury biomarkers: workgroup statements from the tenth acute dialysis quality initiative consensus conference. In: ADQI consensus on AKI biomarkers and cardiorenal syndromes. Vol. 182. Karger Publishers; 2013. p. 13–29.
- Okusa MD, et al. Physiological biomarkers of acute kidney injury: a conceptual approach to improving outcomes. Contrib Nephrol. 2013;182:65–81.
- Evans RG, Goddard D, Eppel GA, et al. Factors that render the kidney susceptible to tissue hypoxia in hypoxemia. Am. J. Physiol. - Regul. Integr. Comp. Physiol. 2011;300(4):931–940.
- Evans RG, Gardiner BS, Smith DW, et al. Intrarenal oxygenation: unique challenges and the biophysical basis of homeostasis. Am. J. Physiol. - Ren. Physiol. 2008;295(5):F1259-F1270.
- Evans RG, Smith DW, Lee CJ, et al. What makes the kidney susceptible to hypoxia? Anat. Rec. 2019. DOI:10.1002/ar.24260
- Kainuma M, Kimura N, Shimada Y. Effect of acute changes in renal arterial blood flow on urine oxygen tension in dogs. Crit. Care Med. 1990;18(3):309–312.
- Kitashiro S, et al. Clinical significance of the urinary oxygen tension in patients with ischemic heart disease. Cardiology. 1997;88(6):540–543.
- Tolley PM, Purcell A, Bolsin SN. Effect of i.v. furosemide on pelvic urinary oxygen tension in humans. Br. J. Anaesth. 1999;83(2):328–329.
- Leonhardt KO, Landes RR Oxygen tension of the urine and renal structures: preliminary report of clinical findings. N. Engl. J. Med. 269, 115–121 (1963).
- Morelli A, et al. Monitoring renal oxygen supply in critically-iii patients using urinary oxygen tension. Anesth. Analg. 2003;97(6):1764–1768.
- Leonhardt KO, Landes RR, McCauley RT. Anatomy and physiology of intrarenal oxygen tensionpreliminary study of the effects of anesthetics. Anesthesiol. J. Am. Soc. Anesthesiol. 1965;26:648–658.
- Aperia AC, Liebow AA. Implications of urine pO2 for renal medullary blood flow. Am. J. Physiol. Content. 1964;206(3):499–504.
- Osawa EA, et al. Effect of furosemide on urinary oxygenation in patients with septic shock. Blood Purif. 2019;48(4):336–345.
- Zhu MZL, et al. Urinary hypoxia: an intraoperative marker of risk of cardiac surgery-associated acute kidney injury. Nephrol. Dial. Transplant. 2018;33(12):2191–2201.
- Sgouralis I, et al. Bladder urine oxygen tension for assessing renal medullary oxygenation in rabbits: experimental and modeling studies. Am. J. Physiol. - Regul. Integr. Comp. Physiol. 2016;311(3):R532–R544.
- Giannakopoulos X, et al. Human bladder urine oxygen content: implications for urinary tract diseases. Int. Urol. Nephrol. 1997;29(4):393–401.
- Valente A, Sorrentino L, La Torre G, et al. Post-transfusional variation in urinary oxygen tension in surgical patients. Clin. Exp. Pharmacol. Physiol. 2008;35(9):1109–1112.
- Lankadeva YR, Okazaki N, Evans RG, et al. Renal medullary hypoxia: a new therapeutic target for septic acute kidney injury? Semin. Nephrol. 2019;39(6):543–553.
- Lankadeva YR, et al. Effects of fluid bolus therapy on renal perfusion, oxygenation, and function in early experimental septic kidney injury. Crit. Care Med. 2019;47(1):E36–E43.
- Lankadeva YR, Kosaka J, Evans RG, et al. Urinary oxygenation as a surrogate measure of medullary oxygenation during angiotensin II therapy in septic acute kidney injury. Crit. Care Med. 2018;46(1):e41–e48.
- Pei G, et al. Renal involvement and early prognosis in patients with COVID-19 pneumonia. J. Am. Soc. Nephrol. 2020;31(6):1157–1165.