ABSTRACT
The application of nanotechnology in medicine, diagnostics, therapeutics, drug delivery, etc. in order to overcome the limitations faced by these fields has already been established. Anaemia and osteoporosis are two of the most widely prevalent iron and calcium deficiency diseases among women. Conventional drugs for both have their inherent limitations which can be overcome by nanotechnology. Nanonization of drugs has been established as a very efficient way to enhance the bioavailability and absorption of the drug in the gastrointestinal tract. In addition to this, encapsulation of drugs in solid lipid nanoparticles is also employed to increase the stability of the drug in the gastrointestinal tract and to facilitate its controlled release. Nanotechnology is also applied in bone tissue engineering to prepare bone grafts for bone injury. Nanotechnology-based bone grafts have shown to possess enhanced mechanical properties and better biocompatibility and osteoconduction than the conventional bone grafts. This review article highlights the research trends and challenges of nanotechnology based drugs to combat iron deficiency anaemia and osteoporosis. This review outlines the recent research trends in nano-drugs and touches on issues concerning the market, industries and patents pertaining to drugs for anaemia and osteoporosis. To provide the safety and risk factors of nanomaterials, we have briefly highlighted the toxicological aspects of nanomaterials used in drugs.
Introduction
Nanotechnology has emerged as a science that has the potential to revolutionize any field it is applied to. Among the many applications of nanotechnology, one is the targeted delivery of specific nutrients, minerals, molecules or drugs. Recently, there has been a surge in the development of nanotechnology-based delivery systems for the targeted delivery of many drugs or to enhance the bioavailability of certain micronutrients in the body. The primary applications of nanomaterials in drug delivery are: to make the drug safer and more biocompatible, to reduce its toxicity without compromising with its therapeutic potential, for specific and targeted drug delivery and to increase its bioavailability and absorption in the body [Citation1]. Entrapment of the drugs in nanomaterials is done to overcome the limitations presented by the bulk non-encapsulated form of the drug. These limitations might be due to the insolubility of the drug in a particular form or conditions, susceptibility of the drug to physical or chemical damage, enzymatic degradation, damaging effect of the drug on the gastrointestinal tract (in case of oral intake), etc. Nano-size reduction of the drugs greatly enhances and alters their properties like stability, solubility, absorption, bioavailability, mechanism of action, etc. [Citation2–4]. Min et al. [Citation5] reported that the drug stability and tumour-targeting ability of camptothecin, encapsulated in glycol chitosan nanoparticles that have been hydrophically modified by conjugating 5β-cholanic acid moieties, were higher than those of free camptothecin, as observed in mice models. Lee Jia [Citation6] reported that nanonization of two poorly soluble drugs, carbenzandim and thiadiazole derivative, increases their oral bioavailability both in in vivo (SCID mice and Sprague–Dawley rats)and in vitro (Caco-2 cell lines) models. When the drugs were reduced to a size of 280 nm by pearl milling, their bioavailability was much higher than that of the micro-sized counterparts [Citation6]. Mahler et al. [Citation7] showed that oral exposure to polystyrene nanoparticles affected iron uptake and absorption through in vivo studies in chickens and in vitro studies in Caco-2 and HT29/MTX cell lines. High doses of nanoparticles caused the disruption of the cell membranes and increased the iron transport through them. Similar results were obtained from the intestinal loop model of the chicken, where acute exposure resulted in lower iron absorption than in chronically exposed chickens. Acute oral exposure to nanoparticles disrupted the iron transport, whereas chronic exposure to high doses of nanoparticles increased the iron transport and iron absorption by increasing the surface area of the intestinal lumen available for iron absorption by remodelling the intestinal villi [Citation7]. This study provides valid evidence for the increase in absorption of molecules when the gastrointestinal tract is exposed to nanoparticles and can be exploited to increase the bioavailability of nutrients and drugs that are administered orally.
These properties of nanomaterials can be exploited to create formulations or delivery systems that enhance the bioavailability and absorption of specific nutrients or minerals. They can be used to combat deficiency diseases that are widely prevalent in both developing and underdeveloped countries [Citation8–15]. Anaemia and osteoporosis are two of the most widely prevalent deficiency diseases, particularly among women. In this review, we discuss the recent research trends, challenges and opportunities of nanotechnology-based delivery systems for the benefits of women mainly – to overcome anaemia and osteoporosis.
Nanotechnology for iron deficiency anaemia
Iron deficiency is one of the most common nutritional disorders in the world prevailing in both developed and underdeveloped countries. Adequate iron concentration is crucial in the body for various metabolic processes like oxygen transport and storage, hormone synthesis, DNA replication and repair, cell cycle control and various enzymatic reactions. Iron deficiency anaemia (IDA) is caused by prolonged low levels of iron in the blood. Low levels of iron in the blood may be due to a variety of factors like iron-deficient diet, worm infestations, blood loss due to bleeding, intestinal diseases, etc. gives a list of the factors responsible for iron deficiency anaemia. It is particularly more prevalent in women of child-bearing age and children because of their increased requirement for iron for growth and developmental processes. According to the estimates of the World Health Organization (WHO), 48% of children between 5 and 14 years and 52% of pregnant women in developing countries are anaemic [Citation8]. shows the prevalence of anaemia among women belonging to different regions of the world. Plant-based diets have a high level of non-heme iron, which is poorly absorbed in the body as compared to heme iron, found in animal tissues [Citation9]. This is another reason for iron deficiency in the developing countries which rely mainly on food crops and plant-based diets. The presence of absorption inhibitors like phytic acids, phenolic compounds, and calcium in cereals and pulses also results in the low absorption of iron in the blood. Phytic acids and polyphenolic compounds cause chelation of the iron and reduce its absorption into the bloodstream [Citation10].
Figure 2. Worldwide prevalence of anaemia in women of reproductive age, based on WHO report on ‘The global prevalence of Anemia in 2011’. (Courtesy: Raw data has been collected from World Health Organization 2005 [8].)
![Figure 2. Worldwide prevalence of anaemia in women of reproductive age, based on WHO report on ‘The global prevalence of Anemia in 2011’. (Courtesy: Raw data has been collected from World Health Organization 2005 [8].)](/cms/asset/cd2a3f76-74ed-4052-a22f-4f927fa170a4/tbeq_a_1335615_f0002_oc.jpg)
Conventional strategies to deal with iron deficiency include iron fortification in food crops and iron supplementation. Development of iron biofortified crops is a good option to combat iron deficiency in rural areas which depend mainly on agriculture [Citation11]. However, there is a significant risk of excess iron as such crops will be consumed even by the non-deficient people. High levels of iron in the blood are associated with heart disease, kidney disease and diabetes. Iron supplementation relies on use of iron salts like ferrous sulphates, ferrous citrate, etc. Ferrous salts are mostly used because of their low cost and availability. However, they are highly reactive and are known to cause severe effects on the gastrointestinal tract and also affect the natural microbiota of the digestive system [Citation12]. Moreover, iron is poorly disposed off from the body; long-term consumption of artificial supplements should be avoided to prevent cellular accumulation of iron, which can lead to oxidative stress, lipid peroxidation and cancer. Thus, there is a need for a new form of therapy or a new form of iron supplementation that can improve the bioavailability of iron in the body by increasing its absorption to treat iron deficiency in a shorter duration and quickly enable the body to overcome anaemia. For these reasons, researchers and enterprises are now interested in employing nanotechnology for the successful delivery and enhancement of bioavailability of iron in the body.
Anaemia has attracted considerable attention of food scientists and technologists around the world and many nanostructure-based delivery systems for iron are being developed or research on such structures is being carried out. Only water-soluble forms of iron are suitable for food fortification but they result in undesirable colour changes and off-taste to the food, which rules out their direct use as food fortificants [Citation13]. The physiological forms of iron used for supplementation or fortification are known to have harmful effects on the gastrointestinal tract and also on the natural microbiota of the digestive system. Also, the generation of free radicals causes inflammation of the intestinal mucosa. Encapsulation of iron in a nanostructure ensures that it is delivered safely without having any harsh effects on the gastrointestinal tract. Encapsulation also ensures enhanced bioavailability and absorption because a decrease in the particle size increases its absorption through the gastrointestinal tract. Also, nano-size reduction increases the solubility and absorption of the molecules. This has been validated by the findings of Wu et al. [Citation14], who report that the absorption of non-heme iron is enhanced by anchovy muscle protein hydrosylate (AMPH) by the formation of nano-sized ferric hydrolysis products. They evaluated the effect of AMPH on the bioavailability and intestinal absorption of non-heme iron in rats and also studied the cellular uptake mechanism in vitro using Caco-2 cells. It was observed that the bioavailability was enhanced and the haemoglobin regeneration efficiency was increased [Citation14]. This proves that, in the nano-form, the bioavailability of the particles, here Fe, increases in the body.
Several studies have been conducted on how nanostructures can be formulated for the encapsulation of elemental iron or ferrous salts and their successful delivery inside the body to combat iron deficiency. Pereira et al. [Citation15] have developed 5–10 nm particles of iron oxo-hydroxide and modified them by the addition of tartaric and adipic acid tails to make them mimic the ferritin core. Ferritin is an iron storage and detoxification protein found in plants, animals and humans. They used animal and cellular models to test the efficacy and uptake of iron oxo-hydroxide nanomaterial by the cells. The toxicity was also tested on the human intestinal Caco-2 cell line and rats. The nanomaterial was found to have minimal cytotoxic effects on the cells and no undesirable effects were reported on the intestinal mucosa and faecal microbiota of the rats. Such a preparation has a strong potential to be used for the treatment of iron deficiency anaemia, as it does not have any significant toxic effects on the cells and the microflora of the gastrointestinal tract and has good solubility in the gastric acids. Moreover, the ligand modified nano-Fe structure closely resembles the naturally occurring ferritin found in the human body and hence, its uptake into the blood is enhanced. In the studies performed on mildly iron deficient menopausal female subjects, to determine the bioavailability of various ligand modified iron oxo-hydroxide nanoparticles, it was found that the absorption of ligand doped Fe (III) oxo-hydroxide nanoparticle was 80% as efficient as that of ferrous sulphate, commonly used in iron supplementation. The former was also three times better absorbed than the simple ferric chloride salt, doped with the same ligand. In addition, in the rat models, administration of iron oxo-hydroxide nanoparticles showed no structural or physiological changes and no abnormal Fe deposition was reported following 14 days of feeding [Citation15]. Thus, it can be said that such a formulation is safer and more efficient than most available conventional iron salts.
Zariwala et al. [Citation16] prepared solid lipid nanoparticles loaded with ferrous sulphate by the double emulsion solvent evaporation process. Solid lipid nanoparticles are widely used in drug targeting and delivery because of the multi-fold advantages they provide. Solid lipid nanoparticles provide the advantage of high absorption, biocompatibility and stability, unlike liposomes which are often unstable. The authors tested the absorption of the nanomaterial using human intestinal Caco-2 cell lines and used ferritin formation as a marker for iron absorption., Iron absorption from ferrous sulphate loaded lipid nanomaterial was found to be 13.42% higher than that from free ferrous sulphate. The higher absorption can be attributed to the presence of lipids in the nanomaterial. Lipids are known to enhance the intestinal absorption of encapsulated drugs or molecules by promoting particle transport across the bimolecular lipid membrane of the gut [Citation17]. Lipids also exhibit bioadhesive properties and adhere to the lining of the gastrointestinal tract, enhancing the cellular uptake [Citation16,Citation18]. Lipids can also modify the barrier function of the gastrointestinal tract by acting as permeability enhancers [Citation19]. The cytotoxicity of solid lipid nanoparticles was tested in Caco-2 cell lines using the MTT (methylthiazolyldiphenyl-tetrazolium bromide) assay. Solid lipid nanoparticles were found to have no significant cytotoxic effects on the Caco-2 cells. Since lipids are biocompatible with the biological systems, solid lipid nanoparticles can emerge as a very efficient delivery system for iron. It would overcome almost all the limitations and demerits presented by conventional iron supplementation drugs.
Another study on solid lipid particles was done by Hosny et al. [Citation20], who developed solid lipid nanoparticles loaded with iron. The solubility of iron in various solid lipids was measured and the effect of variation of various parameters of synthesis like the type and concentration of surfactant, duration of homogenization and ultrasonication on the particle size, zeta potential and entrapment efficiency was also determined. Iron solid lipid nanoparticles were prepared by hot emulsification and ultrasonication. The drug release was evaluated by the dialysis bag method and an in vivo pharmacokinetic study was done on male albino rabbits that were kept in a fasting state for 24 hours prior to the experiment. The evaluation of iron solid lipid nanoparticles was done in comparison to the iron tablets available in the market. It was found that the drug release efficiency and the bioavailability of iron were higher in solid lipid nanoparticles than in the iron tablets available in the market. The bioavailability of iron was increased fourfold by its incorporation into the solid lipid nanoparticles. Iron salts have poor aqueous solubility and iron formulation into solid lipid nanoparticles enhanced both its solubility and tissue permeability. The presence of lipids also facilitates absorption by various mechanisms like intracellular or paracellular uptake through Peyer's patches, bypassing the liver by direct absorption into the lymphatic circulation. They can also be administered by various routes such as tropical, oral, ophthalmic, rectal and parenteral routes [Citation20]. Thus, such lipid nanoparticle delivery systems can very efficiently enhance the release and bioavailability of iron in the body. Lipids, however, have a tendency to clog the pores and channels in the cell membranes, which still needs to be thoroughly investigated.
Ferric pyrophosphate is a water insoluble iron compound which is used as a food fortificant in chocolate drinks and infant cereals to enhance the iron absorption. Due to its low solubility in dilute acids, it dissolves poorly in the gastric acids and, hence, is poorly bioavailable for absorption. Srinivasu et al. [Citation13] synthesized nano-sized ferric pyrophosphate ([FeO4(P2O7)3]) by titration of sodium pyrophosphate solution against ferric chloride in the presence of ethylene glycol at 65 °C with continuous stirring to avoid agglomeration of the particles. The bioavailability of nano-sized ferric pyrophosphate was evaluated in iron-depleted rats by feeding them with food fortified with nano-sized ferric pyrophosphate for 15 days. A part of the experimental rats were fed with the same dose of FeSO4 for the same duration to compare the level of bioavailability in both the experimental groups. The relative bioavailability of ferric pyrophosphate was found by calculating the haemoglobin regeneration efficiency. Its relative bioavailability was calculated to be 103.2% with respect to that of ferrous sulphate. Oral toxicity studies in rats gave no indication of toxic effects of nano-sized ferric pyrophosphate. The higher bioavailability of nano-sized ferric pyrophosphate than ferrous sulphate can be attributed to the increased solubility of ferric pyrophosphate in dilute acids because of the reduction in particle size. It is an established fact that the solubility of metals increases with the decrease in particle size [Citation13].
Another nano-based formulation for iron deficiency treatment was attempted by Salah et al. [Citation21]. They developed magnetite (Fe3O4) nanoparticles capped with vitamin C (ascorbic acid), since iron absorption in the intestinal lumen is facilitated by vitamin C. The effectiveness of the formulation was tested on albino rats. It was found that intra-peritoneal or oral administration of the above drug can effectively cure iron deficiency anaemia. Bone marrow studies of the experimental rats showed that the magnetite nanoparticles capped with vitamin C stimulated erythropoiesis without affecting the haemostasis. Administration of a single dose increased the haemoglobin concentration from 7 to 14 g/dL and the red blood cell counts increased from 3.2 × 106 to 6.5 × 106/mm3 within 10 days [Citation21]. European Egyptian Pharmaceutical Industries (EEPI) holds a patent for the development of iron-based nanocomposites for the treatment of iron deficiency anaemia [Citation22]. Iron oxide (magnetite) nanoparticles capped with a mixture of multivitamins, folic acid, nicotinic acid and ascorbic acid was developed. Folic acid and nicotinic acid are important for cell formation and vitamin C is required for the absorption of iron from the intestinal lumen. The authors developed two forms of the drug: gel-based and aqueous solution for oral administration. The formulation was tested in five-week-old albino rats, in which anaemia was induced by withdrawing blood from the canthus of eyes. A healthy, non-anaemic group of animals was kept as the control. The rats were fasted for six hours prior to administration of drugs. The experimental rats were administered different concentrations of nano iron oxide depending on their body weight, ferric chloride (for comparison) or magnetite nanoparticles coated with multivitamin mixture. It was observed that just a single dose of 0.8 mg of magnetite nanoparticles capped with multivitamin mixture was able to treat iron deficiency anaemia and to recover the erythrocytes in less than four days. The haemoglobin levels rose from 4.4 to 14.6 g/dL in 7 days and stabilized at 13.6 g/dL 80 days after administration. This is much faster than the results with ferric chloride. The vitamin mixture was able to correct anaemia but did not reach the same level as magnetite nanoparticles. Thus, a single dose of magnetite nanoparticles capped with multivitamin mixture, equivalent to 8.3 mg, can correct iron deficiency anaemia in albino rat in less than four days. This is faster and more efficient than most commercially available treatments. In addition, because of the low concentration of nano iron oxide required to significantly correct anaemia, the drug has minimal toxic side effects on the body, unlike conventional iron tablets which have a lot of side effects [Citation22]. Along with nano-size magnetite particles, vitamin C also enhances the absorption of Fe through the intestinal villi. Thus, this combination can enhance the bioavailability of iron and has the potential for being commercialized as a treatment of iron deficiency. Such a product has a lot of scope to be used for treatment of iron deficiency but there are certain parameters that still need to be investigated or evaluated, like, the solubility of the nanoparticles in the gastric acids, their effect on the gastrointestinal cells, their biocompatibility and bioavailability in the human body and long-term effects of their consumption. Since the physiology of the human body is different from that of rats, the effects of magnetite nanoparticles need to be tested in human trials as well, prior to their commercial use as a therapeutic agent.
Thus, it is quite clear that the use of nanostructures for the delivery of iron or iron salts provides benefits that are absent in conventional remedies for iron deficiency. The use of nanomaterials has a potential to circumvent the harmful effects of iron salts, namely ferrous sulphate, on the gastrointestinal tract and on the natural microbiota of the digestive system. gives a diagrammatic representation of the difference between the oral intake of nano-encapsulated Fe drug and encapsulated Fe drug. It has the additional advantage of rendering enhanced bioavailability and absorption compared to conventional iron salts.
Figure 3. A pictorial representation of the intake of Fe2+ drugs, non-encapsulated and nano-encapsulated, and their effect on the gastrointestinal tract. Conventional drugs containing iron salts (left panel) have a damaging effect on the gastrointestinal tract. Some of the drugs are acted upon by the gastric juices, which reduces the drug bioavailability of. Encapsulation of the drug in a lipid nanostructure (right panel) saves the gastrointestinal tract from the harsh effects of the drug and also increases the bioavailability of the drugs.
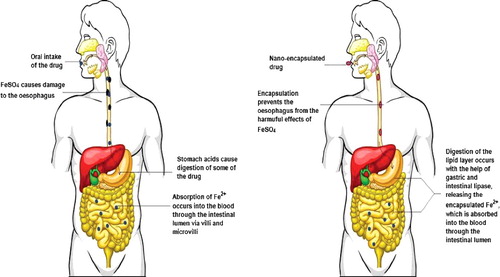
Nanotechnology for osteoporosis
Calcium deficiency is another common deficiency disorder prevalent mostly in developing countries due to low dietary intake of calcium [Citation23]. Apart from maintaining bone strength and regeneration, calcium also plays a role in blood clotting, nervous system functioning, muscle contraction and cell signalling. Calcium deficiency is usually accompanied by vitamin D deficiency, since vitamin D is necessary for the absorption of calcium from the intestinal lumen into the blood [Citation24]. Deficiency of calcium leads to decreased bone mineralization; when the calcium intake is low, calcium deposits present in the bones are utilized to replenish and maintain homeostasis in various body fluids and tissues. This leads to decreased bone density [Citation25]. Osteoporosis is the thinning of bones caused by a variety of factors such as age, menopause, alcoholism, etc. Calcium and vitamin D deficient diet is also an important cause of osteoporosis. Especially in women, bone thinning starts at the onset of menopause and calcium deficiency can subsequently lead to osteoporosis. Ho et al. [Citation26] conducted a 30-month study on 438 pre-menopausal Chinese women to identify the relation between menopause and bone changes. It was observed that an annual bone loss of 0.5% occurred in pre-menopausal women, 2%–2.5% in transitional women and 1.5% in post-menopausal women [Citation26]. As shown in [Citation27], the incidence of osteoporosis is generally higher in women than in men. Such bone density changes in menopausal women can be attributed to the decreased levels of oestrogen during menopause. Oestrogen, along with developing female secondary sexual characteristics, is also involved in maintaining the bone strength. Therefore, a fall in the levels of oestrogen during menopause increases the incidence of osteoporosis in women. Oestrogen treatment is recommended for post-menopausal osteoporosis management. However, oestrogen treatment has its own side effects and is not suitable for all women [Citation28].
Figure 4. Incidence of osteoporosis in males and females (per thousand) of different origins. It is evident from the graph that the incidence of osteoporosis in women is much greater than that in men. (Courtesy: Raw data has been adopted from [Citation27].)
![Figure 4. Incidence of osteoporosis in males and females (per thousand) of different origins. It is evident from the graph that the incidence of osteoporosis in women is much greater than that in men. (Courtesy: Raw data has been adopted from [Citation27].)](/cms/asset/8ae91fe9-4ec8-4383-a04d-c89b68fe4c9a/tbeq_a_1335615_f0004_oc.jpg)
Conventional treatments for calcium deficiency include intake of calcium supplements containing calcium carbonate, calcium citrate or calcium phosphate. Calcium supplements have been found to slow down bone loss and also increase the axial bone density in menopausal women [Citation29]. However, intake of calcium supplements, in turn, has some side effects like constipation and increased incidence of cardiac diseases and stroke. Pentti et al. [Citation30] performed a study on 10,555 women in the age group of 52–62 years to analyse the effect of calcium and vitamin D supplementation on coronary heart disease in women. It was observed that, among the 2723 subjects who regularly took calcium supplements, 513 women were diagnosed with coronary heart disease [Citation30]. However, in another report, Shin and Kim [Citation31] state that the association of cardiac diseases with calcium supplementation has still not been established due to absence of proper investigation and evidence. In either case, the side effects of artificial supplementation cannot be disregarded. The inevitable side effects of artificial supplements lead us to look into the field of nanotechnology to combat calcium deficiency. So far, only a few studies have been done regarding the application of nanotechnology in dealing with calcium deficiency.
Khajuria et al. [Citation32] developed a nano-drug for osteoporosis that includes risedronate loaded onto zinc hydroxyapatite nanoparticles by an absorption method. Risedronate has a high affinity for bone and is used as the targeting moiety. Nano-sized hydroxyapatite has been found to increase the bioactivity of osteoblasts and also to increase bone regeneration [Citation33–36]. The risedronate/zinc–hydroxyapatite particles were tested in ovariectomized rats/mice. Ovariectomy was performed to induce osteoporosis and prevent bone regeneration. In this study, the drug was injected intravenously to the rats. The drug was found to have anti-osteoporotic effect and to correct the bone loss in the ovariectomized rats. Thus, it was demonstrated that the drug not only prevented bone loss, but also stimulated bone growth. The combination of risedronate and hydroxyapatite proves to be a very effective way to deliver both calcium and phosphate, necessary for bone strength [Citation33]. Further study can be done in this relation to find whether the oral route of delivery can be used for this formulation, as oral intake would be more favourable and easier for the patients. In addition, further clinical studies are strongly recommended to evaluate the potential side effects of such a formulation, as it has great potential to be commercially used for the treatment of osteoporosis.
Balasundaram and Webster [Citation37] reported that bioactive compounds and drugs functionalized onto the surface of magnetic nanoparticles can be delivered directly to the osteoporotic bone (and not the healthy bone) and regenerate it. Magnetic nanoparticles can be transported to specific tissues or target site by an externally applied magnetic field and they lose their magnetism once the external magnetic field is removed. In this study, the authors functionalized biodegradable nanoparticles of hydroxyapatite with bioactive compounds that bind to bones of low biomass. These functionalized bioactive molecules were then placed on the outer surface of magnetic nanoparticles using various techniques, such as covalent chemical attachment. After binding to the osteoporotic bone, the nanoparticles deliver bioactive molecules to it to stimulate bone regeneration. What is more, the slow degradation of hydroxyapatite ensures that there is sustained long-term bone regeneration. One additional advantage provided by magnetic nanoparticles is that by using magnetic resonant imaging, the drug activity can be located and monitored. Further study is required to evaluate the attachment efficiency of magnetic nanoparticles to healthy and osteoporotic bones, determine release profiles and evaluate the toxic potential [Citation37,Citation38].
Critical Pharmaceuticals in collaboration with the University of Nottingham is developing a nano-enabled nasal formulation of teriparatide for the treatment of osteoporosis. Teriparatide is a parathyroid hormone and stimulates osteoblasts for bone growth [Citation39]. Present treatment involves daily injection of teriparatide, which can be quite vexing. An absorption enhancer, CriticalSorb, developed by the company itself using nanotechnology, is being used to formulate a nasal spray. The intranasal formulation will be non-invasive and more efficient than the conventional drug because of the enhanced absorption provided by nanotechnology (Information on the current status of the drug is unavailable and all the information discussed here is cited from news transcripts dated February 2012) [Citation39]. The higher absorption and bioactivity can be attributed to the fact that a reduction in particle size greatly enhances the absorption and activity of the drug or molecule by increasing the overall surface-area-to-volume ratio. This is also confirmed by the findings of Erfanian et al. [Citation3] in their study on the influence of nano-size reduction on calcium absorption and bioavailability in rats. Ovariectomized (OVX) and OVX-osteoporosis rats were fed with milk powder nano-fortified with calcium and the calcium absorption and bioavailability was investigated in both these groups. It was observed that the bone calcium and bone-breaking strength increased with the consumption of nano-fortified calcium. Calcium absorption in OVX and OVX-osteoporosis rats was 63.54% and 89.06%, respectively. The bioavailability of calcium from fortified and nano-fortified milk powders in OVX rats was 24.64% and 41.65%, respectively. The calcium bioavailability in the OVX-osteoporosis group from fortified and nano-fortified milk was reported to be 9.74% and 30.17%, respectively [Citation3]. Another related study was conducted by Huang et al. [Citation40] on the effect of nano calcium carbonate and nano calcium citrate on the bioavailability of calcium in OVX mice models and the toxic effects. It was observed that, at a nano calcium citrate and nano calcium carbonate concentration of 2.3 and 1.3 g/kg, there was no toxic effect. Instead, there was an increase in the serum calcium levels and the whole-body bone mineral density in the OVX mice. Thus, it appears that nano-size reduction dramatically enhances the absorption and bioavailability of calcium, as nanoparticles have greater ability to cross the wall of the intestine and also the way they are treated in the gastrointestinal tract gets altered [Citation2]. However, with size reduction, the tendency of the particles to escape into unintended tissues or organs of the body increases and they can prove to be harmful to those organs or tissues. Hence, such side effects also need to be evaluated prior to the use of nano-sized calcium as a calcium supplement.
Tokudome et al. [Citation41] conducted a study on the jaw bone mineral density and mechanical strength of osteoporosis rats administered with zinc-containing β-tricalcium phosphate nanoparticles (ZnTCP). OVX osteoporosis rats were injected with ZnSO4 and ZnTCP powder dissolved in corn oil around the jaw bone. The bone mineral content was measured using an X-ray Computed Tomography scan and the bone mechanical strength was measured using the three-point bending technique. The bone mineral content was found to be 382.0 and 352.5 mg for ZnSO4 and ZnTCP, respectively. The bone mechanical strength was found to be 98.9 and 157.0 N for ZnSO4 and ZnTCP, respectively. ZnTCP induced sufficient bone formation because it is released slowly and in a controlled manner due to its low solubility in body fluids. ZnSO4, on the other hand, is readily soluble due to which the injected ZnSO4 particles immediately diffused into the tissues and were absorbed, dissolved or distributed throughout the body so that therapeutically significant levels on Zn could not be attained around the osteoporosis bone. Thus, it was found that ZnTCP nanoparticles could control the release of Zn and effectively induce osteogenesis in osteoporosis bones [Citation41]. However, the harmful effects of ZnTCP nanoparticles need to be weighed against their therapeutic potential prior to their use as treatment for osteoporosis.
Kumar et al. [Citation42] conducted an in vivo study on the efficacy of a prototype formulation based on layer-by-layer nano-matrix bearing kaempferol for the treatment of osteoporosis. Kaempferol is a flavanoid associated with good skeletal health [Citation43,Citation44]. It has been demonstrated to overcome the deleterious effect on bone density in oestrogen deficiency [Citation43], to exhibit an anti-osteoclastogenic effect [Citation45,Citation46] and to promote osteoblast differentiation [Citation42]. Layer-by-layer nano-matrix was prepared by the sequential absorption of two bilayers of sodium alginate and protamine sulphate over the CaCO3 loaded kaempferol microparticles. OVX Sprague–Dawley rats were administered with kaempferol and formulated kaempferol for 12 weeks, at the end of which, the plasma and bone marrow kaempferol levels increased by 2.8-fold and 1.75-fold, respectively, in comparison to those in the rats given free kaempferol. The bone micro-architecture was well maintained up to 30 days after withdrawal of formulated kaempferol. However, deterioration started within 15 days in the osteoporosis rats administered with free kaempferol [Citation47]. It is a novel formulation to increase the bioavailability of flavanoids for the stimulation of osteoblasts and maintenance of skeletal health. Kaempferol has the additional advantage that it is completely non-toxic. Free kaempferol is poorly bioavailable and, hence, is not used for the treatment of osteoporosis. However, such a formulation can increase its bioavailability and might potentially treat osteoporosis.
Various research works, thus, provide evidence for the superior performance of nanomaterial-based drugs over the conventional drugs for the treatment of osteoporosis. Nanomaterial-based drugs even provide minimally invasive therapies with remarkable results, not well-accomplished by conventional therapies. This could also replace the hormone therapy given to osteoporotic women, which has serious side effects [Citation28]. Similarly, in the case of iron deficiency anaemia, nanotechnology-based drugs could be used to achieve significant results even in lower dosage and with minimum side effects. The only hurdle faced is the evaluation of these proposed concepts in human trials and subsequent commercialization. gives an overview of the recent research work and patents that have proposed novel nanotechnology-based treatments for iron deficiency anaemia and osteoporosis.
Table 1. Recent research work and patents that have proposed novel nanotechnology-based treatments for iron deficiency anaemia and osteoporosis.
Nanotechnology in bone tissue engineering
There has been an increase in the occurrence of bone disorders and conditions and it is expected to double by 2020 [Citation56]. These are mostly prevalent in communities that lead a sedentary lifestyle in which aging increases the incidence of obesity and hence, bone disorders. Bone conditions are also prevalent in underprivileged communities that have low dietary intake of calcium and other vitamins and minerals. Autologous and allogenic transplantation of bone tissue has traditionally been used for bone repair and regeneration [Citation56]. However, due to the inherent limitations of both these methods, efforts have been made to construct bone grafts using the principles of tissue engineering, biomaterials, and very recently, using nanotechnology [Citation57]. Scaffolds meant for bone tissue engineering should have the following properties: biocompatibility, remarkable porosity and pore size of less than 100 µm, biodegradability, ability to hold growth factors, mechanical strength equivalent to cortical bone and low cost [Citation58]. The use of nanomaterials to engineer scaffolds covers mostly all of these requirements and, hence, is becoming widely popular for bone tissue engineering. gives a list of nanomaterials commonly used to develop scaffolds in bone tissue engineering and also outlines the properties that the nanomaterials impart to the scaffold. gives a schematic representation of various nanoparticles used in bone tissue engineering and properties imparted by them to the final scaffold. gives a schematic representation of the steps involved in the manufacturing of scaffolds for bone tissue engineering.
Table 2. Recent advances in construction of chitin/chitosan-based scaffolds incorporated with nano-sized biomaterials.
Figure 5. Schematic representation of the various biomaterials used in conjugation with chitosan- and chitin-based scaffolds and the respective properties they impart to the scaffold.
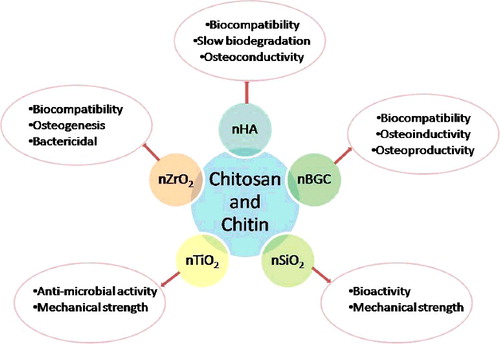
Figure 6. Steps involved in bone tissue engineering. Mesenchymal cells are isolated from the donor patient and differentiated into osteoblasts in vitro before loading onto engineered scaffolds consisting of polymers, biomaterials, growth factors, etc. The scaffolds are then implanted onto the bone to initiate bone repair and regeneration (Figure has been recreated from [Citation76]).
![Figure 6. Steps involved in bone tissue engineering. Mesenchymal cells are isolated from the donor patient and differentiated into osteoblasts in vitro before loading onto engineered scaffolds consisting of polymers, biomaterials, growth factors, etc. The scaffolds are then implanted onto the bone to initiate bone repair and regeneration (Figure has been recreated from [Citation76]).](/cms/asset/578aa387-ad22-4c95-bce5-573ea1d6867d/tbeq_a_1335615_f0006_oc.jpg)
Deepthi et al. [Citation59] and Mahoney et al. [Citation60] review the applications of chitin- and chitosan-based nanocomposites for construction of scaffolds for bone tissue engineering. Chitin and chitosan are ideal materials for bone tissue engineering because of their biocompatibility, biodegradability, non-antigenicity, structural similarity to glycosaminoglycans (major components of the extracellular matrix of the bone), ability to form highly porous scaffolds with interconnected pores, ability to enhance bone formation and osteoconductivity. They can be engineered into various forms like beads, microparticles, gel, nanoparticles, scaffolds, nanofibres, etc. Different biomaterials (summarized in ) can be incorporated into the chitin and chitosan scaffolds to improve their mechanical strength [Citation61], biocompatibility [Citation59,Citation62] and osteogenesis [Citation61–66]. The following biomaterials are mainly incorporated into the scaffolds: nano- hydroxyapatite (nHAp), nano-bioactive glass ceramics, nano-silicon dioxide (nSiO2), nano-titanium oxide (nTiO2), nano-zirconium oxide (nZrO2) [Citation67–72]. Hydroxyapatite is the most ideal candidate for composite incorporation because it is structurally similar to the natural bone components’ osteoconductivity and biocompatibility. Nano-sized hydroxyapatite provides numerous advantages over micro-sized hydroxyapatite because it easily gets dispersed in the polymer matrix and forms regular pores instead of irregular pores [Citation73]. Uswatta et al. [Citation54] developed injectable porous scaffolds from chitosan biopolymer, nano-hydroxyapatite and sodium tripolyphosphate (to crosslink nano-hydroxyapatite and chitosan) by using non-toxic coacervation and lypophilization techniques. This was based on the hypothesis that incorporation of nano-hydroxyapatite could improve the strength of the scaffold and also stimulate osteoconduction, since it can emulate the mineral structure of the bone. Murine osteoblast (OB-6) cell lines were used to evaluate the cell viability, attachment and proliferation. The scaffolds showed good results for cell proliferation for nHA in 2% (w/v) chitosan. No toxic effects were found on the cells. The authors also found that increasing nano-hydroxyapatite increased the cell adhesion and proliferation on the scaffolds. The mechanical strength of the scaffolds can also be increased by reducing the pore sizes [Citation54]. The findings of the above study are also substantiated by the work of Sharma et al. [Citation74], in which a bone regeneration scaffold was prepared using nano-hydroxyapatite, chitosan, gelatin and alginate by using a foaming method (without surfactant). Thus, nano-hydroxyapatite is an excellent means of bone regeneration, as it can easily be injected to the target site in a minimally invasive manner. However, in vivo studies are required to evaluate its side effects and to understand how well it can be applied for bone repair in humans. In another study, in vivo trials were performed in rabbits to test the bone formation ability of a nano-hydroxyapatite–fucoidan nanocomposite. The prepared nanocomposite was inserted in the tibia of osteomitized rabbits. Slight bone formation was observed in the damaged bone [Citation75]. However, the exact role of fucoidan in bone formation could not be established and needs further examination.
Nano-bioglass scaffold is another novel method of bone tissue engineering. Bioactive glass consists of SiO2, CaO, P2O5 and Na2O. A thin layer of hydroxyapatite or hydroxycarbonate apatite forms on the glass surface, when it comes in contact with the biological fluids, which leads to the binding of soft tissues in the bone. Since the components of bioactive glass are those naturally found in the body, the glass is eventually absorbed and replaced by bone. The angiogenic potential of bioglass is enhanced by doping it with alum. It has been found that bioglass doped with alum exhibits higher cytocompatibility and biocompatibility than bioglass alone [Citation93]. However, that study failed to bring out the advantages of bioglass over other biomaterials for use in bone tissue engineering. It also failed to evaluate the toxic effects of the components of the glass on surrounding cells and tissues and whether those are present in levels that are clinically recommended and safe for the human body or not.
Alginate is also used for construction of scaffolds owing to its biocompatibility, biodegradability, non-toxicity, non-immunogenecity, easy commercial availability and low cost. It can also be modified into desired forms like microcapsules, microspheres, hydrogels, sponges, fibres and foam. Alginate is incorporated with chitosan and/or various nano-sized biomaterials like hydroxyapatite, SiO2, bioactive glass, calcium phosphate cement, etc. to improve its mechanical and osteoconductive properties [Citation58].
Challenges
The potential of nanotechnology to fight deficiency diseases like anaemia and osteoporosis is, no doubt, remarkable. However, it faces certain challenges that still need to be overcome. One of the main challenges it faces, is that there is very little information available with respect to the fate of the nanomaterial once it enters the systemic circulation through any route. This makes it very challenging to develop nano-based drugs or targeting moieties as the information pertaining to it is limited. Usually, drugs are tested on animal models like rats, mice or rabbits, but the same physiology and drug interaction is hardly applicable to the human body [Citation94–99]. This increases the chances of failure of the drug or might result in the drug reaching untargeted sites. Also, this limitation makes it difficult to quantify the correct dose of the drug that is required to bring out the desired effect that is safe and tolerable for the body. For example, in the case of nano-Fe drugs for treatment of anaemia, the correct quantity of drug needs to be administered as a high dose, resulting in harmful side effects and potentially causing accumulation of iron in the body which is, again, detrimental. Another challenge is the transport of the nano-drugs across the compartmental boundaries like endothelial and epithelial barriers of vessels, placental and intestinal walls, etc. Another type of barriers faced by the drug are in the form of degrading enzymes, scavenging phagocytes, abnormal flow of blood and hydrostatic pressure at target sites and molecular and ionic efflux pumps that can expel the drug out of the cell [Citation100]. Thus, detailed knowledge of the interaction of nano-drugs with these barriers is important to design a successful, barrier-resistant drug.
Another challenge related to the manufacturing of nanomaterials for drug delivery, is the large-scale production. There is a need to scale up the laboratory or pilot technologies for consistent and reproducible production and commercialization. It is difficult to modify or control the desired properties of these nanomaterials at large scale. The main challenges to scale up the process include agglomeration, low concentration of nanomaterials and chemical processes. For instance, maintaining the size and composition of nanomaterials at laboratory scale is possible, whereas it is a challenge at large-scale production of these nanomaterials. Although a number of drug delivery technologies have been patented, the commercialization of these technologies is still at its early stage. The main reason of the more limited commercialization is the gap between academic research and pharmaceutical companies because this type of research has been mostly carried out by researchers in universities. Therefore, there should be partnership between universities and pharmaceutical companies so that these technologies can reach into the market [Citation101,Citation102].
Along with the augmented research and development work on drug delivery, safety concerns about the uses of these technologies are also emerging. Nanoparticles have distinct properties from corresponding bulk particles, such as surface characteristics, physicochemical properties and crystal structure. Hence the distinct toxicity of nanoparticles [Citation103,Citation104]. There are various ways of entry of nanoparticles into the human body, such as dermal, gastrointestinal and respiratory routes. The smaller size and larger surface area of nanoparticles than their corresponding bulk materials are the main factors that contribute to the toxicity of nanomaterials. Due to the small size of nanoparticles, they can easily pass through the biological barriers and membranes and can cause toxicity at various sites in the body, i.e. genotoxicity, carcinogenic potential and oxidative stress in organelles, mitochondrial stress and cell-wall disruption [Citation105–107]. Therefore, the safety and the possible impact of these nanomaterials should not only be considered for the affected population, but should also cover the entire manufacturing and disposal processes [Citation102]. Proper regulatory guidelines must be established for the assessment and evaluation of nanotechnology-based drugs and products.
Conclusions
Technologies to encapsulate micronutrients to increase their bioavailability, safety and stability are either already under development or active research is underway pertaining to such technologies. Such technology can not only be utilized to overcome the limitations of the conventional drugs and nutrient supplements, but they can also provide better results than the conventional drugs. Currently, it is necessary to develop low cost and safe nano-based drugs or formulations to overcome deficiency diseases, highly prevalent in developing and underdeveloped countries. It is imperative to bring nanotechnology based drugs from the laboratory into large scale manufacturing and conduct clinical trials for the same so that they can be put to use as quickly as possible. Nanotechnology provides abundant advantages and benefits which must be exploited for the betterment of mankind.
Acknowledgment
A. Shukla acknowledges Dr Alka Shukla and Mr Vimal Kant Shukla, Kanpur, Uttar Pradesh, India, for all their technical support throughout the work.
Disclosure statement
No potential conflict of interest was reported by the authors.
Additional information
Funding
References
- De Jong WH, Borm PJA. Drug delivery and nanoparticles: applications and hazards. Int J Nanomed. 2008;3(2):133–149.
- Chaudhry Q, Scotter M, Blackburn J, et al. Applications and implications of nanotechnologies for the food sector. Food Addit Contam. 2008;25(3):241–258.
- Erfanian A, Mirhosseini H, Manap MYA, et al. Influence of nano-size reduction on absorption and bioavailability of calcium from fortified milk powder in rats. Food Res Int. 2014;66:1–11.
- Erfanian A, Mirhosseini H, Rasti B, et al. Absorption and bioavailability of nano-size reduced calcium citrate fortified milk powder in ovariectomized and ovariectomized-osteoporosis rats. J Agric Food Chem. 2015;63(24):5795–5804.
- Min KH, Park K, Kim Y-S, et al. Hydrophobically modified glycol chitosan nanoparticles-encapsulated camptothecin enhance the drug stability and tumor targeting in cancer therapy. J Control Release. 2008;127(3):208–218.
- Jia L. Nanoparticle formulation increases oral bioavailability of poorly soluble drugs: approaches experimental evidences and theory. Curr Nanosci. 2005;1(3):237–243.
- Mahler GJ, Esch MB, Tako E, et al. Oral exposure to polystyrene nanoparticles affects iron absorption. Nat Nanotechnol. 2012;7(4):264–271.
- Database WHOG. [Internet]. Worldwide prevalence of anaemia. 2005. cited [2016 Nov 10]. Available from: http://www.who.int/vmnis/publications/anaemia_prevalence/en/
- Hurrell R, Egli I. Iron bioavailability and dietary reference values. Am J Clin Nutr. 2010;91(5):1461S–1467S.
- Vikas K, Sinha AK, Makkar HPS, et al. Dietary roles of phytate and phytase in human nutrition: a review. Food Chem. 2010;120(4):945–959.
- Saini RK, Nile SH, Keum Y. Food science and technology for management of iron deficiency in humans: a review. Trends Food Sci Technol. 2016;53:13–22.
- Saha L, Pandhi P, Gopalan S, et al. Comparison of efficacy, tolerability, and cost of iron polymaltose complex with ferrous sulphate in the treatment of iron deficiency anemia in pregnant women. MedGenMed. 2007 [cited 2017 Mar 29];9(1):1. Available from:https://www.ncbi.nlm.nih.gov/pmc/articles/PMC1924983/
- Srinivasu BY, Mitra G, Muralidharan M, et al. Beneficiary effect of nanosizing ferric pyrophosphate as food fortificant in iron deficiency anemia: evaluation of bioavailability, toxicity and plasma biomarker. RSC Adv. 2015;5(76):61678–61687.
- Wu H, Zhu S, Zeng M, et al. Enhancement of non-heme iron absorption by anchovy (Engraulis japonicus) muscle protein hydrolysate involves a nanoparticle-mediated mechanism. J Agric Food Chem. 2014;62(34):8632–8639.
- Pereira DIA, Bruggraber SFA, Faria N, et al. Nanoparticulate iron(III) oxo-hydroxide delivers safe iron that is well absorbed and utilised in humans. Nanomedicine Nanotechnology, Biol Med. 2014;10(8):1877–1886.
- Zariwala MG, Elsaid N, Jackson TL, et al. A novel approach to oral iron delivery using ferrous sulphate loaded solid lipid nanoparticles. Int J Pharm. 2013;456(2):400–407.
- Eldridge JH, Hammond CJ, Meulbroek JA, et al. Controlled vaccine release in the gut-associated lymphoid tissues. I. Orally administered biodegradable microspheres target the Peyer's patches. J Control Release. 1990;11(1–3):205–214.
- Severino P, Andreani T, Macedo AS, et al. Current state-of-art and new trends on lipid nanoparticles (SLN and NLC) for oral drug delivery. J Drug Deliv. 2012; [cited 2017 Mar 29];2012:750891. DOI: 10.1155/2012/750891
- Aungst BJ. Intestinal permeation enhancers. J Pharm Sci. 2000;89(4):429–442.
- Hosny KMKM, Banjar ZM, Hariri AH, et al. Solid lipid nanoparticles loaded with iron to overcome barriers for treatment of iron deficiency anemia. Drug Des Dev Ther. 2015;9(9):313–320.
- Salah EDTA, Bakr MM, Kamel HM, inventors; Innovative Research and Development Co. (Inrad), assignee, et al.. Magnetite nanoparticles as a single dose treatment for iron deficiency anemia. Google Patents. 2010; cited [2016 Nov 15]. Available from:http://www.google.co.in/patents/WO2010034319A1?cl=en
- Mahmoud MBM, Helmy SHA.inventors; European Egyptian Pharmaceutical Industries, assignee. Novel formula of iron based nanocomposites for rapid and efficient treatment of iron deficiency anemia. Google Patents. 2014; cited [2016 Nov 15]. Available from:http://www.google.co.in/patents/WO2014135170A1?cl=en
- Orphan Nutrition. Micronutrient : calcium [Internet]. 2016. cited [2016 Nov 15]. Available from: http://www.orphannutrition.org/orphan-nutrition-library/micronutrient-malnutrition-calcium/
- Christakos S, Dhawan P, Porta A, et al. Vitamin D and intestinal calcium absorption. Mol Cell Endocrinol. 2011;347(1–2):25–29.
- FAO,WHO. Vitamin and mineral requirements in human nutrition Second edition. World Heal Organ [Internet]. 1998;1–20. cited [2016 Nov 16]. Available from:www.who.org
- Ho SC, Chan SG, Yip YB, et al. Change in bone mineral density and its determinants in pre- and perimenopausal Chinese women: the Hong Kong perimenopausal women osteoporosis study. Osteoporos Int. 2008;19(12):1785–1796.
- Johnell O, Kanis JA. An estimate of the worldwide prevalence and disability associated with osteoporotic fractures. Osteoporos Int. 2006;17:1726–1733.
- Lindsay R. Estrogen and osteoporosis. Phys Sportsmed. 1987;15(11):105–108.
- Reid IR, Ames RW, Evans MC, et al. Effect of calcium supplementation on bone loss in post menopausal women. N Engl J Med. 1993;328(7):460–464.
- Pentti K, Tuppurainen MT, Honkanen R, et al. Use of calcium supplements and the risk of coronary heart disease in 52–62-year-old women: the Kuopio Osteoporosis Risk Factor and Prevention Study. Maturitas. 2009;63(1):73–78.
- Shin CS, Kim KM. The risks and benefits of calcium supplementation. Endocrinol Metab. 2015;30(1):27–34.
- Khajuria DK, Disha C, Vasireddi R, et al. Risedronate/zinc-hydroxyapatite based nanomedicine for osteoporosis. Mater Sci Eng C. 2016;63:78–87.
- Iafisco M, Ruffini A, Adamiano A, et al. Biomimetic magnesium-carbonate-apatite nanocrystals endowed with strontium ions as anti-osteoporotic trigger. Mater Sci Eng C. 2014;35(1):212–219.
- Lin L, Chow KL, Leng Y. Study of hydroxyapatite osteoinductivity with an osteogenic differentiation of mesenchymal stem cells. J Biomed Mater Res A. 2009;89(2):326–335.
- Webster TJ, Ergun C, Doremus RH, et al. Enhanced functions of osteoblasts on nanophase ceramics. Biomaterials. 2000;21(17):1803–1810.
- Sun H, Ye F, Wang J, et al. The upregulation of osteoblast marker genes in mesenchymal stem cells prove the osteoinductivity of hydroxyapatite/tricalcium phosphate biomaterial. Transplant Proc. 2008;40(8):2645–2648.
- Balasundaram G, Webster TJ. HA coated magnetic nanoparticles for the treatment of osteoporosis. 2010;27(9).
- Honeywell M, Philips S, Vo K. Teriparatide for osteoporosis: a clinical review. Drug Forecast Citeseer. 2003;28(11):713–716.
- GEN [Internet]. c2017. New Rochelle (NY): genetic engineering & biotechnology news. Critical Pharmaceuticals, University of Nottingham to work on nano-enabled nasal spray for osteoporosis; 2012 Feb 16 [cited 2016 Nov 17]; [about 2 screens]. Available from: http://www.genengnews.com/gen-news-highlights/critical-pharmaceuticals-university-of-nottingham-to-work-on-nano-enabled-nasal-spray-for-osteoporosis/81246375/
- Huang S, Chen JC, Hsu CW, et al. Effects of nano calcium carbonate and nano calcium citrate on toxicity in ICR mice and on bone mineral density in an ovariectomized mice model. Nanotechnology. 2009; [cited 2017 Mar 29];20(37):375102. DOI: 10.1088/0957-4484/20/37/375102
- Tokudome Y, Ito A, Otsuka M. Effect of zinc-containing β-tricalcium phosphate nano particles injection on jawbone mineral density and mechanical strength of osteoporosis model rats. Biol Pharm Bull. 2011;34(8):1215–1218.
- Kumar A, Gupta GK, Khedgikar V, et al. In vivo efficacy studies of layer-by-layer nano-matrix bearing kaempferol for the conditions of osteoporosis: a study in ovariectomized rat model. Eur J Pharm Biopharm. 2012;82(3):508–517.
- Sharan K, Siddiqui JA, Swarnkar G, et al. Role of phytochemicals in the prevention of menopausal bone loss: evidence from in vitro and in vivo, human interventional and pharmacokinetic studies. Curr Med Chem. 2009;16(9):1138–1157.
- Siddiqui J, Sharan K, Swarnkar G, et al. Quercetin-6-C-β-D-glucopyranoside isolated from Ulmus wallichiana planchon is more potent than quercetin in inhibiting osteoclastogenesis and mitigating ovariectomy-induced bone loss in rats. Menopause. 2011;18(2):198–207.
- Trivedi R, Kumar S, Kumar A, et al. Kaempferol has osteogenic effect in ovariectomized adult Sprague-Dawley rats. Mol Cell Endocrinol. 2008;289(1–2):85–93.
- Arjmandi BH, Birnbaum R, Goyal NV, et al. Bone-sparing effect of soy protein in ovarian hormone-deficient rats is related to its isoflavone content. Am J Clin Nutr. 1998;68(6 Suppl):1364S–1368S.
- Kumar A, Singh AK, Gautam AK, et al. Identification of kaempferol-regulated proteins in rat calvarial osteoblasts during mineralization by proteomics. Proteomics. 2010;10(9):1730–1739.
- Powell JJ, Bruggraber SFA, Faria N, et al. A nano-disperse ferritin-core mimetic that efficiently corrects anemia without luminal iron redox activity. Nanomedicine Nanotechnology, Biol Med. 2014;10(7):1529–1538.
- Prochorova AM, Pavlovb GV, Okpattah GAC. Nano-disperse iron for the treatment and prevention of iron-deficiency anemia in weaning pigs. Paper presented at: 10th Foresight Conference on Molecular Nanotechnology [Internet]. 2002 Oct 11–12. Bethesda, MD. [cited 2016 Nov 17]. Available from:https://www.foresight.org/Conference/MNT10/Abstracts/Prochorov2/index.html
- XCodex Foundation [Internet]. Heemstede (Netherlands): XCodex Foundation; c2017. Welcome to the next generation of mineral waters; 2016. [cited 2016 Nov 17]; [about 3 screens]. Available from: http://nano-mineralwater.com/nano-iron/
- Consumer Products Inventory. ColloidaLife™ Trace Minerals [Internet]. The project on emerging nanotechnologies. 2014. cited [2016 Nov 18]. Available from: http://www.nanotechproject.org/cpi/products/colloidalife-trace-minerals/
- Consumer Products Inventory. Nanogel [Internet]. The project on emerging nanotechnologies. 2013. cited [2016 Nov 18]. Available from: http://www.nanotechproject.org/cpi/products/nanogel/
- Consumer Products Inventory. Rejuvenate [Internet]. The project on emerging nanotechnologies. 2013. cited [2016 Nov 18]. Available from: http://www.nanotechproject.org/cpi/products/rejuvenate/
- Uswatta SP, Okeke IU, Jayasuriya AC. Injectable porous nano-hydroxyapatite/chitosan/tripolyphosphate scaffolds with improved compressive strength for bone regeneration. Mater Sci Eng C. 2016;69:505–512.
- Chen HS, Chang JH, Wu JSB. Calcium bioavailability of nanonized pearlpowder for adults. J Food Sci. 2008;73(9):H246–H251.
- Amini AR, Laurencin CT, Nukavarapu SP. Bone tissue engineering: recent advances and challenges. Crit Rev Biomed Eng. 2012;40(5):363–408.
- Petrovic V, Zivkovic P, Petrovic D, et al. Craniofacial bone tissue engineering. Oral Surg Oral Med Oral Pathol Oral Radiol Endod. 2012;114(3):e1–e9.
- Venkatesan J, Bhatnagar I, Manivasagan P, et al. Alginate composites for bone tissue engineering: a review. Int J Biol Macromol. 2015;72:269–281.
- Deepthi S, Venkatesan J, Kim S-K, et al. An overview of chitin or chitosan/nano ceramic composite scaffolds for bone tissue engineering. Int J Biol Macromol. 2016;93(Pt B):1338–1353.
- Mahoney C, McCullough BM, Bhattarai N, et al. Nanofibrous structure of chitosan for biomedical applications. J Nanomed Biotherap Discov. 2012; [cited 2017 Mar 29];2(1):102. DOI: 10.4172/2155-983X.1000102
- Zhao H, Jin H, Cai J. Preparation and characterization of nano-hydroxyapatite/chitosan composite with enhanced compressive strength by urease-catalyzed method. Mater Lett. 2014;116:293–295.
- Chang C, Peng N, He M, et al. Fabrication and properties of chitin/hydroxyapatite hybrid hydrogels as scaffold nano-materials. Carbohydr Polym. 2013;91(1):7–13.
- Manjubala I, Scheler S, Bössert J, et al. Mineralisation of chitosan scaffolds with nano-apatite formation by double diffusion technique. Acta Biomater. 2006;2(1):75–84.
- Xing Ma, Yang Wang, et al. Nano-hydroxyapatite/chitosan sponge-like biocomposite for repairing of rat calvarial critical-sized bone defect. J Bioact Compat Polym. 2011;26(4):335–346.
- Kong LJ, Gao Y, Lu GY, et al. A study on the bioactivity of chitosan/nano-hydroxyapatite composite scaffolds for bone tissue engineering. Eur Polym J. 2006;42(12):3171–3179.
- Zou Q, Li Y, Zhang L, et al. Characterization and cytocompatibility of nano-hydroxyapatite/chitosan bone cement with the addition of calcium salts. J Biomed Mater Res - Part B Appl Biomater. 2009;90(B1):156–164.
- Siripireddy B, Mandal BK, Shivendu R, et al. Nano-zirconia – Evaluation of its antioxidant and anticancer activity. J Photochem Photobiol B Biol. 2017;170:125–133.
- Janardan S, Suman P, Ragul G, et al. Assessment on antibacterial activity of nanosized silica derived from hypercoordinated silicon(IV) precursors guidelines to the referees. RSC Adv. 2016;6:66394–66406.
- Shivendu R, Nandita D, Srivastava P, et al. A Spectroscopic study on interaction between bovine serum albumin and titanium dioxide nanoparticle synthesized from microwave-assisted hybrid chemical approach. J Photochem Photobiol B Biol. 2016;161:472–481.
- Ranjan S, Nanditha D, Chinnappan S, et al. Titanium dioxide nanoparticle-protein interaction explained by docking approach. Int J Nano. Forthcoming 2017;
- Ranjan S, Dasgupta N, Chinnappan S, et al. A novel approach to evaluate titanium dioxide nanoparticle-protein interaction through docking: an insight into mechanism of action. Proc Natl Acad Sci India. 2015; cited [2016 Nov 20]. Available from:https://link.springer.com/article/10.1007/s40011-015-0673-z
- Ranjan S, Ramalingam C. Titanium dioxide nanoparticles induce bacterial membrane rupture by reactive oxygen species generation. Environ Chem Lett. 2016;14(4):487–494.
- Wei G, Ma PX. Structure and properties of nano-hydroxyapatite/polymer composite scaffolds for bone tissue engineering. Biomaterials. 2004;25(19):4749–4757.
- Sharma C, Dinda AK, Potdar PD, et al. Fabrication and characterization of novel nano-biocomposite scaffold of chitosan–gelatin–alginate–hydroxyapatite for bone tissue engineering. Mater Sci Eng C. 2016;64:416–427.
- Young AT, Kang JH, Kang DJ, et al. Interaction of stem cells with nano hydroxyapatite-fucoidan bionanocomposites for bone tissue regeneration. Int J Biol Macromol. 2016;93(Pt B):1488–1491.
- Sowjanya JA, Singh J, Mohita T, et al. Biocomposite scaffolds containing chitosan/alginate/nano-silica for bone tissue engineering. Colloids Surfaces B Biointerfaces. 2013;109:294–300.
- Correia CO, Leite ÁJ, Mano JF. Chitosan/bioactive glass nanoparticles scaffolds with shape memory properties. Carbohydr Polym. 2015;123:39–45.
- Teimouri A, Ebrahimi R, Emadi R, et al. Nano-composite of silk fibroin–chitosan/Nano ZrO2 for tissue engineering applications: fabrication and morphology. Int J Biol Macromol. 2015;76:292–302.
- Wers E, Oudadesse H, Lefeuvre B, et al. Evaluation of the kinetic and relaxation time of gentamicin sulfate released from hybrid biomaterial Bioglass-chitosan scaffolds. Appl Surf Sci. 2015;353:200–208.
- Kavya KC, Jayakumar R, Nair S, et al. Fabrication and characterization of chitosan/gelatin/nSiO2 composite scaffold for bone tissue engineering. Int J Biol Macromol. 2013;59:255–263.
- Saravanan S, Sameera DK, Moorthi A, et al. Chitosan scaffolds containing chicken feather keratin nanoparticles for bone tissue engineering. Int J Biol Macromol. 2013;62:481–486.
- Serra IR, Fradique R, Vallejo MCS, et al. Production and characterization of chitosan/gelatin/β-TCP scaffolds for improved bone tissue regeneration. Mater Sci Eng C. 2015;55:592–604.
- Shokri S, Movahedi B, Rafieinia M, et al. A new approach to fabrication of Cs/BG/CNT nanocomposite scaffold towards bone tissue engineering and evaluation of its properties. Appl Surf Sci. 2015;357(Part):1758–1764.
- Venkatesan J, Ryu B, Sudha PN, et al. Preparation and characterization of chitosan–carbon nanotube scaffolds for bone tissue engineering. Int J Biol Macromol. 2012;50(2):393–402.
- Pourhaghgouy M, Zamanian A, Shahrezaee M, et al. Physicochemical properties and bioactivity of freeze-cast chitosan nanocomposite scaffolds reinforced with bioactive glass. Mater Sci Eng C. 2016;58:180–186.
- Nazemi K, Azadpour P, Moztarzadeh F, et al. Tissue-engineered chitosan/bioactive glass bone scaffolds integrated with {PLGA} nanoparticles: a therapeutic design for on-demand drug delivery. Mater Lett. 2015;138:16–20.
- Jayakumar R, Ramachandran R, Kumar PTS, et al. Fabrication of chitin–chitosan/nano ZrO2 composite scaffolds for tissue engineering applications. Int J Biol Macromol. 2011;49(3):274–280.
- Heidari F, Bahrololoom ME, Vashaee D, et al. In situ preparation of iron oxide nanoparticles in natural hydroxyapatite/chitosan matrix for bone tissue engineering application. Ceram Int. 2015;41(2, Part B):3094–3100.
- Tripathi A, Saravanan S, Pattnaik S, et al. Bio-composite scaffolds containing chitosan/nano-hydroxyapatite/nano-copper–zinc for bone tissue engineering. Int J Biol Macromol. 2012;50(1):294–299.
- Fricain JC, Schlaubitz S, Visage C Le, et al. A nano-hydroxyapatite – Pullulan/dextran polysaccharide composite macroporous material for bone tissue engineering. Biomaterials. 2013;34(12):2947–2959.
- Shakir M, Jolly R, Khan MS, et al. Nano-hydroxyapatite/β-CD/chitosan nanocomposite for potential applications in bone tissue engineering. Int J Biol Macromol. 2016;93(Part A):276–289.
- Lowe B, Venkatesan J, Anil S, et al. Preparation and characterization of chitosan-natural nano hydroxyapatite-fucoidan nanocomposites for bone tissue engineering. Int J Biol Macromol. 2016;93(Pt B):1479–1487.
- Durgalakshmi D, Subhathirai SP, Balakumar S. Nano-bioglass: a versatile antidote for bone tissue engineering problems. Procedia Eng. 2014;92:2–8.
- Dasgupta N, Ranjan S, Rajendran B, et al. Thermal co-reduction approach to vary size of silver nanoparticle: its microbial and cellular toxicology. Env Sci Pol Res. 2016;23:4149–4163.
- Ranjan S, Dasgupta N, Rajendran B, et al. Microwave-irradiation-assisted hybrid chemical approach for titanium dioxide nanoparticle synthesis: microbial and cytotoxicological evaluation. Env Sci Pollut Res. 2016;23(12):12287–12302.
- Dasgupta N, Ramalingam C. Silver nanoparticle antimicrobial activity explained by membrane rupture and reactive oxygen generation. Environ Chem Lett. 2016;14(4):477–485.
- Sireesh BM, Mandal BK, Ranjan S, et al. Diastase assisted green synthesis of size- controllable gold nanoparticles. RSC Adv. 2015;5:26727–26733.
- Babu Maddinedi S, Kumar Mandal B, Patil SH, et al. Diastase induced green synthesis of bilayered reduced graphene oxide and its decoration with gold nanoparticles. J Photochem Photobiol B. 2017;166:252–258.
- Tammina SK, Mandal BK, Ranjan S, et al. Cytotoxicity study of Piper nigrum seed mediated synthesized SnO2 nanoparticles towards colorectal (HCT116) and lung cancer (A549) cell lines. J Photochem Photobiol B Biol. 2017;166:158–168.
- Sanhai WR, Sakamoto JH, Canady R, et al. Seven challenges for nanomedicine. Nat Nanotechnol. 2008;3(5):242–244.
- Bamrungsap S, Zhao Z, Chen T, et al. Nanotechnology in therapeutics: a focus on nanoparticles as a drug delivery system. Nanomed Future Med. 2012;7(8):1253–1271.
- Sahoo KB, Pattajoshi SP. Challenges of nano drug delivery and its safety issues. Int J Pharm Pharm Res. 2016; [cited 2017 Mar 29];6(3): Available from:http://ijppr.humanjournals.com/wp-content/uploads/2016/07/42.Bijay-Kumar-Sahoo-Sidheswar-Prasad-Pattajoshi.pdf
- Hobson DW, Roberts SM, Shvedova AA, et al. Applied Nanotoxicology. Int J Toxicol. 2016;35(1):5–16.
- Jain A, Shivendu R, Nandita D, et al. Nanomaterials in Food and Agriculture: an overview on their safety concerns and regulatory issues. Crit Rev Food Sci Nutr. Forthcoming 2017; DOI: 10.1080/10408398.2016.1160363
- Kisin ER, Murray AR, Keane MJ, et al. Single-walled carbon nanotubes: geno- and cytotoxic effects in lung fibroblast V79 cells. J Toxicol Environ Health A. 2007;70:2071–2079.
- Barnes CA, Elsaesser A, Arkusz J, et al. Reproducible comet assay of amorphous silica nanoparticles detects no genotoxicity. Nano Lett. 2008;8:3069–3074.
- Deng ZJ, Liang M, Monteiro M, et al. Nanoparticle-induced unfolding of fibrinogen promotes Mac-1 receptor activation and inflammation. Nat Nanotechnol. 2011;6(1):39–44.