ABSTRACT
This study devised an effective method for the direct production of hydroxyfatty acids (HFAs) from free fatty acids (FFAs) using Escherichia coli. By employing fatty acid hydroxylase P450BM3 and glucose dehydrogenase (GDH) to convert FFAs to HFAs, high-specificity production of C12 HFAs was achieved. By further knocking out the endogenous fadD gene of E. coli, an engineered strain capable of producing 702.3 mg/L C12 HFA was finally obtained, accounting for 92.9% of the total HFAs production, representing a higher titre reported in shake flask so far. This study provides an attractive strategy for increasing HFAs production in E. coli by increasing NADPH accumulation.
Introduction
Long-chain hydroxyfatty acids (HFAs), with hydrocarbon chains ranging from C12 to C18, are well known to have special properties such as precursors for pseudoceramides, polyesters and lactones.[Citation1–3] Traditionally, HFAs can be prepared through metathesis or using transition metal as the catalyst, but harsh reaction conditions and low region-selectivity become the limitation of the catalytic reaction.[Citation4–6] In order to cope with the increasing energy costs and environmental concerns, there is much interest in developing technologies that could convert renewable biomass. Many studies have proposed the production of HFAs by microorganisms and the reports suggested that Bacillus,[Citation7] yeast,[Citation8] and Pseudomonas [Citation9] are able to produce HFAs from FFAs. However, HFAs production has been hampered by the slow growth rates and complicated regulation mechanisms of these organisms.
As an effort to solve problems mentioned above, Escherichia coli has been developed as an alternative host and hydroxylation as an alternative technology.[Citation10] Liu et al. [Citation11] showed that E. coli with hydroxylase P450BM3 could convert dodecanoic acid into ω-HFAs, but the highest conversion rate was only 59.7%. Another study showed that the engineered E. coli with acyl-CoA synthase (fadD) deletion could increase hydroxypalmitic acid productivity. However, this method needs a long reaction time (36 h) and the fed-batch reaction in the process limits its further industrial application.[Citation12] Wang et al. [Citation13] reported an engineered E. coli could produce HFAs directly from glucose, but the production was low (117.0 mg/L). All these drawbacks led people to search for more effective biosynthesis pathway that may contribute to a further improvement of HFAs production.
Theoretically, to reach the goal of converting FFA to HFA more effectively by metabolically engineered E. coli catalysts, three approaches are required: first, convert FFAs to HFAs with an exogenous effective fatty acid hydroxylase; second, improve the level of hydroxylation by using NADPH in vivo; third, knock out the endogenous fadD gene of the E. coli to block the FFAs recycling and degradation.
For the first step, the cytochrome P450 family members, P450BM3 from Bacillus megaterium and YetO from Bacillus subtilis, have strong ability of efficiently hydroxylating the FFAs for the synthesis of HFAs,[Citation14,Citation15] which are capable of breaking C–H bonds and attach a hydroxyl group to the carbon and hydroxylating the FFAs at the ω-(1-3) position, as HFAs with short branched chains possess better application than counterparts in the polymer industry.[Citation16,Citation17] To employ the better-performing one of the two P450s, it is necessary to compare their efficacies on the hydroxylation of FFAs in E. coli host. On the other hand, to convert E. coli into an effective producer of HFAs, E. coli required a ready supply of NADPH as a reductant in vivo.[Citation18,Citation19] In-situ NADPH regeneration was efficient and cost-effective, and several enzymes have been cloned and expressed in hosts.[Citation20–22] Among them, glucose dehydrogenase (GDH) and malic enzyme (ME) are preferred for the enzyme-coupled systems to recycle NAD+/NADP+, by this feature, the E. coli bio-oxidation system has been used in indigo,[Citation23] α-pinene,[Citation24] and fatty acid [Citation25] production.
The objective of the present study is to devise an effective method to improve the production of HFAs from FFAs using E. coli. To employ the better-performing fatty acid hydroxylase, two strains were first constructed to compare their efficacies on the hydroxylation of FFAs. Then two derivative strains were constructed, which contained hydroxylase and different NADPH regeneration enzyme, respectively, to enhance the production of HFAs. Furthermore, the E. coli endogenous fadD gene was knocked out to inhibit fatty acid β-oxidation pathway. This work represents the potentiality to reduce the process cost and enhance the production in the scale-up biosynthesis of HFAs from renewable biomass.
Materials and methods
Plasmid construction
The ME gene from E. coli K12, P450BM3 and GDH genes from B. megaterium ATCC 14851 and yetO gene from B. subtilis ATCC23857 were obtained by polymerase chain reaction (PCR) using Pyrobest DNA polymerase (TaKaRa, Dalian, China) with the primer set ME-F/ME-R, P450BM3-F/P450BM3-R, GDH-F/GDH-R and yetO-F/yetO-R, respectively. Custom oligonucleotides (primers) for all PCR amplifications are shown in
Table 1. Bacterial strains, plasmids and primer used in this study.
The amplified yetO gene was first gel purified, then digested with BgIII and XhoI and finally ligated into pACYCDuet-1 (Novagen, Darmstadt, Germany) that had been digested with the same restriction enzymes, yielding pW1. In the same means, the NcoI-EcoRI-digested P450BM3 were ligated into pACYCDuet-1 to yield pW2. For overexpression of GDH and malic enzyme (ME), the digested ME gene and digested gene GDH were ligated into corresponding sites of pET-28a (+) and pET-30a (+) (Novagen, Darmstadt, Germany) to create pW3 and pW4, respectively. All plasmids used in this work are listed in
Strains, medium and culture conditions
The E. coli DH5α (TaKaRa, Dalian, China) was used as the host for plasmid propagation, and the E. coli BL21 (DE3) (TaKaRa, Dalian, China) was used as the host to overproduce proteins. The fadD gene of E. coli BL21 (DE3) was knocked out using TargeTron Gene Knockout System (Sigma-Aldrich) according to the manufacturer's instructions. All E. coli strains used in this study are listed in
During strain construction, shaking flask culture cultivations were performed at 37 °C in LB medium (10 g/L tryptone, 10 g/L NaCl, 5 g/L yeast extract). For initial protein production in shake flasks, strains were grown in M9 medium (15.13 g/L Na2HPO4•12H2O, 3 g/L KH2PO4, 1 g/L NH4Cl, 0.5 g/L NaCl, 2 mM MgSO4) containing 20 g/L glucose, 50 μg ml−1 of kanamycin and 34 μg mL−1 chloramphenicol were added to the medium if necessary. The cultures were initially inoculated at 37 °C .When the OD600 reached 0.6∼0.8, protein expression was induced by adding 0.1 mmol//L isopropylthiogalactoside (IPTG) and the cultures were switched to incubate at 30 °C.
Oxidation of FFAs by resting cells of recombinants
For bioconversion studies with resting cells, when the cells grew to late-exponential phase in M9 medium, they were harvested and resuspended with a density of 2 g/L cell dry weight (CDW) in 0.2 mol//L sodium phosphate buffer (pH 7.4), containing 0.5% (wt/v) glucose as the carbon source. The cultures were incubated after the addition of the FFAs (dissolved in dimethyl sulfoxide). To avoid the HFAs to be further oxidized to multiple chemicals, e.g. ketones, the bioconversions were performed under oxygen-unlimited conditions. Unless otherwise stated, reactions were incubated for 12 h at 30 °C, 180 rpm.
GC/MS analysis of the fatty acid metabolites
Prior to GC/MS analysis, the cultures were centrifuged and separated into cell pellet and supernatant fraction; the supernatant was acidified by 6 mol/L HCl (pH2) and extracted with equal volume of chloroform. The derivatized chloroform was evaporated to clear the solvent with nitrogen, and the methyl esters were generated by adding sulphuric acid/methanol (1:4, by vol) at 60 °C for 30 min. The GC analysis was performed on Varian 450GC equipped with a HP-5 column (30 m × 0.32 mm × 0.25 μm) fitted with a flame ionization detector. The temperature programme was 80 °C for 2 min, ramping at 5 °C per minute to 240 °C, followed by heating until 240 °C with 10 min. 10-Hydroxydecanoic acid was added to the assay mixture before acidification of the sample to serve as an internal standard.
For GC-MS analysis to identify the oxidation products’ structures, the products were derivatized and the GC column and separation conditions were the same as those for GC analysis mentioned above. Electron impact mass spectra were obtained with an Agilent Trace GC 5975C system coupled with a quadrupole detector (ion source temperature, 220 °C; EI ionization, 70 eV). Quantifiable product data were calculated from GC percentages relative to internal standard.
Results and discussion
Comparison of the efficiencies of different fatty acid hydroxylases
Fatty acids serve as reaction substrates for HFA production; comparison between the hydroxylases catalytic efficiencies for the biosynthesis of HFA in E. coli is needed. The resultant engineered strains were designated as WX1 and WX2, respectively. On the basis of previous reports,[Citation13] the FFAs with carbon chain lengths over C16 cannot be efficiently converted by the engineered E. coli. Therefore, palmitic acid (C16:0), stearic acid (C18:0) and oleic acid (C18:1) were excluded as the candidate fatty acid substrates. An equivalent blend of fatty acids (0.5 mol//L), lauratic acid (C12:0) and myristic acid (C14:0) were added into the cultures to test substrate specificities and conversion efficiencies. A significant difference in HFAs production was observed (); compared with the WX1, the WX2 showed higher HFAs production and conversion efficiency, and no HFAs was detected in the control strain carrying an empty vector.
Figure 1. Total HFA production and theoretical yield by engineered E. coli strains WX1 (employing yetO) and WX2 (employing P450BM3). Both the WX1 and WX2 cultures were supplemented with 0.5 mmol/L C12 and C14 FFAs. WX2 produced more HFAs than WX1. The hydroxydodecanoic acid (C12) was the major HFAs in both cultures of WX1 and WX2. The error bars represent the range from two independent experiments.
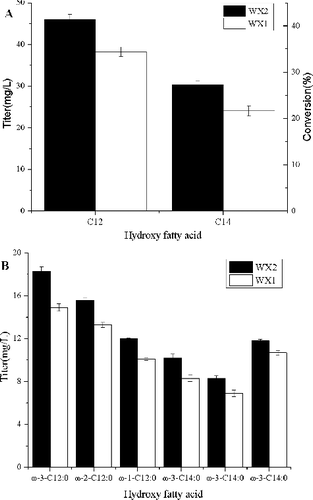
In addition, WX2 produced 45.9 mg/L hydroxydodecanoic acid (C12) with 42.5% conversion. WX1 obtained 30.3 mg/L hydroxydodecanoic acid (C12) with 28.3% conversion. WX2 synthesized 1.5-fold more hydroxydodecanoic acid than WX1. Moreover, the production and conversion of WX1 and WX2 dramatically decreased when myristic acid (C14) was used as substrate ((a)). These results demonstrated the hydroxylases, WX1 and WX2 employed, have a substrate preference towards C12 fatty acid, so large C12 HFAs were produced than C14 HFAs. Likewise, because P450BM3 exhibited higher catalytic activity to C12 and C14, WX2 produced more HFAs with higher FFAs conversion efficiencies than WX1. Based on the data, the P450BM3 was fixed as the fatty acid hydroxylase for bioconversion of FFAs to HFAs.
The chemical compositions of HFAs produced by WX1 and WX2 were analyzed and ω-(1-3)-hydroxydodecanoic acid and ω-(1-3)-hydroxytetradecanoic acid were their major HFA constituents, respectively ((b)). This result was consistent with previous reports.[Citation13] In addition, the separation of FFAs from microorganism cells by conventional methods was found both energy- and cost-intensive,[Citation11,Citation26] but in this study, the hydroxylated FFAs were excreted to the culture supernatant and not in the cells. In order to skip FFAs separation processes, the mechanism of HFAs secretion needs further research.
Comparison of the efficiencies of different enzymes of NADPH regeneration
Considering the P450BM3 had a higher catalytic activity to lauratic acid (C12:0) than myristic acid (C14:0), when expressed in E. coli, the lauratic acid was fixed as the substrate for bioconversion. To test if NADPH regenerations are contributed to the reaction rates and achieve better yields, malic enzyme (ME) and GDH were overexpressed in E. coli. The resultant two engineered strains were WX3 and WX4. WX3 (harbouring P450BM3 and ME gene) bioconversion was supplied with 0.5 mmol/L lauratic acid. However, HFAs production did not significantly change with in vivo expressed malic enzyme (data not shown), which might be due to the lack of malate, serving as a substrate for NADPH synthesis, when expressed in E. coli.[Citation27] Therefore, in order for WX3 to convert FFAs to HFAs, it is first needed to add enough malate, serving as a substrate for NADPH synthesis.
Having obtained a large amount of HFAs, P450BM3 alone is unable to provide efficiencies towards FFAs for HFAs production in E. coli. When using recombinant WX3 and WX4 as biocatalysts, WX4 produced 101.3 mg/L HFAs; the hydroxylation efficiencies towards the lauric acid range from 42.5% to 93.8% at the same concentration, so a steep increase of product formation was observed ((a)). Malate was added to the culture to improve the availability of substrate for malic enzyme during WX3 cultivation.[Citation25] However, the strains WX3 achieved an HFA titre of 49.6 mg/L with only 45.9% theoretical yields ((a)). WX3 synthesized 1.3-fold more hydroxydodecanoic acid than WX2, while XW4 produced 3.3-fold more hydroxydodecanoic acid than WX2. The reason for this could be toxicity of malate to E. coli or its inhibition caused from other related enzymes.[Citation25]
Figure 2. Total HFA production and theoretical yield by engineered E. coli strains WX3 (employing P450BM3 and ME) and WX4 (employing P450BM3 and GDH). Both the WX3 and WX4 cultures were supplemented with 0.5 mmol/L C12 FFA. WX4 produced more HFAs than WX3. The error bars represent the range from two independent experiments.
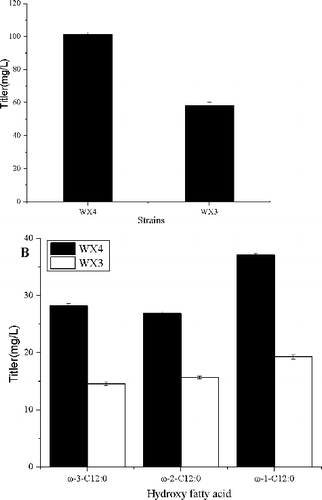
The results showed that the conversion of FFAs to HFAs could be efficiently completed with NADPH regeneration, catalyzed by GDH gene. Considering the production assays, the results would support the hypothesis that the NADPH concentration was a limiting factor in the fatty acid hydroxylation reaction. The recombinant E. coli expressing GDH gene could efficiently biosynthesize 4-chloro-3-oxobutanoate, which was consistent with the previous report.[Citation28] Furthermore, the composition of HFAs did not make much change with different enzymes ((b)), suggesting NADPH regeneration in E. coli only affected the quantity but not the composition of FFAs.
Enhanced production of HFA from FFA
To further determine the production of HFAs, effects of substrate concentration on transformation of lauric acid were investigated ((a)). WX4 was cultivated in M9 medium with different concentrations of lauric acid in the range of 0.5—5 mmol/L. The amount of HFAs increased with increasing substrate concentration, reached a maximum production (510.8 mg/L with 94.6% yield) at the concentration of 2.5 mmol/L, whereas the conversion did not change significantly in the range between 0.5 and 2.5 mmol/L. However, HFAs production and conversion tended to decrease with increasing concentration of lauric acid in the range between 3.5 and 5 mmol/L. When cultivation was carried out with 3.5 mmol/L lauric acid, 321.3 mg/L HFAs were produced with the conversion only 42.5%. This is probably because high concentration of substrate caused the inactivation of the enzyme or has toxic effects on E. coli.
Figure 3. Total HFA production and theoretical yield by engineered E. coli strains WX4 and WX5. (a) The HFA production and theoretical yield of WX4 (employing P450BM3 and GDH) with different concentration lauric acid. (b) The HFA production and theoretical yield of fadD deletion on product synthesis. The error bars represent the range from two independent experiments.
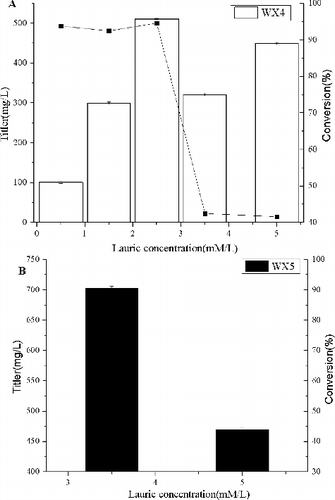
Because the FFAs could not be recycled and degraded in the fadD-deleted strain,[Citation10] the next step for further improving the productivity of the WX4 was investigated. The fadD gene of WX4 was knocked out, yielding WX5. When WX5 convert 3.5 mmol/L lauric acid into HFAs, the yield was increased up to 702.3 mg/L with the conversion of 92.9%, a 2.2-fold increase in titre compared to the strain WX4 at the same concentration (). This result indicates that a fadD deletion mutant successfully increased the yield of ω-hydroxylation. With increasing substrate concentration to 5 mmol/L, HFAs production decreased to 469.8 mg/L with the conversion of only 43.5%. The poor HFA production of WX5 may be attributed to low adenosine triphosphate (ATP), or membrane lipid during hydroxylation in E. coli.[Citation12] The chemical compositions of HFAs produced by WX5 did not make much change. The results are not surprising, because the deletion of fadD only enhanced the quantity, but not the composition of FFAs.[Citation13]
Conclusion
The goal of this study was to develop an E. coli strain for FFAs’ effective hydroxylation. In the present study, we demonstrated that engineered E. coli coupled with NADPH regeneration system can be used for the improvement of value-added HFAs production. The final genetic strain, WX5, accumulated HFAs up to 702.3 mg/L and the conversion in shake flasks was 92.9%. In addition, in this study, just C12 and C14 HFAs were obtained, by the production of the hydroxylases or finding new hydroxylases with different substrate preference with the engineering strategy described above. That could have great potential to expand the HFAs production with more chain lengths.
Disclosure statement
The authors declare no conflict of interest.
Additional information
Funding
References
- Hou C, Forman RJ. Growth inhibition of plant pathogenic fungi by hydroxy fatty acids. J Ind Microbiol Biotechn. 2000;24:275–276.
- Shin SY, Kim HR, Kang SC. Antibacterial activity of various hydroxy fatty acids bioconverted by Pseudomonas aeruginosa PR3. Agric Chem Biotechnol. 2004;47:205–208.
- Zimmerman DC. A new product of linoleic acid oxidation by a flaxseed enzyme. Biochem Biophys Res Commun. 1966;23:398–402.
- Trzaskowski J, Quinzler D, Bährle C, et al. Aliphatic long‐chain C20 polyesters from olefin metathesis. Macromol Rapid Commun. 2011;32:1352–1356.
- Dapurkar SE, Kawanami H, Yokoyama T, et al. Catalytic oxidation of oleic acid in supercritical carbon dioxide media with molecular oxygen. Top Catal. 2009;52:707–713.
- Metzger JO. Fats and oils as renewable feedstock for chemistry. Eur J Lipid Sci Technol. 2009;111:865–876.
- Kuo TM, Nakamura LK, Lanser AC. Conversion of fatty acids by Bacillus sphaericus-like organisms. Curr Microbiol. 2002;45:265–271.
- Lu W, Ness JE, Xie W, et al. Biosynthesis of monomers for plastics from renewable oils. J Am Chem Soc. 2010;132:15451–15455.
- Wallen L, Benedict R, Jackson R. The microbiological production of 10-hydroxystearic acid from oleic acid. Arch Biochem Biophys. 1962;99:249–253.
- Steen EJ, Kang Y, Bokinsky G, et al. Microbial production of fatty-acid-derived fuels and chemicals from plant biomass. Nature. 2010;463:559–562.
- Liu Q, Liu YZ, Liu DJ, et al. Study on engineering strain of E. coli biotransformation fatty acid. J Chin Cereals Oiles Assoc. 2013;28:41–45. Chinese.
- Bae JH, Park BG, Jung E, et al. fadD deletion and fadL overexpression in Escherichia coli increase hydroxy long-chain fatty acid productivity. Appl Microbiol Biotechnol. 2014;98:8917–8925.
- Wang X, Li L, Zheng Y, et al. Biosynthesis of long chain hydroxyfatty acids from glucose by engineered Escherichia coli. Bioresour Technol. 2012;114:561–566.
- Lentz O, Urlacher V, Schmid RD. Substrate specificity of native and mutated cytochrome P450 (CYP102A3) from Bacillus subtilis. J Biotechnol. 2004;108:41–49.
- Gustafsson MC, Roitel O, Marshall KR, et al. Expression, purification, and characterization of Bacillus subtilis cytochromes P450 CYP102A2 and CYP102A3: flavocytochrome homologues of P450 BM3 from Bacillus megaterium. Biochemistry. 2004;43:5474–5487.
- Scheps D, Honda Malca S, Richter SM, et al. Synthesis of ω‐hydroxy dodecanoic acid based on an engineered CYP153A fusion construct. Microb Biotechnol. 2013;6:694–707.
- Herrington R, Hock K, Autenrieth R. Flexible polyurethane foams. Midland, MI: Dow Chemical Company; 1997.
- Schneider S, Wubbolts MG, Sanglard D, et al. Biocatalyst engineering by assembly of fatty acid transport and oxidation activities for in vivo application of cytochrome P-450BM-3 monooxygenase. Appl Environ Microbiol. 1998;64:3784–3790.
- Schneider S, Wubbolts MG, Sanglard D, et al. Production of chiral hydroxy long chain fatty acids by whole cell biocatalysis of pentadecanoic acid with an E. coli recombinant containing cytochrome P450 BM-3 monooxygenase. Tetrahedron: Asymmetry. 1998;9:2832–2844.
- Lim SJ, Jung YM, Shin HD, et al. Amplification of the NADPH-related genes zwf and gnd for the oddball biosynthesis of PHB in an E. coli transformant harboring a cloned phbCAB operon. J Biosci Bioeng. 2002;93:543–549.
- Yasohara Y, Kizaki N, Hasegawa J, et al. Stereoselective reduction of alkyl 3-oxobutanoate by carbonyl reductase from Candida magnoliae. Tetrahedron: Asymmetry. 2001;12:1713–1718.
- Kubo T, Peters MW, Meinhold P, et al. Enantioselective epoxidation of terminal alkenes to (R)‐and (S)‐epoxides by engineered cytochromes P450 BM‐3. Chem A Euro J. 2006;12:1216–1220.
- Lu Y, Mei LH. Co-expression of P450BM3 and glucose dehydrogenase by recombinant Escherichia coli and its application in an NADPH-dependent indigo production system. J Ind Microbiol Biotechnol. 2007;34:247–253.
- Girhard M, Klaus T, Khatri Y, et al. Characterization of the versatile monooxygenase CYP109B1 from Bacillus subtilis. Appl Microbiol Biotechnol. 2010;87:595–607.
- Meng X, Yang J, Cao Y, et al. Increasing fatty acid production in E. coli by simulating the lipid accumulation of oleaginous microorganisms. J Ind Microbiol Biotechnol. 2010;38:919–925.
- Liu X, Sheng J, Curtiss III R. Fatty acid production in genetically modified cyanobacteria. Proc Natl Acad Sci. 2011;108:6899–6904.
- Sanwal B. Regulatory characteristics of the diphosphopyridine nucleotide-specific malic enzyme of Escherichia coli. J Biol Chem. 1970;245:1212–1216.
- Xu Z, Jing K, Liu Y, et al. High-level expression of recombinant glucose dehydrogenase and its application in NADPH regeneration. J Ind Microbiol Biotechnol. 2007;34:83–90.