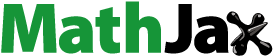
ABSTRACT
The commonly used methods to produce diosgenin by acid hydrolysis generate large amounts of wastewater and other pollution. Exploring a new environmentally friendly method is becoming urgent. Mild conditions and less pollution are the advantages of biotransformation. Therefore, aiming at exploring the effect of probiotic Lactobacillus on the production of diosgenin via biotransformation, batch studies were performed. Twenty-four strains maintained in laboratory were screened by conversion rate and pH. Based on screening, purification and 16 s rDNA sequences, four probiotic strains were identified and classified. The results indicated that all the strains had biotransformation potential. Among all the strains, four strains, including L20, L35, L83 and L33, performed better, with conversion rates reaching up to 15.12%, 15.83%, 17.07% and 17.47%, respectively. The highest conversion rate, 17.47%, was that of L33 and the lowest one, 7.07%, of L4. Nineteen of the 24 strains achieved more than 10% conversion rate. The pH decreased from 7 to 3–4 after fermentation, reflecting the progress of fermentation and indicating the end of the biotransformation process. Molecular characterization based on 16S rDNA homology of partial sequences confirmed the preliminary identification as L. rhamnosus (L20, L35), L. paracasei (L83) and L. salivarius (L33).
Abbreviations | ||
RSM | = | response surface methodology |
CCD | = | Central–Composite design |
DZW | = | Dioscorea zingiberensis Wright |
Introduction
Dioscorea zingiberensis Wright, also known as yellow ginger, is a perennial herbaceous plant rich in bioactive components such as saponins [Citation1,Citation2], alkaloids, flavonoid glycosides and cardiac glycosides. Moreover, it is the main source of diosgenin [Citation3]. Diosgenin exists in plants as a saponin precursor and is linked to the cell wall through a covalent bond with a sugar moiety at C3 position. Disogenin is an important pharmaceutical chemical. It is used as a raw material in the synthesis of steroid prophylactic agents and steroid hormones [Citation4], and is also widely used in cosmetics, health care, the medicine of contraception, analgesia, anesthesia etc. The method of sulphuric acid hydrolysis is commonly used in the production of diosgenin for its convenience and simplicity. However, large quantities of wastewater are produced, with high chemical oxygen demand (COD) and high concentrations of inorganic acid and SO42−, which makes the wastewater difficult to treat. The pollution from diosgenin production restricts the further development of diosgenin industry, which is a major source of income for local farmers. Against this background, exploring a new method with lower pollution and higher efficiency is of particular importance.
Saponins are converted to diosgenin when a glucosidic bond is broken through physical, chemical or biological methods. Thus, several studies have been performed to solve the associated problems [Citation5–7], most of which focus on the pretreatment of raw materials. An eco-friendly process of diosgenin production through physical separation and multi-enzyme hydrolysis has been established [Citation8]. Proper pretreatment can efficiently reduce the energy consumption and wastewater production [Citation9]. A reduction of the wastewater discharge has been accomplished by the method of double separation. This method removes the fibre and starch from D. zingiberensis efficiently and consumes less sulphuric acid. However, it does not completely solve the problem associated with the pollution resulting from the acid hydrolysis.
Thus, the methods of enzymatic hydrolysis have become a preferable choice for the mild reaction conditions [Citation10]. However, the high costs of enzymes, coupled with the product inhibition effect, restrict its further application in diosgenin production. As known, micro-organisms can secrete enzymes rapidly in response to the ambient environment; meanwhile, the product inhibition effects could be ameliorated through metabolic processes. In other words, the biotransformation method, which has lower costs, instead of commercial enzyme hydrolysis, emerges as a favourable method for diosgenin production. For example, removing starch from the raw material showed a significant effect on the biotransformation by Trichoderma reesei, with fewer pollutants detected during the process of biotransformation [Citation11]. Compared to the traditional sulphuric acid method, Trichoderma harzianum also performed well in producing diosgenin. Meanwhile, Aspergillus oryzae has been demonstrated to have considerable biotransformation potential [Citation12]. The results from diosgenin production through mould show that glycosidases produced by micro-organisms could efficiently cut the glycosidic bond, thereby converting saponins into diosgenin. As known, most moulds are harmful and the control of mould during large-scale production is not easy. Therefore, bacteria, especially probiotic strains, are believed to be more suitable for industrial production.
Inspired by former studies on producing diosgenin through biotransformation as a more environmentally-friendly method of diosgenin production [Citation13], we studied the conversion of saponins to diosgenin by means of 24 probiotic Lactobacillus strains [Citation14] isolated from commercial probiotic dairy products. The functions of probiotics, such as production of antihypertensive peptides, and their application in nutritional health food products have been explored in our previous research [Citation15–19]. Employing probiotics in biotransformation could also broaden the scope of application of strains. Biotransformation is mainly affected by the following factors. The utilization of carbon sources by D. zingiberensis directly affects the growth of strains, which is considered as a prerequisite for biotransformation. Enzymes secreted as part of the metabolism of probiotic Lactobacillus strains could effectively convert saponin to diosgenin and are hence considered as the tool for biotransformation [Citation20]. Lactic acid secreted during fermentation will restrict the growth and biotransformation. Hence, the conversion rate and pH were selected as the indicators for screening the strains with higher potential to convert saponin to diosgenin. Based on the screening results, the strains that performed better in the biotransformation process were isolated and identified based on 16 s rDNA sequences for the purpose of proper recognition and accurate classification of the strains.
Materials and methods
Micro-organism and culture conditions
Twenty-four Lactobacillus isolates were maintained in the collection of the School of Food and Biological Engineering, Shaanxi University of Science and Technology. All the strains were isolated from commercial dairy products and had been characterized in our relevant preliminary experiments. The strains were cultured in MRS (De Man, Rogosa and Sharpe) broth three times each for 24 h at 37 °C to obtain the active bacteria. The inoculum size for the cultures was 5%.
Preparation of D. zingiberensis fermentation broth
The D. zingiberensis fermentation broth was prepared by double separation of the D. zingiberensis biomass. First, fresh D. zingiberensis biomass was mixed with water and then milled thoroughly more than three times. Second, most of the cellulose was removed by gauze filtration, and after standing for 30 min the filtrate was divided into three layers. Third, the supernatant and the starch in the upper and lower layer were removed. The suspension in the middle layer was collected and was further purified by centrifugation at 3000 r/min for 15 min to meet the fermentation requirements. Fourthly, the fermentation began with inoculation of 5% (v/v) activated Lactobacillus strains into the D. zingiberensis suspension at 37 °C. The pH was adjusted to neutral before inoculation for better fermentation results. The pH of fermented D. zingiberensis supernatant was measured by a pH-meter (pHS-3C Shanghai Precision Scientific Instrument Co, Ltd, Shanghai, China).
Extraction of saponins and diosgenin
Saponins were extracted based on their unique solubility in water-saturated butanol. Saturated butanol was added to the suspension at twice the volume, followed by extraction for 2 h. The aqueous phase was removed by rotary evaporation at 50 °C until the saponin suspension turned into extract. Similarly, diosgenin was resolved in petroleum ether. The fermentation broth was dried in an oven and was subjected to Soxhlet-extraction for 2 h. Diosgenin solution was obtained through further concentration.
Measurement of the saponin and diosgenin content
The saponins contained in the sample were dissolved in 99.5% methanol in a 1:2 ratio. The saponin content was measured by the methanol and concentrated sulphuric acid method. Briefly, 0.6 mL of each sample was added to a colourimetric tube and the samples were dried in a water bath at 70 °C for 2 min. Then, 5 mL of methanol-sulphuric acid (V/V, 7:3) solution were added to the colourimetric tube. Following heating in a water bath for 1 h at 65 °C and cooling, the absorbance was measured at 329 nm (UV-5300 PC, Shanghai Metash Instruments Co., Ltd, Shanghai, China) against a blank control.
The method of vanillin-acetic acid was used to measure the diosgenin content. The diosgenin fraction extracted from the fermentation broth was dissolved in petroleum ether. Firstly, 1 mL of each sample was added to a colourimetric tube and the sample solution was dried in a water bath at 75 °C for 2 min. Next, 0.2 mL 5% (w/v) vanillin-acetic acid solution and 0.8 mL perchloric acid were added to the colourimetric tube one after another. After the thorough colour reaction of chromogenic reagent in a thermostatic water bath at 75 °C for 15 min, the colorimetric tube was cooled down to room temperature quickly. Then, acetic acid solution was added to the cooled tube up to 10 mL and the samples were left standing for 30 min before measurement of absorbance at 450 nm against a blank control.
DNA extraction and amplification of 16S rDNA sequences
A pure colony of each probiotic strain was picked and lysed in 50 μL TaKaRa Lysis Buffer (Cat. No. 9164) [Citation21]. After denaturation at 80 °C for 15 min, the supernatant was used as a template after centrifugation (14,000g). The amplification of the target fragment was performed by a TaKaRa 16S rDNA Bacterial Identification PCR Kit (Cat. No. RR176). The target DNA was amplified by polymerase chain reaction (PCR) using ‘Forward primer/Reverse primer2’, which could be utilized for amplification of full sequence DNA fragments. The reaction system and reaction conditions are described in .
Table 1. PCR reaction conditions.
Purification of PCR products
The amplified 16S rDNA fragments were purified by using a Takara MiniBEST Agarose Gel DNA Extraction Kit Ver.4.0 (Cat. no. 9762) according to the manufacturer's instructions. DL 2,000 DNA Maker (including 2000, 1000, 750, 500, 250 and 100 bp fragments) was used in the electrophoresis.
DNA sequencing and construction of phylogenetic tree
Primer of Seq forward, Seq reverse and Seq internal primers () were used for the sequencing of the 16S rDNA gene fragments. The sequencing was performed by ABI PRISM™ 33730XL DNA Sequence (Applied Biosystem). To construct phylogenetic trees, the sequences were analysed by the NCBI (National Center of Biotechnology Information) BLAST (basic local alignment search tool) and the evolutionary history was inferred using the neighbour-joining method with the unweighted pair group method with arithmetic-mean (UPGMA) [Citation22] among the 16S rDNA sequences of the strains (MEGA 7.0). The bootstrap consensus tree inferred from 1000 replicates is taken to represent the evolutionary history of the taxa analysed. The evolutionary distances were computed using the maximum composite likelihood method.
Table 2. Sequencing primers.
Calculation of conversion rate
The conversion rate was calculated by the following formula:where A is the saponin content after the biotransformation and B is the saponin content before the biotransformation. The saponin content after the biotransformation was calculated based on the content of diosgenin after the biotransformation, because the saponin content changed during the period of fermentation.
Results and discussion
Effect of probiotic Lactobacillus strains on biotransformation of D. zingiberensis saponins
Several experiments were conducted to screen the strains for potential for efficient biotransformation. After 48-h fermentation, the conversion rate and pH were determined ( and ). All the assayed probiotic Lactobacillus strains showed biotransformation potential. Among all the strains, 18 strains gave a conversion rate of more than 10%; these were L10, L28, LD, L35, L20, L5, L6, L9, LA, L49, L61, L54, L83, L21, L33, L60, L69 and L24. Notably, the conversion rate of strains L20, L35, L83 and L33 was about 15% or higher: 15.12%, 15.83%, 17.07% and 17.47%, respectively. Lower conversion rates were observed for strains LB, L41, L48, L57, L4 and LC (9.84%, 9.35%, 7.16%, 9.76%, 6.83% and 9.72%), indicating a certain ability of converting saponin to diosgenin. The different performance of various strains during biotransformation was highly dependent on the inherent factors by their own. The fermentation conditions were also vital for better conversion. The optimal fermentation parameters including time, inoculum size and temperature had been explored in our initial experiments. Thus, change of pH was considered being the main cause for distinct results. The pH value dynamics reflected the status of growth and fermentation during the biotransformation process. After 48 h of fermentation, the pH of the broth decreased to a range from 3.66 to 4.35, indicating the end of the fermentation process. The decline in pH could be attributed to acid released by probiotics during the fermentation, and more acid released into the broth indicated that the strains were more active during fermentation, with the conversion rate being higher in general. At the same time, more enzyme secreted could promote the process of biotransformation, which could be considered the main cause for obtaining better results. Property of acid tolerance under stress conditions could also have promoted the fermentation by different strains to some extent.
Phylogenetic analysis by 16S rDNA analysis
The agarose gel electrophoresis results () showed that all amplicons had the expected size and, after sequencing, the lengths obtained were beyond 1000 bp: 1484 bp (L20), 1476 bp (L33), 1484 bp (L35) and 1484 bp (L83) (Figure 1S, Online supplemental data). After amplification and purification of the target fragment, molecular characterization based on 16S rDNA homology of partial sequences (L20, L35, L83 and L33) with the sequences available in the NCBI database confirmed the preliminary identification as L. rhamnosus (L20, L35), L. paracasei (L83) and L. salivarius (L33). To trace the evolutionary patterns of the studied isolates based on sequence similarities, a phylogenetic tree was constructed using the neighbour-joining method with UPGMA among the 16S rDNA sequences of the strains and corresponding sequences of different probiotic Lactobacillus isolates in the NCBI database [Citation23]. As shown in , the resulting trees showed strain L20 had 100% homology with L. rhamnosus NBRC 3425 and L. rhamnosus JCM 1136. Just like L20, L35 had the same homology relationship with L. rhamnosus NBRC 3425 and L. rhamnosus JCM 1136. Similarly, 69% homology with L. salivarius JCM 1231 was shown in the tree of L33 and both had 100% homology with L. salivarius HO 66,99% homology was found for strain L83 with L. paracasei strain ATCC 25 302, L. paracasei strain R094, L. paracasei strain NBRC 15 906 and L. paracasei strain NBRC 15 889. Overall, the four strains selected for further study due to their high biotransformation potential were verified as L. rhamnosus (L20, L35), L. paracasei (L83) and L. salivarius (L33) via molecular phylogenetic analysis. Based on these results, clear recognition of strains was obtained via reclassification.
Figure 3. Agarose gel electrophoresis of PCR products from genomic DNA isolated from representative probiotic strains.
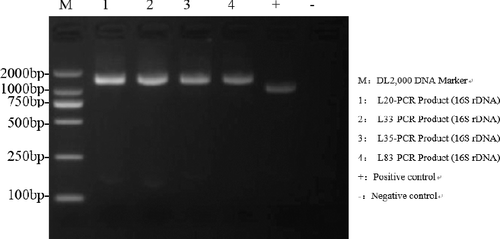
Figure 4. Phylogenetic tree of concatenated Lactobacillus strain L20 (A), L35 (B), L83 (C) and L33 (D) constructed by the neighbour-joining method with Kimura 2-parameter as evolution model and based on 16S rDNA nucleotide sequences.
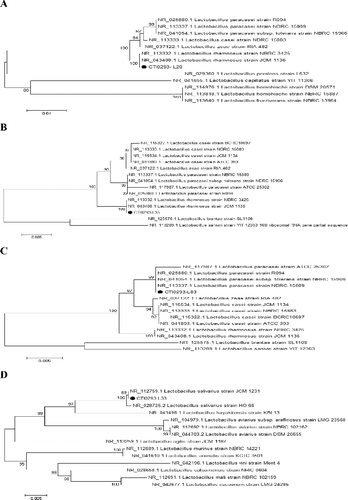
The obtained results, thus, indicate that the studied probiotic strains have high diosgenin-production potential. L. salivarius (L33) in this study showed the highest conversion rate of 17.47%, which was close to the value reported for Aspergillus oryzae as the fermentation strain [Citation24]. Based on the results for strain screening and identification, further analysis was performed to understand the reason why the four strains performed better during biotransformation. Ability to utilize glucose, fructose or cellobiose as a carbon resource for fermentation was found in a previous study of L. rhamnosus [Citation25]. Because of the utilization of various carbon resources for growth and fermentation, a higher conversion rate was obtained using L. rhamnosus. Furthermore, better acid tolerance of L. rhamnosus was inferred to be the main cause for the higher conversion rate in this study. Thus, it could be speculated that better biotransformation results were obtained due to good strain growth and production of more extracellular enzymes, owing to utilization of multiple carbon resources and tolerance to lactic acid. The utilization of various carbon sources and the multiple enzymes system promoted the process of biotransformation via L. paracasei [Citation26]. In L. salivarius, the characteristic of utilizing fructo-oligosaccharides and glucose during metabolism coupled with lactic-acid tolerance could be considered as propitious for the process of biotransformation [Citation27]. These characteristics could be considered as part of the factors behind the higher results achieved in biotransformation via L. rhamnosus (L20, L35), L.paracasei (L83) and L.salivarius (L33).
The results from this study could lay the basis for designing a more environmentally-friendly method for diosgenin production by eliminating the acid hydrolysis step. Moreover, utilizing biotransformation for diosgenin production would efficiently reduce the costs, since the method is less time- and energy-consuming, making it a feasible option in industrialized production. Thus, the biotransformation approach could provide a means of overcoming the major factors that limit the development of the diosgenin-production industry. What is more, it would consequently promote the development of local farmers who make a living from planting D. zingiberensis. However, further research is needed for optimizing the proposed method towards larger-scale production of diosgenin from D. zingiberensis biomass by biotransformation using L. rhamnosus (L20, L35), L. paracasei (L83) or L. salivarius (L33).
Conclusions
The results from this study showed that all the 24 assayed strains had some capacity for biotransformation of D. zingiberensis saponins into diosgenin. Among the 24 strains, L33 had the highest biotransformation capacity, with a conversion rate of 17.47%. Four of the strains, L20, L35, L83 and L33 had a conversion rate of more than 15%, i.e. 15.12%, 15.83%, 17.07% and 17.47% respectively. Furthermore, molecular characterization based on 16S rDNA homology of partial sequences (L20, L35, L83 and L33) with the sequences available in the NCBI database confirmed the preliminary identification as L. rhamnosus (L20, L35), L. paracasei (L83) and L. salivarius (L33). The results from this study could serve as a starting point for future studies aimed at introducing a more environmentally-friendly and cost-effective method for large-scale diosgenin production.
Supplementary_Data.pdf
Download PDF (368.7 KB)Disclosure statement
No potential conflict of interest was reported by the authors.
Additional information
Funding
References
- Chen YQ, Fan JF, Luo ZX, et al. Rapid clonal propagation of Dioscorea zingiberensis. Plant Cell Tiss Org Cult. 2003;73(1):75–80.
- Wang Y, Li X, Sun H, et al. Biotransformation of steroidal saponins in sisal (Agave sisalana Perrine) to tigogenin by a newly isolated strain from a karst area of Guilin, China. Biotechnol Biotechnol Equip. 2014;28(6):1024–1033.
- Wang Y, Liu H, Bao J, et al. The saccharification–membrane retrieval–hydrolysis (SMRH) process: a novel approach for cleaner production of diosgenin derived from Dioscorea zingiberensis. J Cleaner Prod. 2008;16(10):1133–1137.
- Oncina R, Botía JM, Río JAD, et al. Bioproduction of diosgenin in callus cultures of Trigonella foenum-graecum, L. Food Chem. 2000;70(4):489–492.
- Peng C, Zhao H, Zhao B, et al. Pilot treatment of wastewater from Dioscorea zingiberen sis C.H. Wright, production by anaerobic digestion combined with a biological aerated filter. Bioresour Technol. 2009;100(12):2918–2925.
- Cheng Y, Dong C, Huang C, et al. Enhanced production of diosgenin from Dioscorea zingiberensis in mixed culture solid state fermentation with Trichoderma reesei and Aspergillus fumigatus. Biotechnol Biotechnol Equip. 2015;29(4):773–778.
- Cheng Y, Hu S, Li T, et al. Production of diosgenin from Dioscorea zingiberensis with mixed culture in a new tray bioreactor. Biotechnol Biotechnol Equip. 2016;30(1):158–164.
- Huang W, Zhao HZ, Ni JR, et al. The best utilization of D. zingiberensis C. H. Wright by an eco-friendly process. Bioresour Technol. 2008;99(15):7407–7411.
- Qiu L, Niu H, Huang W. Ultrasonic and fermented pretreatment technology for diosgenin production from Diosorea zingiberensis, C.H. Wright. Chem Eng Res Des. 2011;89(2):239–247.
- Huang H, Zhao M, Lu L, et al. Pathways of biotransformation of zingiberen new saponin from Dioscorea zingiberensis, C. H. Wright to diosgenin. J Mol Cat B Enzymatic. 2013;98(98):1–7.
- Zhu YL, Wen H, Ni JR, et al. Production of diosgenin from Dioscorea zingiberensis, tubers through enzymatic saccharification and microbial transformation. Appl Microbiol Biotechnol. 2010;85(5):1409–1416.
- Lei J, Niu H, Li T, et al. A novel β-glucosidase from Aspergillus fumigates releases diosgenin from spirostanosides of Dioscorea zingiberens is C. H. Wright (DZW). World J Microbiol Biotechnol. 2012;28(3):1309–1314.
- Zhu Y, Huang W, Ni J. A promising clean process for production of diosgenin from Dioscorea zingiberensis, C. H. Wright. J Cleaner Prod. 2010;18(3):242–247.
- Ji Z. Screening and optimization of Lactobacillus sp. fermenting goat milk for production ACE-inhibitory peptides [master's thesis]. Xi'an: Shaanxi University of Science & Technology; 2013.
- Chen H, Bao C, Shu G, et al. Response surface methodology for optimizing fermentation conditions of goat yogurt with Bifidobacterium bifidum and Lactobacillus casei. Emirates J Food Agric. 2016;28(8):547–553.
- Lei N, Shu GW, Cao JL, et al. Extraction and antioxidant activities assay of polysaccharides from white hyacinth bean and promoting growth to probiotics. The Annals of the University Dunarea de Jos of Galati, Fascicle VI: Food Technol. 2016;40(2):51–62.
- Shu GW, Niu JF, Wan HC, et al. Investigations on the capacity of lactic acid bacteria to produce ace inhibitory peptides. The Annals of the University Dunarea de Jos of Galati, Fascicle VI: Food Technol. 2016;40(1):43–57.
- Chen H, Hui Y, Chen L, et al. Effect of probiotic Lactobacillus strains on antioxidant activity from fermented goat milk. Carpathian J Food Sci Technol. 2015;7(2):109–114.
- Chen H, Zhang JH, Shu GW. Screening of probiotic goat milk tablets using Plackett-Burman design. Acta Sci Pol Technol Aliment. 2014;13(4):351–358.
- Adham NZ, Zaki RA, Naim N. Microbial transformation of diosgenin and its precursor furostanol glycosides. World J Microbiol Biotechnol. 2009;25(3):481–487.
- Goranov B, Denkova R, Teneva D, et al. Molecular-genetic identification of Lactobacillus strains, isolated from homemade yoghurt. Ukrainian Food J. 2015;4(1):67–76.
- Sokal RR. A statistical method of evaluating systematic relationships. Univ Kansas Sci Bull. 1958;38:1409–1438.
- Sun G, Yao T, Feng C, et al. Identification and biocontrol potential of antagonistic bacteria strains against Sclerotinia sclerotiorum, and their growth-promoting effects on Brassica napus. Biol Control. 2017;104:35–43.
- Dong YS, Shan-Shan QI, Lin L, et al. Direct biotransformation of Dioscorea zingiberensi s C.H. Wright by Aspergillus oryzae to produce diosgenin. Chinese J Process Eng. 2009;9(5):993–998.
- Reale A, Renzo TD, Rossi F, et al. Tolerance of Lactobacillus casei, Lactobacillus paracasei and Lactobacillus rhamnosus, strains to stress factors encountered in food processing and in the gastro-intestinal tract. LWT – Food Sci Technol. 2015;60(2):721–728.
- Petrova P, Velikova P, Popova L, et al. Direct conversion of chicory flour into L (+)-lactic acid by the highly effective inulinase producer Lactobacillus paracasei DSM 23505. Bioresour Technol. 2015;186:329–333.
- Gao Y, Yu HL, Zhou YQ, et al. Metabolism of fructooligosaccharides by Lactobacillus salivairus. China Brewing. 2010;29(12):114–117.