Abstract
Calcium-dependent protein kinase (CDPK) plays an important role in plant resistance to disease. In this study, we assessed CDPK activity in Hami melons during Penicillium infection, in order to investigate the possible role of CDPK in this context. The results showed the induction of CDPK by Penicillium infection may contribute to the early stages of disease resistance in Hami melons. In order to further study the molecular mechanisms underlying this response, relatively high expression of HmCDPK1 was screened form the transcriptome database of Hami melons. Bioinformatics analysis showed HmCDPK1 had a length of 2365 bp, with a maximum open reading frame of 1560 nucleotides, encoding 519 amino acids with a molecular weight of 58,646.83. Moreover, its theoretical isoelectric point was 6.34, as a hydrophilic and non-transmembrane protein. Real-time quantitative PCR showed the transcript of HmCDPK1 was persistently increased after Penicillium infection and reached its maximum at 12 h, being significantly higher than in non-infected plants. These results suggest the induction of CDPK activity and HmCDPK1 expression in the early response of Hami melons to Penicillium may contribute to the resistance against this infection.
Introduction
Hami melon (Cucumis melo) is a popular fruit in Xinjiang, and an important cash crop in this locality [Citation1]. However, factors such as infections and mechanical damage lead to serious postharvest diseases every year, severely affecting sales and postharvest storage of Hami melons and resulting in large economic losses. Among these pathogens, Penicillium is the major source of postharvest deterioration and decay in Hami melons under cold storage conditions [Citation2, Citation3]. Certain treatments have been found to improve disease resistance in Hami melons. For example, treatment with acibenzolar-S-methyl (ASM) before harvest appears to enhance the activity of defense-related enzymes in melons, effectively reducing fungal infection and postharvest deterioration rates [Citation4]. On the other hand, hot water and shellac treatment can inhibit the incidence of Fusarium and Alternaria infection in Hami melons fruits, and increase the activity of defensive enzymes in some fruits [Citation5]. Moreover, the growth of Alternaria and semi-solid Fusarium on the surface of melon can be inhibited by a combination of pimaricin with a bilayer membrane composed of chitosan and polyethylene wax [Citation6]. Although many studies are available regarding disease resistance in postharvest melons, research on Penicillium-infected Hami melons remains limited.
Calcium-dependent protein kinase (CDPK) is a serine/threonine protein kinase involved in multiple aspects of plant development and plant response to biotic and abiotic stress signal transduction [Citation7–9]. CDPK is a member of the single-peptide chain family of enzymes, featuring a N-terminal variable domain, a kinase domain, a self-suppressing domain and a calmodulin-like domain [Citation10]. CDPK has been shown to exist only in plants and some protozoa, and not in animals [Citation11–13]. CDPK-like genes have also been found in monocotyledons, green algae, apical complex parasites and ciliates [Citation14–16]. The CDPK gene is widely expressed in different plant organs, such as roots, stems, flowers, fruits and seeds [Citation17–19], and its encoding protein is also abundantly found in the plasma membrane, nucleus, chromatin, cytoskeleton, chloroplasts and mitochondria [Citation20–24]. CDPK is extensively involved in stress responses in plants. Increased expression of AtCPK1 in Arabidopsis thaliana has been found to confer broad-spectrum resistance to bacteria and fungi [Citation25]. OsCPK12 promotes salt tolerance by reducing the accumulation of reactive oxygen species (ROS) [Citation26]. ZmCPK4 and OsCPK9 can improve drought tolerance by regulating stomatal closure during drought stress [Citation14, Citation27], whereas overexpressing AtCDPK1 in potatoes plants significantly enhanced the resistance to drought and osmotic stresses [Citation28]. Various VpCDPKs such as VpCDPK6, VpCDPK9, VpCDPK14, VpCDPK16 and VpCDPK19 play an active role in the plant response to powdery mildew fungus [Citation29]. Although the role of CDPK in disease resistance has been extensively studied in many plant species, reports on Hami melons remain limited.
In this study, CDPK activity was assessed in Penicillium-infected Hami melons. Moreover, HmCDPK1 was evaluated through bioinformatics analysis, revealing the transcript pattern in response to Penicillium infection. These results will provide important information for understanding the response of Hami melons to Penicillium infection at physiological and molecular levels, which would aid in its storage and preservation.
Materials and methods
Experimental samples and treatments
The Penicillium strain used in this study was isolated from Hami melons surface in previous study by our lab. The Penicillium strain was grown in the potato medium (2% glucose, 2% agar and 20% potato) and then cultivated at 28 °C until a large quantity of spores were produced. The Penicillium spores were harvested by using sterile water flushing and the spores suspensions were diluted with sterile water to obtain 1 × 105 spores/mL dilutions.
Hami melons used in the experiment were of the Jiashigua variety, obtained from a farm in the 121 regiment of Shihezi, Xinjiang. Fruits with a harvesting maturity of 80%, uniform size, and no disease, pests, or mechanical damage were harvested and transported to the warehouse of the Food College of the Shihezi University within 12 h. Thirty fresh and healthy Hami melons of equal size were selected and dried after washing with clean water, and then disinfected with 70% alcohol. At room temperature, a sterile perforator was used to punch 6 holes of 1 cm in diameter and 1 cm in depth on each specimen of Hami melons. Then, the 3 holes on the left of each Hami melons were infused with 10 µL sterile distilled water, while the 3 holes on the right were infused with 10 µL of a suspension with 1 × 105 spores/mL of Penicillium (). Then, samples were obtained from the left and right holes at 0, 12, 18, 24, 30, 36, 42, 48, 54, 60, 66, 72, 78 and 84 h after infection, and three Hami melons were collected from each treatment group and repeated in triplicates. Approximately 5 g of plant tissue was collected around each hole in a centrifugal tube using a sterilized perforator and a blade. This tissue was quickly frozen in liquid nitrogen and transferred to a refrigerator at −80 °C for subsequent analysis.
Assay of enzyme activity
CDPK activity was evaluated using an enzyme-linked immuno-sorbent assay (ELISA) Kit (Quanzhou Conodi Biotechnology Co., Ltd.), according to the manufacturer’s instructions. Firstly, plant protein was extracted and diluted 5-fold according to instructions. Then, 50 µL of control, standard and experimental samples were added to the enzyme-coated plate without touching the hole wall. After gentle mixing and sealing the plate with a film, the plate was incubated at 37 °C for 30 min. After discarding the liquid, all holes were washed 5 times with washing solution. Next, 50 µL of enzyme-labelled reagent was added to each pore (except the blank pore) for incubation and washing. Thereafter, 50 µL of colour rending A and 50 µL of colour rending B solution were added and incubated at 37 °C for 15 min. Finally, 50 µL of termination solution was added to end the reaction, with the blue colour turning to yellow immediately. After adjusting to zero by the blank hole, the absorbance of each hole was measured at a 450 nm wavelength within 15 min of adding the termination solution.
Screening and identification of HmCDPKs
Construction of the transcriptome library of Penicillium infected with Hami melons (at 0, 48 and 60 h) was performed using the Illumina HiSeq 2000 high throughput sequencing platform. Differentially expressed genes at different times after infection (0, 48 and 60 h) were obtained by RNA-seq. In total, 31 differentially expressed HmCDPKs were identified in this study. After combining the information from the differential expression of these genes at different times and the changing folds, Unigene 13609_QHMG, the gene with the highest expression and the largest changing fold, was chosen for further analysis.
Sequence analysis and bioinformatics analysis of HmCDPK1
The open reading frame (ORF) of HmCDPK1 was analyzed using ORF Finder software; then, the amino acid sequence was inferred. The physical and chemical properties of HmCDPK1 were analyzed by multiple software packs, including ProtParam, ProtScale, Signal 3.0 Server, SOPMA and TMHMM Server v.2.0. The subcellular localisation of HmCDPK1 was predicted using ProtComp v. 9.0, whereas the amino acid sequence was analyzed by SOPMA and SWISS-MODEL to construct models of protein secondary and tertiary models. After Blast analysis of the amino acid sequences encoded by the largest ORF, its sequence and similar sequences of other plants were used to construct a phylogenetic tree using MAGE 6.0.
Total RNA extraction and reverse transcription of cDNA
Total RNA was extracted using a column type of total RNA extraction and purification kit (SK8661), according to manufacturer’s instructions. In general, experimental samples and control samples at 0, 6, 12, 18, 24, 30, 36, 42, 48, 54, 60, 66, 72, 78 and 84 h of treatment were used for the extraction of total RNA. For the reverse transcription of cDNA, a 14.5 μL mixture with 1 μl dNTP Mix and 1 μl of random primer and RNA were incubated at 65 °C for 5 min, then ice incubated for 2 min, and finally centrifuged for 3–5 s. Next, at 4 °C, 4 μL of 5× RT buffer, 0.5 μL of Thermo Scientific RiboLock RNase Inhibitor (20 U) and 1 μL RevertAid Premium Reverse Transcriptase (200 U) were added, and the mixture was incubated at 25 °C for 10 min, at 50 °C for 30 min and at 85 °C for 5 min. Thereafter, the cDNA solution was stored at −20 °C for further analysis.
Real time fluorescence quantitative PCR analysis
The transcript of HmCDPK1 in Hami melons fruit was analyzed using LightCycler 480 Software Setup (Roche), according to manufacturer’s instructions. Different DNA samples were used for PCR amplification with three repeats. The primers were designed and synthesized by Bioengineering (Shanghai) Co., Ltd. The primers of HmCDPK1 and the internal reference gene HmGAPDH were CDPK1-F-CGAGTGGTTCGTTCTACA, CDPK1-R-GCATACTTTCCGTTGCC; GAPDH-F: CTTTCCGTGTTCCTACCGTT, GAPDH-R-CAGTGTACCCCAATTCCC, respectively. The PCR reaction system included 40 nM of primers, 10 µL of SybrGreen qPCR Master Mix and cDNA. PCR amplification was set as denaturation at 95 °C for 3 min, 45 cycles of denaturation at 95 °C for 7 s, annealing at 57 °C for 10 s and elongation at 72 °C for 12 s. Amplification was carried out in a plate with 96 or 384 pores, and the SybrGreen fluorescence signal was detected at the end of elongation at 72 °C. All qPCR were standardized by the corresponding CT value of the reference gene, and the relative expression of target genes was calculated by the 2−(ΔΔCt) method with the average value of each biological repeat.
Statistical analysis of data
Transcriptome data was analyzed using Microsoft EXCEL, while figures were rendered using Origin 8.5. Differences between samples were analyzed with SPSS v. 17.0.
Results and discussion
Sequence analysis of HmCDPK1
Based on the database of RNA-seq, 31 HmCDPKs showed differential expression at different infection times (0, 48 and 60 h). Among these genes, Unigene 13609_QHMG displayed the highest expression and the largest changing fold, and was chosen for further analysis. The gene had a length of 2365 bp, with a maximum ORF of 1560 nucleotides based on ORF Finder software, encoding 519 amino acids. Blast analysis of conserved sequences found a N-terminal variable region, a kinase region, a junction region and four conserved calcium-binding regions (EF-hand), showing typical CDPK characteristics (), which is in agreement with the findings of Liese et al. [Citation30] and Fedorowicz et al. [Citation31]. Therefore, this gene was identified as HmCDPK1 and the NCBI submission number was XP_004152931.1.
Prediction of the physical and chemical properties of HmCDPK1 protein
The physical and chemical properties of HmCDPK1 were analyzed with ProtParam in ExPASy. The HmCDPK1 encoded protein had 519 amino acids, with a molecular weight of 58,646.83, a theoretical isoelectric point of 6.34, and a fat index of 77.11 (). In addition, the average hydrophilicity coefficient and the instability index of HmCDPK1 protein were −0.505 and 44.09, respectively (), indicating that it may be a hydrophilic and unstable protein. Analysis with ProtComp v.9.0 for the subcellular localisation of HmCDPK1 suggested that it may be located in the plasma membrane. At the cellular level, the plasma membrane acts as an important hub, sensing external stress signals and initiating a series of regulatory events on gene expression in plant cells [Citation32, Citation33]. As an important component of the cell membrane, plasma membrane proteins play essential roles in coping with biotic and abiotic stress, as well as cellular osmotic regulation [Citation34, Citation35]. The subcellular localization of HmCDPK1 was also consistent with the predicting analysis of signal peptides, as well as that of other plant CDPK.
Table 1. Prediction of the physical and chemical properties of HmCDPK1.
Prediction and analysis of the secondary and tertiary structure of the HmCDPK1 protein
The secondary structure of the HmCDPK1 coded protein was predicted with SOPMA software, with its highest ratio of a-helices being 246 amino acids, accounting for 47.39% of the total amino acids. Besides a-helices, HmCDPK1 had 148, 66 and 59 irregular coiled amino acids, 66 extended-chain amino acids and 66 β-corner amino acids, accounting for 28.52%, 12.72% and 11.37% of the total amino acids, respectively (). In addition, the tertiary structure of the HmCDPK1 protein was analyzed and constructed with SWISS-MODEL software. Results showed the prediction of a-helices, irregular coils, β-corners and extended chains were generally consistent with that of the secondary structure. Moreover, the highest structural similarity was determined between HmCDPK1 and 4IEB_A of Toxoplasma gondii CDPK in the PDB database, at 42.15% ().
Construction of the phylogenetic tree of HmCDPK1
Multiple homologous proteins of HmCDPK1 were obtained with Blast software using amino acid sequences, including Cucumis sativus XM_004152883.2, C. melo XM_008465191.2, Vitis vinifer XM_002278143.4, Vitis amurensis KC488319.1, Citrus clementina XM_006452308.1, Theobroma cacao XM_018125646.1 and Aegilops tauschii XM_020324118.1, with similarities of 98%, 95%, 82%, 81%, 81%, 72% and 73%, respectively. Analysis of the phylogenetic tree of these proteins showed the amino acid sequence of HmCDPK1 belonged to the same branch as cucumber and both of them formed a branch with melon, which had close homology with V. amurensis, but less homology with cocoa (). Thus, the coding region of HmCDPK1 appears to be conserved in long-term biological evolution, and may play an important role in the evolution of various plant species.
Effects of Penicillium infection on HmCDPK activity and the transcript of HmCDPK1
In order to further investigate the possible role of HmCDPKs in the response of Hami melons to Penicillium infection, the protein activity and gene expression of HmCDPK1 were assessed. Under control conditions, the activity of HmCDPK increased gradually and reached its maximum at 12 h. Thereafter, it declined rapidly, only increasing slightly from 48 to 60 h (). After infection with Penicillium, HmCDPK activity displayed a similar trend to that of control, yet with higher levels (). Consistently, the levels of HmCDPK1 transcript were higher in Penicillium-infected plants than in controls, peaking at 12 h, and then rapidly decreasing, with a slight increase at 42 to 60 h (). Thus, in response to Penicillium infection, plant CDPK appears to be induced and activated to regulate cell adaptation and resistance to the adverse environment. With increasing time under stress, the cell structure and signalling pathways may be disrupted, affecting normal cell metabolism and leading to a decline of enzymatic activity. In this context, HmCDPK may be an important protein in the early stages of resistance against Penicillium infection in Hami melons. The early response of HmCDPK to Penicillium infection is also consistent with previous studies by Allwood et al. [Citation36] and Chico et al. [Citation37].
Figure 6. Expression of HmCDPK in response to Penicillium infection at different time points. (A) CDPK activity in Hami melons infected by Penicillium infection and controls. (B) Levels of HmCDPK1 transcript in Hami melons infected by Penicillium infection and controls. Note: Error bars represent the standard deviation of the means.
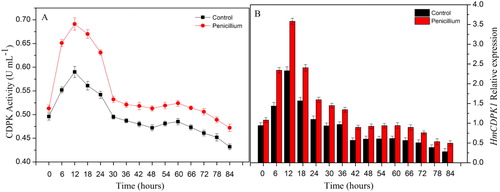
Conclusions
Our findings indicate the induction of CDPK activity and HmCDPK1 expression in the early response of Hami melons to Penicillium infection may contribute to the resistance against this infection during early storage. The bioinformatics analysis of HmCDPK1 and the change patterns we report, regarding its expression and its underlying enzyme activity, will provide important information for further understanding and research on the storage and preservation of Penicillium-infected Hami melons.
Disclosure statement
No potential conflict of interest was reported by the authors.
Additional information
Funding
References
- Ming N, Fengxian T, Qin Z, et al. The quality of Gold Queen Hami melons stored under different temperatures. Sci Hortic. 2019;243:140–147.
- Liu T, Tong JM, Ma WR, et al. Separation and identification of main pathogen in Hami melon during cold storage. Mod Food Sci Technol. 2013;29:2030–2034.
- Chen CK, Wang WS, Ning J, et al. Isolation and identification of the main pathogenic fungi from postharvest Hami melon in later period of storage. Adv Mater Res. 2011;343-344:1212–1216.
- Zhang Z, Bi Y, Ge Y, et al. Multiple pre-harvest treatments with acibenzolar-S-methyl reduce latent infection and induce resistance in muskmelon fruit. Sci Hortic. 2011;130:126–132.
- Zhou R, Wang X, Hu Y, et al. Reduction in Hami melon (Cucumis melo var. saccharinus) softening caused by transport vibration by using hot water and shellac coating. Postharvest Biol Technol. 2015;110:214–223.
- Cong F, Zhang Y, Dong W. Use of surface coatings with natamycin to improve the storability of Hami melon at ambient temperature. Postharvest Biol Technol. 2007;46:71–75.
- Hamel LP, Sheen J, Séguin A. Ancient signals: comparative genomics of green plant CDPKs. Trends Plant Sci. 2014;19:79–89.
- Romeis T, Herde M. From local to global: CDPKs in systemic defense signaling upon microbial and herbivore attack. Curr Opin Plant Biol. 2014;20:1–10.
- Simeunovic A, Mair A, Wurzinger B, et al. Know where your clients are: subcellular localization and targets of calcium-dependent protein kinases. J Exp Bot. 2016;67:3855–3872.
- Satterlee JS, Sussman MR. Unusual membrane-associated protein kinases in higher plants. J Membr Biol. 1998;164:205–213.
- Harmon AC, Gribskov M, Gubrium E, et al. The CDPK superfamily of protein kinases. New Phytol. 2001;151:175–183.
- Valmonte GR, Arthur K, Higgins CM, et al. Calcium-dependent protein kinases in plants: evolution, expression and function. Plant Cell Physiol. 2014;55:551–569.
- Subhashree R, Rajani KM. In silico analysis of plasmodium falciparum CDPK5 protein through molecular modeling, docking and dynamics. J Theor Biol. 2019;461:254–267.
- Li AL, Zhu YF, Tan XM, et al. Evolutionary and functional study of the CDPK gene family in wheat (Triticum aestivum L.). Plant Mol Biol. 2008;66:429–443.
- Ma PD, Liu JY, Yang XD, et al. Genome-wide identification of the maize calcium-dependent protein kinase gene family. Appl Biochem Biotechnol. 2013;169:2111–2120.
- Zhang XS, Choi JH. Molecular evolution of calmodulin-like domain protein kinases (CDPKs) in plants and protists. J Mol Evol. 2001;53:214–224.
- Liu H, Che Z, Zeng X, et al. Genome-wide analysis of calcium-dependent protein kinases and their expression patterns in response to herbivore and wounding stresses in soybean. Funct Integr Genomics. 2016;16:481–493.
- Ray S, Agarwal P, Arora R, et al. Expression analysis of calcium-dependent protein kinase gene family during reproductive development and abiotic stress conditions in rice (Oryza sativa L. ssp. indica). Mol Genet Genomics. 2007;278:493–505.
- Gromadka R, Cieśla J, Olszak K, et al. Genome-wide analysis and expression profiling of calcium-dependent protein kinases in potato (Solanum tuberosum). Plant Growth Regul. 2018;84:303–315.
- Lu SX, Hrabak EM. An Arabidopsis calcium-dependent protein kinase is associated with the endoplasmic reticulum. Plant Physiol. 2002;128:1008–1021.
- Myers C, Romanowsky SM, Barron YD, et al. Calcium-dependent protein kinases regulate polarized tip growth in pollen tubes. Plant J Cell Mol Biol. 2009;59:528–539.
- Chehab EW, Patharkar OR, Hegeman AD, et al. Autophosphorylation and subcellular localization dynamics of a salt- and water deficit-induced calcium-dependent protein kinase from ice plant. Plant Physiol. 2004;135:1430–1446.
- Dammann C, Ichida A, Hong B, et al. Subcellular targeting of nine calcium-dependent protein kinase isoforms from Arabidopsis. Plant Physiol. 2003;132:1840–1848.
- Zhang H, Liu WZ, Zhang Y, et al. Identification expression and interaction analyses of calcium-dependent protein kinase (CPK) genes in canola (Brassica napus L.). BMC Genomics. 2014;15:211.
- Coca M, San Segundo B. AtCPK1 calcium-dependent protein kinase mediates pathogen resistance in Arabidopsis. Plant J. 2010;63:526–540.
- Asano T, Hayashi N, Kobayashi M, et al. A rice calcium-dependent protein kinase OsCPK12 oppositely modulates salt-stress tolerance and blast disease resistance. Plant J. 2012;69:26–36.
- Wei S, Hu W, Deng X, et al. A rice calcium-dependent protein kinase OsCPK9 positively regulates drought stress tolerance and spikelet fertility. BMC Plant Biol. 2014;14:133.
- Nie LZ, Yu XX, Ma YH, et al. Enhanced drought and osmotic stress tolerance in transgenic potato plants expressing AtCDPK1, a calcium-dependent protein kinase. Russ J Plant Physiol. 2018;65:865–873.
- Zhang K, Han YT, Zhao FL, et al. Genome-wide identification and expression analysis of the CDPK gene family in grape, Vitis spp. BMC Plant Biol. 2015;15:164.
- Liese A, Romeis T. Biochemical regulation of in vivo function of plant calcium-dependent protein kinases (CDPK). Biochim Biophys Acta. 2013;1833:1582–1589.
- Fedorowicz-Strońska O, Koczyk G, Kaczmarek M, et al. Genome-wide identification, characterisation and expression profiles of calcium-dependent protein kinase genes in barley (Hordeum vulgare L.). J Appl Genet. 2017;58:11–22.
- Hennig L. Plant gene regulation in response to abiotic stress. Biochim Biophys Acta. 2012;1819:85.
- Mizoi J, Shinozaki K, Yamaguchi-Shinozaki K. AP2/ERF family transcription factors in plant abiotic stress responses. Biochim Biophys Acta. 2012;1819:86–96.
- Alexandersson E, Saalbach G, Larsson C, et al. Arabidopsis plasma membrane proteomics identifies components of transport, signal transduction and membrane trafficking. Plant Cell Physiol. 2004;45:1543–1556.
- Hossain Z, Nouri MZ, Komatsu S. Plant cell organelle proteomics in response to abiotic stress. J Proteome Res. 2012;11:37–48.
- Allwood EG, Davies DR, Gerrish C, et al. Regulation of CDPKs, including identification of PAL kinase, in biotically stressed cells of French bean. Plant Mol Biol. 2002;49:533–544.
- Chico JM, Raices M, Tellez IM, et al. A calcium-dependent protein kinase is systemically induced upon wounding in tomato plants. Plant Physiol. 2002;128:256–270.