Abstract
The aim of this study was to assess the capability of bacterial isolates immobilized on poly(ethylene oxide) (PEO) cryogels to degrade and utilize phenol as a sole source of carbon and energy. Two xenobiotic-degrading bacteria were isolated from industrial areas polluted with heavy metals and aromatics. Sequencing of their 16S rDNA classified them as Pseudomonas rhodesiae (denoted as KCM R5) and Bacillus subtilis (denoted as KCM RG5). The following operation parameters were used: sequencing batch process, 24 h cycle of feeding, increasing phenol concentrations from 300 to 1000 mg·L−1, volume of inflow – 250 mL, volume of outflow – from 212 to 7 mL and temperature of 28 °C. The PEO-KCM R5 biofilter was found to remove phenol at a concentration of 1000 mg·L−1, while the PEO-KCM RG5 system was unable to degrade phenol at a concentration of about 600 mg·L−1. After four weeks of biodegradation, the PEO biofilms remained compact, porous and elastic, while containing compact microbial biofilm as shown by scanning electron microscopy analysis of the cryogels. Taken together, our results demonstrate that our novel bacterial entrapment system in PEO cryogels is highly effective and sustainable for phenol degradation and can be relevant for application in the detoxification technologies of industrially polluted waters.
Introduction
Phenol and its numerous derivatives such as оrtho-nitrophenol (о-NP), 2,4-dinitrophenol (2,4-dNP), 2,5-dinitrophenol (2,5-dNP), penthachlorophenol (PCP) and 2,4-dichlorophenoxyacetic acid (2,4-D) are widely exploited in the chemical industry, agriculture and wood processing. They are highly toxic, mutagenic and/or teratogenic and some of them have been reported as potential carcinogens. All studied xenobiotics are listed in the European Community (EC) and the USA Priority List Pollutants. Phenol is also a major raw material in the chemical industry and a byproduct from benzene processing.[Citation1] It is released in the environment by the chemical-, wood-, textile- and oil-processing industry. The world production reaches seven millions of tonnes per year.[Citation2] Many bacteria belonging to genus Pseudomonas,[Citation2,Citation3,Citation4] Acinetobacter,[Citation5] Alcaligenes,[Citation6,Citation7] Bacillus,[Citation8] Sphyngomonas,[Citation9] Geobacter [Citation10] as well as some fungal species that belong to Aspergillus,[Citation11] Trichosporon [Citation12,Citation13] or Candida [Citation14] are known to degrade phenol. A limited number of studies have been reported for phenol degradation by immobilized bacteria. These include reports on the immobilization of microbes on polymers such as polyacrylamide,[Citation15] polyurethane,[Citation16] polyamide,[Citation17] polyacrylonitrile [Citation18,Citation19] or polyvinyl alcohol [Citation20]. Recent investigations demonstrated that the most appropriate materials for microbial immobilization especially for biotechnological applications are synthetic polymers.[Citation21,Citation22]
Immobilized bacteria possess some advantages to the free-swimming or planktonic cells. They harbour higher genetic capability due to the increased plasmid transfer in the microbial biofilms produced during the cell immobilization.[Citation23] Also, immobilization of the cells is biotechnologically easier because of facilitated process control.
Among the synthetic polymers, poly(ethylene oxide) (PEO) hydrogels are excellent candidates for bacterial immobilization because they are nontoxic, biocompatible materials and meet all of the requirements of strength, absorbance, flexibility and adhesiveness.[Citation24,Citation25] PEO hydrogels were first obtained in situ by γ-irradiation of dilute aqueous solutions [Citation26] and later via methods based on chemical cross-linking.[Citation27] Hydrogels of high molecular weight (MW) PEO are also easy to synthesize in situ by ultraviolet (UV) cross-linking of PEO in aqueous solution. Moreover, when UV cross-linking is carried out in a frozen aqueous system, macroporous hydrogels (cryogels) with a very high yield of gel fraction (GF) and high cross-linking density can be obtained.[Citation28,Citation29]
In this report, we describe the entrapping of two isolated xenobiotic-degrading bacteria in novel macroporous PEO cryogels and show the high performance of this new immobilization system for phenol degradation.
Materials and methods
Bacteria isolation and genotype identification
Two bacterial isolates were obtained from polluted soil in the industrial area near the Pb–Zn smelter called KCM, about 6 km from the city of Plovdiv, and from the pesticide-producing factory AGRIA in Bulgaria. The Pb–Zn smelter is the largest processing metallurgical enterprise on the Balkan Peninsula. The AGRIA factory manufactures more than 50 different pesticides.
Bacterial isolates were identified by sequencing of their 16S rDNA as described by Selenska-Pobell et al.[Citation30] Polymerase chain reaction (PCR) amplification was performed by using the primers 8F and 1513R, resulting in almost full length gene amplification. The PCR reactions were performed under the following conditions: one cycle of initial denaturation at 95 °C for 3 min, followed by 35 cycles of denaturation at 94 °C for 90 s, primer annealing at 55 °C for 40 s and primer extension at 72 °C for 1.5 min, finishing with a final extension step at 72 °C for 20 min. Restriction analysis was performed by using RsaI endonuclease (Promega, USA) and sequencing in an automated sequencer (ABI-PRISM® 310 Genetic Analyzer, PE Applied Biosystems, Forster City, CA, USA). The sequences obtained were analysed using the basic local alignment search tool (BLAST) program (NCBI). The 16S rDNA sequences were submitted to the Genbank-EMBL (European Molecular Biology Laboratory) under accession number AJ 830707 (KCM-R5) and AJ830709 (KCМ-RG5). Both strains are deposited in the Bulgarian National Bank for Industrial Microorganisms and Cell Cultures (NBIMCC): accession number NBIMCC 8726 (for KCМ-RG5) and NBIMCC 8727 (for strain KCМ-R5).
Cultivation procedures and xenobiotic tolerance of the strains
Bacteria were cultivated at 28 °C in a mineral medium prepared according to Furukawa and Chakrabarty [Citation31]** and containing: 5.6 g·L−1 of K2HPO4·3H2O, 3.4 g·L−1 of KH2PO4, 2 g·L−1 of (NH4)2SO4, 0.34 g·L−1 of MgCl2·6H2O, 0.001 g·L−1 of MnCl2·4H2O, 0.0006 g·L−1 of FeSO4×7H2O, 0.026 g·L−1 of CaCl2×2H2O and 0.002 g·L−1 of Na2MoO4 × 2H2O.
The heavy metal tolerance of the two strains was tested by adding to the medium either 20 mmol·L−1 МnSO4, 4 mmol·L−1 Pb(NO3)2, 1 mmol·L−1 CuSO4, 1 mmol·L−1 ZnSO4 or 0.5 mmol·L−1 CdCl2. 2,4-D was used to test for pesticide tolerance and was added at a concentration of 1 mmol·L−1. Growth of the bacteria in the presence or absence of these xenobiotics was scored at optical density (OD) 450 nm after cultivation for 48 h at 30 °C in liquid mineral medium. The mineral medium was prepared according the ISO/DIS 10712.2 standard and contained 1 g·L−1 NaNO3, 0.24 g·L−1 K2HPO4, 0.12 g·L−1 KH2PO4, 0.1 g·L−1 yeast extract, 10 g·L−1 glucose, 0.4 g·L−1 MgSO4 and 0.0001 g·L−1 Fe citrate.
Growth of KCM R5 and KCM RG5 on phenol and its derivatives
To examine the ability of the bacterial cultures KCM-R5 and KCM-RG5 to utilize phenol, they were grown for 144 h on phenol or its derivatives as a sole carbon and energy source. The cultivation was performed in Erlenmeyer flasks containing 500 mL of sterile synthetic mineral media. K2HPO4·3H2O and CaCl2×2H2O were added after dissolving the other components in order to avoid precipitation. The medium was autoclaved according to a standard laboratory protocol. Phenol and its derivatives were added to the medium as a sterile filtered solution at the following concentrations: phenol at 100 mg·L−1; о-NP, 2,4-dNP, 2,5-dNP, PCP or 2,4-D at 20 mg·L−1.
Cryogel preparation
High molecular weight PEO (Union Carbide; MW = 2 × 106 g mol−1) was dissolved in distilled water (5 wt.%). The photo-initiator, (4-benzoylbenzyl) trimethylammonium chloride (Aldrich; 2 wt.% with respect to the polymer), was added under stirring at room temperature, resulting in a homogeneous solution that was poured into Teflon dishes (50 mm in diameter) forming a 2.5 mm thick layer. These dishes were then kept frozen at −20 °C for 2 h. After being quickly placed in a thermostatic open chamber connected with a ‘Julabo’ cryostat apparatus, the dishes were irradiated with full spectrum UV–VIS light at −20 °C with a Dymax 5000-EC UV curing equipment with a 400 W metal halide flood lamp for 2 min (dose of 11.4 J cm−2; input power of 93 mW cm−2).
The PEO cryogels were extracted in distilled water for seven days, quickly frozen in liquid nitrogen, and freeze dried in an Alpha 1-2 freeze dryer (Martin Christ, Osterode am Harz, Germany) at −55 °C and 0.02 mbar for 24 h. The GF yield was calculated by the following equation: GF yield [%] = (weight of dried sample/initial weight of polymer) × 100.
Entrapment of bacteria into PEO cryogels
The dried PEO cryogels were swelled in 100 mL synthetic mineral medium for 24 h, without shaking. The two bacterial isolates, KCM R5 and KCM RG5, were prepared for entrapment in the PEO cryogels as follows: for the three replicates of each cryogel, a total volume of 600 mL mineral medium containing 0.1% sterile glucose and 100 mg·L−1 phenol was inoculated with 20 mL of bacterial suspension. The strains were cultivated in 1 L Erlenmayer flasks at 28 °C and 180 r·min−1 for 72 h to reach OD of 0.550. Bacterial cultures were transferred in sterile vessels in which the pre-swollen PEO cryogels were introduced and this procedure was performed three times for three independent replicates. The vessels which fitted well to the oval shape of the cryogels were tightly locked under sterile conditions and shaken mildly at 100 r·min−1 for 48 h. The immobilization of the bacterial cells in PEO cryogels could take place during this time of incubation. The resulting PEO-KCM R5 and PEO-KCM RG5 cryogels with the entrapped bacteria were taken out of the vessels and gently placed on the biofilter system of sterile reusable bottle-Top Filter 45 mm, 500 mL (Nalgene, Rochester, USA). The plain biofilter usable area was 141 mm. The locking rings were softly screwed up in order to avoid cutting of the cryogels. The operation parameters were as follows: sequencing batch process, 24 h cycle of feeding, increasing phenol concentrations from 300 to 1000 mg·L−1, volume of inflow – 250 mL, volume of outflow – from 212 to 7 mL and temperature of 28 °C. Every 24 h, 250 mL of sterile medium that contained a higher phenol concentration was poured in the upper funnel of the biofilters. No pressure was applied to the liquid and it was allowed to run through the PEO-bacterial biofilters only by the force of gravity. The PEO biofilms were then examined for phenol biodegradation for 28 days, using sterile mineral medium and the following scheme: 7 days with 300 mg·L−1, 5 days with 400 mg·L−1, 4 days with 600 mg·L−1 and 12 days with 1000 mg·L−1. Both biofilters, PEO-KCM R5 and PEO-KCM RG5, were prepared in triplicates.
Phenol biodegradation
After construction, the PEO-KCM R5 and PEO-KCM RG5 biofilters were washed for 24 h with 250 mL of phenol-free synthetic mineral medium to remove the residual compounds. The phenol amount in the filtered liquid (outflow) was measured every 24 h. The phenol concentration in the inflow and in the outflow was colorimetrically determined following the pyramidone (aminophenazone) based method.[Citation31] Briefly, 0.125 mL of filtered liquid, 0.250 mL of ammonium chloride buffer (pH 9.3; adjusted with ammonium), 0.125 mL of 3.5% pyramidone and 0.375 mL of ammonium persulfate (pH 7.0; adjusted with ammonium) were added into 12.375 mL of distilled water. After mixing, the reaction was kept at room temperature for 45 min. The absorption was measured spectrophotometrically (Spekol-11, Carl Zeiss Jena, Germany) at 540 nm against a control in which 0.125 mL of distilled water was added instead of biofilter filtrate. The phenol amount was calculated according to a standard curve. The phenol biodegradation by the constructed biofilters was conducted at 28 °C.
Scanning electron microscopy (SEM)
Phenol treated biofilms were investigated by Jeol Scanning Electron Microscope JSM-5510 (Jeol Ltd., Tokio, Japan), 10 kV at magnification ×500, ×1500, ×2500, ×5000, ×10,000 and ×20,000.
Results and discussion
The focus of our study was the construction of the biofilters and evaluation of their capability for phenol biodegradation by using two different bacterial strains isolated from heavily polluted soil near a Pb–Zn smelter enterprise in South Bulgaria.
Phenotype and genotype characterization of isolated bacteria
Analysis of 16S rDNA gene of the two isolates revealed that they belong to Pseudomonas and Bacillus genera, respectively. The KCM R5 isolate was determined as a Gram-negative, motile and oval-shaped bacterium, while the KCM RG5 isolate was a Gram-positive, motile and rod-shaped one. The morphology of the two isolates is shown in .[Citation32]
Genotype characterization of the two strains was performed by sequencing of the 16S rDNA gene. It was found that the 16S rDNA gene of the KCM R5 isolate shares 99.6% identity with that of Pseudomonas rhodesiae KK1 (АY043360), which belongs to Gammaproteobacteria and which was isolated from a former gas-processing supply.[Citation33] This strain is described to be able to catabolize polyaromatic hydrocarbons likely because of its high content of dioxygenases.[Citation33] Based on the genotype analysis results, we renamed our isolate P.rhodesiae KCM R5. The 16S rDNA gene sequence of the second isolate showed 99.8% identity with the gene of the xenobiotics tolerant strain Bacillus subtilis BHP6-1 (АY162131). The latter strain is a member of Firmicutes and has been isolated from soils contaminated with xenobiotics (heavy metals and hydrocarbons); it is currently used for bioremediation.[Citation33] Thus, we renamed the second isolate B.subtilis KCM RG5. These two new strains are available under No. NBIMCC 8726 (KCМ-RG5) and No. NBIMCC 8727 (KCМ-R5) in the Bulgarian NBIMCC.
Growth in glucose liquid mineral medium in the presence of the heavy metals (4 mmol·L−1 Pb(NO3)2, 1 mmol·L−1 CuSO4 or 1 mmol·L−1 ZnSO4) revealed that B. subtilis KCM-RG5 was more sensitive than P. rhodesiae KCM-R5 to the above-mentioned heavy metals. Strain KCM-R5 was able to tolerate up to 1 mmol·L−1 of pesticide 2,4-D, while KCM-RG5 could not grow in the presence of such concentrations of pesticide. shows the growth profile of P.rhodesiae KCM-R5 and B.subtilis KCM-RG5 at phenol concentrations of 100 mg·L−1 or its derivatives at 20 mg·L−1. Overall, the biomass yield on these substrates was higher for strain KCM-R5 than strain KCM-RG5. For both strains, 2,5-dNP appeared to be the preferred substrate, whereas phenol was less efficient as a carbon source. Interestingly, strain KCM-RG5, when growing on 2,4-D, showed an unexpectedly long lag phase up to 72 h. We could assume that this was due to longer time needed for the activation of oxygenase enzymes.
PEO gel fabrication and characterization
PEO cryogel of high GF yield (93%) was synthesized via UV irradiation of moderately frozen semi-diluted solution of PEO and photo-initiator for an extremely short time and subsequent thawing as described elsewhere.[Citation28,Citation29] The obtained cryogel was a heterogeneous, super-macroporous material with large interconnected pores (50 to 100 µm) surrounded by dense thin walls (ca. 1–2 µm). Importantly, more than 80% of the water in the cryogel was capillary-bound water that filled the space of the macropores. The freeze-dried PEO cryogel possesses the original macroporous structure and, once immersed in the suspension, the liquid fills the channels (interconnected pores) of the cryogel.[Citation32] The fabrication of PEO cryogels as carriers is attractive because they are not expensive, not toxic and show high biocompatibility.
Entrapment of bacteria and efficiency for xenobiotic degradation in the PEO cryogels
As indicated above, the two bacterial strains used in our study do not grow very well in the presence of phenol at 100 mg·L−1. Thus, we presumed that feeding the biofilters with an initial high phenol level (300 mg·L−1) could lead to cell-growth inhibition. Therefore, we initially allowed both bacterial biofilms in the biofilters to adapt in the presence of lower phenol concentration (100 mg·L−1) for 24 h before raising it to 300 mg·L−1. Also, we chose to fix the operational temperature at 28 °C, taking into account that this parameter is critical for xenobiotic degradation. Indeed, several reports have described the optimal temperature conditions to be 30 °C for optimal phenol degradation by Pseudomonas sp. at concentrations up to 500 mg·L−1,[3,Citation4] while other authors describe that the optimal temperature for xenobiotic degradation is within the range of 25–30 °C and that phenol degradation stops at temperatures close to 5 °C.[Citation34]
The results plotted in show that both biofilters, PEO-KCM R5 and PEO-KCM RG5, demonstrated similar rate of phenol removal at phenol concentrations of 300, 400 and 600 mg·L−1, the efficiency being near 100%. Interestingly, at 17th day, when 1000 mg·L−1 of phenol were fed into the PEO biofilters, only strain KCM R5 was able to degrade it over 24 h, while the PEO-KCM RG5 biofilter lost this capability and the phenol removal efficiency decreased rapidly. This indicates that strain KCM RG5 entrapped in the PEO cryogel was no longer able to degrade phenol at concentrations higher than 600 mg·L−1.[Citation32]
Figure 3. Efficiency of phenol biodegradation by biofilters PEO-KCM R5 and PEO-KCM RG5. Biofilms were treated for 28 days with different phenol concentrations: 300 mg·L−1 (1–7 day), 400 mg·L−1 (8–12 day), 600 mg·L−1 (12–16 day) and 1000 mg·L−1 (17–28 day).
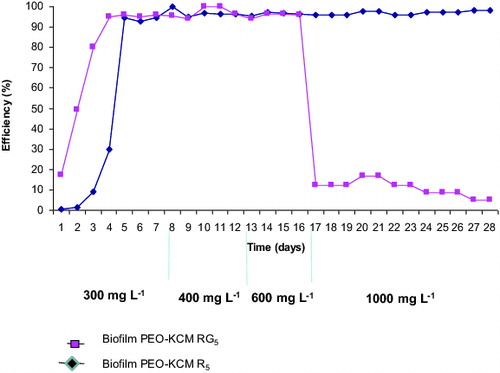
It is noteworthy that at the end of our experiments, the amounts of the filtered liquid (outflow) in both biofilters decreased up to 10 times compared to that of the filtered liquid in the initial days. A possible explanation for this reduction of the size of the filtered liquid is the intensive bacterial colonization in the PEO cryogels, which filled up the cryogel pores as shown by SEM of the biofilters imaged after phenol treatments ( and ). The macrostructure of both biofilters from the SEM micrographs showed that the PEO cryogels remained compact and elastic, with no damages even after long and aggressive bacterial development on phenol.
Figure 4. Macrostructure and SEM analysis of the PEO-KCM R5 (A) and PEO-KCM RG5 (B) biofilter treated with phenol.
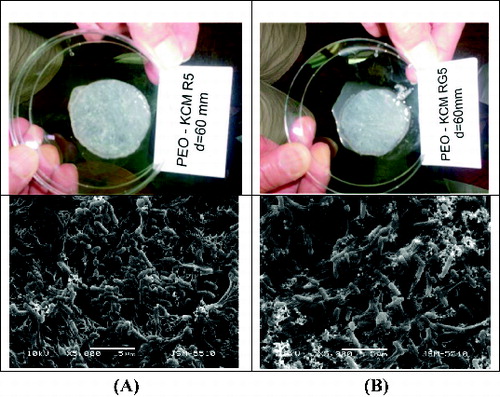
Figure 5. SEM micrography of biofilm formed on the PEO-KCM R5 biofilter. Magnification ×500 (a), ×1500 (b), ×2500 (c), ×5000 (d), ×10,000 (e) and ×20,000 (f).
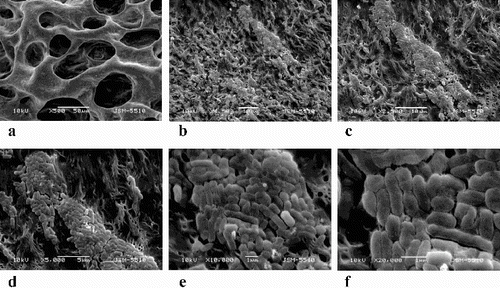
As phenol degradation is an oxidative process, it depends on oxygen availability. Our experiment was conducted in aerobic conditions simulating the degradation that may occur under natural conditions. In other words, there was no extra aeration supplied and thus, it could be assumed that the amount of oxygen dissolved in the water was enough for the process to take place. Therefore, it might be possible that additional oxygen would improve the phenol degradation.
Another focus of this work was to study the effect of increasing phenol concentrations from 300 to 1000 mg·L−1. Phenol concentrations were chosen according to the industrial phenol water contamination values which were between 500 and 700 mg·L−1. Quite remarkably, one of the studied strains, P.rhodesiae KCM R5, entrapped in our PEO cryogel was able to remove 1000 mg·L−1 phenol in 24 h, while the Bacillus strain suddenly slowed down its degradation capability at 600 mg·L−1 phenol. Since our assays were carried out at pH 6.65 and some bacilli are more effective in biodegradation at pH 5.0,[Citation34] it is possible that the pH value in our experiments might be the reason for the lower capacity of this strain to degrade phenol. The performance of our Pseudomonas strain is comparable to that previously reported also about a member of genus Pseudomonas which was able to degrade 1 g·L−1 phenol in liquid culture [Citation36] and with that shown by a strain of Alcaligenes faecalis that was found to grow on 1.6 g·L−1 of phenol.[Citation7]
As reported in this paper, the immobilization of bacteria increases their capability for phenol biodegradation. This is likely due to the biofilm formation shown by SEM. The size of the PEO-cryogels pores, which is between 50 and 100 µm, fits well the bacterial cell size, allowing easier entrapment of cells in the inner channels. When entrapped in the PEO cryogel, the bacterial cells can divide easily likely due to the high amount of capillary-bounded water inside the cryogel, which amounts to about 80% of the total water content, leading to vast bacterial colonization in the cryogels. The decrease in phenol-degrading capacity of the biofilter observed at the end of the experiment could most probably be considered as result of reduced permeability of the gel due to progressive bacterial colonization.
Conclusions
This study shows the phenol-degradation performance of a promising new environmentally friendly biomaterial that was constructed and investigated for its biodegradation capability. The biofilter is cheap, highly effective and could be potentially useful in industrial wastewater treatment. P.rhodesiae KCM-R5 and B.subtilis KCM-RG5 entrapped in PEO cryogel can easily degrade xenobiotics as phenol. Important factors influencing the xenobiotic biodegradation, such as optimal temperature, optimal pH, oxygen availability, rate of nutrient inflow and presence of other chemicals in the industrially polluted water, should be further investigated to achieve optimized PEO biofilters combining efficient bacterial development and high performance in xenobiotic degradation, relevant for depollution treatment.
Disclosure statement
No potential conflict of interest was reported by the authors.
Additional information
Funding
References
- Bahidsky M, Hronec M. Direct hydroxylation of benzene to phenol. Petroleum Coal. 2004;46:49–55.
- Neumann G, Tera R, Monson L, Kivisaar M, Schauer F, Heipieper HJ. Simultaneous degradation of atrazine and phenol by Pseudomonas sp. strain ADP: effect and adaptation. Appl Environ Microbiol. 2004;70:1907–1912.
- Polymenakou P, Stephanou E. Effect of temperature and additional carbon sources on phenol degradation by an indigenous soil Pseudomonad. Biodegradation. 2005;16:403–413.
- Tsai S-Y, Juang R-S. Biodegradation of phenol and sodium salicylate mixtures by suspended Pseudomonas putida CCRC 14365. J Hazard Mater. 2006;138:125–132.
- Pessione E, Bosco F, Specchia V, Giunta C. Acinetobacter radioresistens metabolizing aromatic compounds. Optimization of the operative conditions for phenol degradation. Microbios. 1996;88:213–221.
- Hudges E, Bayly R, Scurray R. Evidence for isofunctional enzymes in the degradation of phenol, m- and p-toluate, and p-cresol at catechol meta-cleavage pathways in Alcaligenes eutrophus. J Bacteriol. 1984;158:79–83.
- Jiang Y, Wen J, Bai J, Jia X, Hu Z. Biodegradation of phenol at high initial concentration by Alcaligenes faecalis. J Hazard Mater. 2007;147:672–676.
- Tallur P, Megadi V, Kamanavalli C, Ninnekar H. Biodegradation of p-Cresol by Bacillus sp. Strain PHN 1. Curr Microbiol. 2006;53:529–533.
- Liu Y, Nikolausz M, Wang X. Biodegradation and detoxification of phenol using free and immobilized cells of Acinetobacter sp. XA05 and Sphyngomonas sp. FG03. J Environ Sci Health A. 2009;44:130–136.
- Kunapuli U, Jahn MK, Lueders T, Geyer R, Heipieper HJ, Meckenstock RU. Desulfitobacterium aromaticivorans sp. nov. and Geobacter toluenoxydans sp. nov., iron-reducing bacteria capable of anaerobic degradation of monoaromatic hydrocarbons. Int J Syst Evol Microbiol. 2010;60:686–695.
- Jones K, Trudgill P, Hopper D. Evidence of two pathways for the metabolism of phenol by Aspergillus fumigatus. Arch Microbiol. 1995;163:176–181.
- Santos V, Linardi V. Phenol degradation by yeasts isolated from industrial effluent. J Gen Appl Microbiol. 2001;47:213–221.
- Chtourou M, Ammar E, Nasri M, Medhioub K. Isolation of a yeast Trichosporon cutaneum, able to use low molecular weight phenolic compounds: application to olive mill waste water treatment. J Chem Technol Biotechnol. 2004;79:869–878.
- Yan J, Jianping W, Hongmei L, Suliang Y, Zongding H. The biodegradation of phenol at high initial concentration by the yeast Candida tropicalis. Biochem Eng J. 2005;24:243–247.
- Lusta K, Starostina N, Gorkina N, Fikhte B, Lozinski V. Immobilization of E. coli cells in macroporous polyacrylamide cryogels. Appl Biochem Microbiol. 1988;24:418–426.
- Stormo K, Crawford R. Preparation of encapsulated microbial cells for environmental applications. Appl Environ Microbiol. 1992;58:727–730.
- Godjevargova T, Aleksieva Z, Ivanova D, Shivarova N. Biodegradation of phenol by Trichosporon cutaneum cells covalently bound to polyamide granules. Process Biochem. 1998;33:831–835.
- Godjevargova T, Ivanova D, Aleksieva Z, Dimova N. Biodegradation of toxic organic components from industrial phenol production waste waters by free and immobilized Trichosporon cutaneum R57. Process Biochemistry. 2003;38:915–920.
- Yordanova G, Ivanova D, Godjevargova T, Krastanov A. Biodegradation of phenol by immobilized Aspergillus awamori NRRL 3112 on modified polyacrylonitrile membrane. Biodegradation. 2009;20:717–726.
- El-Naas MH, Al-Muhtaseb SA, Makhlouf S. Biodegradation of phenol by Pseudomonas putida immobilized in polyvinyl alcohol (PVA) gel. J Hazard Mater. 2009;164:720–725.
- Lozinski VI. Galaev IY, Plieva FM, Savina IN, Jungvid H, Mattiasson B. Review: polymeric cryogels as promising materials of biotechnological interest. Trends Biotechnol. 2003;21:445–451.
- Plieva F, Galaev I, Noppe W, Mattiasson B. Cryogel applications in microbiology. Trends Microbiol. 2008;16:543–551.
- Lappin-Scott H, Costerton J. Microbial biofilms. Cambridge (England): Cambridge University Press; 2003. p. 15.
- Peppas N, Mikos A. Preparation methods and structure of hydrogels. Boca Raton, FL: CRC Press; 1987. p. 24.
- Loh K, Chung T, Ang W. Immobilized-cell membrane bioreactor for high-strength phenol wastewater. J Environ Eng. 2000;126:75–79.
- King P, Warwick N. United States patent US 3,264,202. 1966 Aug.
- Gnanou J, Hild G, Rempp P. Hydrophilic polyurethane networks based on poly(ethylene oxide): synthesis, characterization, and properties. Potential applications as biomaterials. Macromolecules. 1984;17:945–952.
- Doycheva M, Petrova E, Stamenova R, Tsvetanov C, Riess G. UV-induced cross-linking of poly(ethylene oxide) in aqueous solution. Macromol Mater Eng. 2004;289:676–680.
- Petrov P, Petrova E, Stamenova R, Tsvetanov CB, Reiss G. Cryogels of cellulose derivatives prepared via UV irradiation of moderately frozen systems. Polymer. 2006;47:6481–6484.
- Selenska-Pobell S, Kampf G, Flemming K, Radeva G, Satchanska G. Bacterial diversity in soil samples from two Uranium waste piles as determined by rep-APD, RISA and 16S rDNA retrieval. Antonie van Leewenhoek. 2001;79:146–161.
- Furukawa R, Chakrabarty S. Common induction and regulation of biphenyl, xylene, toluene and salycilate in Pseudomonas paucimobilis. J Bacteriol. 1983;154:1356–1363.
- Satchanska G, Topalova Y, Dimkov R, Petrov P, Tsvetanov C, Selenska-Pobell S, Gorbovska A, Bogdanov V, Golovinsky E. Phenol biodegradation by two xenobiotics tolerant bacteria immobilized in polyethyleneoxide cryogels. Compt Rend Acad Bulg Sci. 2009;62:957–963.
- Kahng H, Nam K, Kukor J, Yoon B, Lee D, Oh D, Kam S, Oh K. PAH utilization by Pseudomonas rhodesiae KK1 isolated from a former manufactured-gas plant site. Appl Microbiol Biotechnol. 2002;60:475–480.
- Bodour AA, Drees KP, Maier RM. Distribution of biosurfactant-producing bacteria in undisturbed and contaminated arid southwestern soils. Appl Environ Microbiol. 2003;69:3280–3287.
- Kvasnikov E, Klushnikova T. Microorganisms – oil destructors in water basins. Kiev: Naukova dumka; 1981. p. 76.
- Hinteregger C, Leitner R, Loidl M, Ferschl A, Streichsbier F. Degradation of phenol and phenolic compounds by Pseudomonas putida EKII. Appl Microbiol Biotechnol [Internet]. 1992 (cited 2014 Oct 7);37:252–259. Available from: http://www.embl.org/