Abstract
β-poly(L-malic acid) (PMLA) is a highly water-soluble biopolymer used in medicine and other industries. PMLA is usually biosynthesized by Aureobasidium pullulans, but in low concentrations, which has restricted its further large-scale production. Here effects of surfactants on PMLA production were investigated. Of various surfactants examined, the addition of Tween 80 at the start of cell growth increased mycelial growth, PMLA production, PMLA productivity and the ratio of PMLA to cell biomass concentration (Yp/x) of A. pullulans. Transmission electron microscopy and flow cytometry showed that cell membrane permeability was increased, improving the secretion of PMLA and uptake of nutrients and oxygen. Proteomic analysis showed that key pathways of PMLA synthesis, along with cellular energy metabolism, were enhanced. Moreover, glutathione (GSH) synthesis and anti-oxidation capacity were also improved. These results indicated that the addition of Tween 80 is an efficient approach for improving the production of PMLA.
Introduction
Poly(β-L-malic acid) (PMLA) is a biopolymer that consists of repetitive L-malyl units linked by ester bonds between one carboxyl and one hydroxyl group of adjacent molecules of L-malic acid (MA) [Citation1,Citation2]. PMLA has outstanding features as biocompatibility, biodegradability, water solubility, absorbability, non-immunogenicity and easy chemical modification [Citation1]. Therefore, PMLA and its derivatives may have great potential for applications in the drug delivery field and the production of advanced biomaterials [Citation1,Citation3,Citation4].
Aureobasidium pullulans, a cosmopolitan yeast-like fungus, is the most commonly used microorganism for PMLA production [Citation5]. Recently, the production of PMLA has attracted wider attention because of the expanding number of potential applications for this biopolymer [Citation6]. To meet the increasing demand for PMLA, it has become necessary to improve the efficiency of PMLA production. Numerous approaches have been developed to improve PMLA production, including screening for high-yield mutant strains, investigating improvements to fermentation modes and strategies, optimisation of carbon sources, adding exogenous substances and control of agitation speed [Citation3,Citation7,Citation8]. In our study, we have focussed on developing methods to weaken the resistance of cells to the movement of substances into/out of them and to enhance PMLA production.
Polyoxyethylenesorbitan monooleate, generally known as Tween 80, is a non-ionic surfactant that contains a mono-unsaturated fatty acid (oleate). Tween 80 has been extensively employed as a vehicle for the addition of water insoluble compounds and is known to interfere with the permeability of cell membranes, enhancing nutritional input from the surroundings to the cell body [Citation9,Citation10]. Sheng et al. [Citation11] found that the addition of Tween 80 to culture medium significantly enhanced pullulan production, but had no impact on biomass accumulation. Xia [Citation12] found that the addition of Tween 80 to the culture medium increased the production of the polysaccharide curdlan and that this enhanced curdlan production linked to Tween 80 was highly correlated with glucosyl transferase activity. However, little information is available about the effect of Tween 80 on the production of PMLA by A. pullulans, as well as on certain other aspects of its biology, including its cell membrane morphology and cell metabolism. Until now, only Tu et al. [Citation5] reported that Tween 80 was beneficial for the biosynthesis of PMLA and pullulan and that it could regulate the ratio of PMLA to pullulan in a dose-dependent manner. In addition, we conducted a preliminary study on the mechanism behind the effect of Tween 80 on PMA and pullulan production.
In this study, we aimed to explore the mechanism by which the production of PMLA by A. pullulans is enhanced by Tween 80. To gain insights into how the mechanism works, we used transmission electron microscopy (TEM) and proteomic technology to analyse how the morphology of the cell membrane and key enzymes in A. pullulans metabolic pathways changed following the addition of Tween 80. The results will assist in finding a cost effective and feasible approach for producing high amounts of PMLA.
Materials and methods
Microorganisms and media
The PMLA-producing strain A. pullulans was deposited in the China General Microbiological Culture Collection Center (Storage No.: CGMCC3337). This strain was maintained on potato dextrose agar slants at 4 °C and subcultured every two weeks. The seed medium contained 140 g L−1 glucose, 3 g L−1 yeast extract, 2 g L−1 succinic acid, 0.4 g L−1 K2CO3, 0.1 g L−1 KH2PO4, 0.1 g L−1 MgSO4·7H2O, 0.005 g L−1 ZnSO4·7H2O and 20 g L−1 CaCO3. The fermentation medium contained 180 g L−1 sucrose, 35 g L−1 peptone, 0.1 g L−1 KH2PO4, 2 g L−1 NaNO3, 0.3 g L−1 MgSO4·7H2O, 0.5 g L−1 KCl, 0.05 g L−1 MnSO4 and 20 g L−1 CaCO3. The initial pH of the medium was adjusted to 7.0 and autoclaved at 121 °C for 15 min, then cooled to room temperature.
Culture conditions
The primary seed culture for A. pullulans was prepared by inoculating cells grown on solid medium into 500 mL Erlenmeyer flasks containing 100 mL seed culture medium and cultured at 25 °C for approximately 40 h in a rotary shaker (IS-RDS3, Crystal Technology & Industries, Inc., USA) at 200 rpm. To evaluate the effect of different surfactants (SDS, Triton X-100, Tween 60 and Tween 80) on PMLA production, different concentrations of surfactants were fed into 500 mL Erlenmeyer flasks containing 100 mL fermentation medium. The seed culture (10%, v/v) was inoculated into the fermentation medium and cultured at 25 °C for 6 days in a rotary shaker at 200 rpm. Batch fermentation kinetics were studied in a 5 L stirred-tank fermentor (GRJB-5D, Zhenjiang Gree Co. Ltd, China) containing 3 L fermentation medium. The fermentation medium was inoculated with 300 mL of seed culture grown in a shake flask at 25 °C, with an aeration rate of 1.2 vvm and agitation speed of 300 rpm for 40 h.
Analytical methods
Cell biomass and residual sugar concentration
The cell density was determined using the dry cell weight method. Before taking the measurement, excess CaCO3 in the broth was eliminated by the addition of 3 M HCl. The culture broth (10 mL) was centrifuged at 8000 g for 10 min. Precipitates were washed three times with phosphate buffered saline (PBS) (137 mmol·L−1 NaCl, 2.7 mmol·L−1KCl, 10 mmol·L−1 Na2HPO4, 1.76 mmol·L−1 KH2PO4, pH 7.4), then centrifuged again. The precipitates were subsequently dried at 105 °C until their weight remained constant. The fermentation broth was centrifuged for residual sugar analysis using the 3,5-dinitrosalicylic acid assay method [Citation13].
Assay of PMLA production
For analysis of PMLA production, the fermentation broth was centrifuged and 5 mL of the resulting supernatant was mixed with 5 mL 2 mol L−1 H2SO4 and incubated at 110 °C for 9 h. After neutralising the solution, the hydrolysed sample was analysed by HPLC (L-2000, Hitachi Ltd., Japan) for its malic acid content, using a Spursil C18-EP organic acid column eluted with 5 mmol·L−1 H2SO4 at 25 °C and a flow rate of 1 mL min−1.
PMLA productivity calculation
The rate of PMLA production was estimated from the ratio of the increased PMLA production versus interval time.
Yp/x calculation
The Yp/x was estimated from the ratio of increased PMLA to the increased cell biomass concentration over the interval time [Citation14].
Determination of cell membrane permeability
The effect of Tween 80 on cell membrane permeability of A. pullulans was investigated by determining changes in the fluorescence intensity of cells stained with propidium iodide (PI), using flow cytometry (FACSCalibur, Becton, Dickinson and Company, USA). The cells were stained with 100 mg of PI in 2 mL of PBS buffer at room temperature and stored in the dark for 30 min. Samples were then washed several times and changes in fluorescence intensity were examined by flow cytometry [Citation15].
Fatty acid methyl analysis
Cells harvested at the late-logarithmic phase were washed three times with PBS followed by centrifugation at 15,000 g for 5 min. In the first step, fatty acids were extracted by freeze-drying mycelial cells. In the second step, total lipids were extracted from 20 mg of freeze-dried sample according to Bligh’s method [Citation16]. Briefly, saponification was carried out with 2 mL of saponification reagent (7.5 mol L−1 NaOH:CH3OH, 1:1 v/v) at 100 °C for 30 min. For generation of fatty acid methyl esters (FAMEs), 4 mL of methylation reagent (CH3OH:6 N HCl, 1:1 v/v) was added to the saponified sample and incubated at 80 °C for 10 min. After the reaction, 2.5 mL of extraction reagent (hexane:methyltert-butyl ether, 1:1 v/v) was added and incubated for 10 min. The upper phase was recovered by centrifugation. Washing was performed with 6 mL of 0.5 mol·L−1 NaOH. The FAMEs analysis was conducted using an Agilent 7890 A gas chromatograph with a flame ionisation detector and capillary column (Agilent DB-225 10 m 0.1 mm 0.1 μm, Agilent Technologies Inc. USA) [Citation17]. Each FAME component was identified and quantified using Supelco® 37 Component FAME Mix (Sigma, USA).
TEM observation of cell membranes
The cell membranes of A. pullulans were observed using a transmission electron microscope (JEM-1200EX, JEOL Ltd., Japan) with a Gatan 830 CCD camera atan accelerating voltage of 80 kV. The sample was stained with both uranyl acetate and lead nitrate before observation by TEM. Gatan DigitalMicrograph (version 3.7) software was used to analyse the images. Samples were tested in triplicate and four images were obtained for each replication.
Proteome analysis
Protein extraction, digestion and iTRAQ
After 120 h culture, the fermentation broth (5 mL) was centrifuged at 5000 g for 30 s to remove unused CaCO3, and the supernatant was transferred to a new centrifuge tube. Cell pellets of A. pullulans were collected by centrifuging at 10,000 g for 2 min at 4 °C. The cell pellets were washed three times with PBS buffer, and then resuspended in PBS. Next, 300 μL lysate (7 mol·L−1 urea, 1.4 mol·L−1 thiourea, 4% CHAPS) and protease inhibitor were added. The samples were vortexed for 1 min and placed on ice for 60 s, which was repeated for a total of 6 cycles. The lysates were centrifuged at 14,000 g for 20 min at 4 °C, and the supernatants were collected. The concentration of extracted protein was measured using the Bradford protein assay [Citation18]. One hundred micrograms of each sample were added to 4 μL Recuding Reagent for 1 h at 60 °C, then 2 μL Cysteine-Blocking Reagent was added and the samples were left for 10 min at room temperature. A 10 K ultrafiltration device was used to fill the above solution at 12,000 g for 20 min, then the solution at the bottom was discarded. One hundred microlitres of Dissolution Buffer from the iTRAQ kit were added and the mixture was centrifuged at 12,000 g for 20 min; the solution at the bottom was discarded. This step was repeated three times. A new collection tube was used to mix 4 μg trypsin with the acquired solution, which was then incubated at 37 °C overnight. The enzymatically digested peptide was centrifuged at 12,000 g for 20 min. Then 50 μL Dissolution Buffer 5 centrifuged at 12,000 g for 20 min was combined with the above products, resulting in a 100 μL sample after digestion. The samples were labelled with iTRAQ reagents by adding the contents of the iTRAQ® Reagent-8Plex Multiplex Kit to the sample solutions (Applied Biosystems).
Protein separation and detection
Sample analysis was performed using a RIGOL L-3000 dual gradient HPLC (Puyuanjingdian Science and Technology Ltd., China) and Thermo Scientific EASY-nLC 1000 System (Nano-HPLC) to generate MS and tandem MS (MS/MS) data. The mixed labelled sample was dissolved in 100 μL of buffer A (98% double distilled H2O, 2% acetonitrile (pH 10)) which was then centrifuged at 14,000 g for 20 min and the supernatant was collected for further analysis. Peptides were loaded onto a Durashell-C18 column (DC952505-0, 4.6 mm × 250 mm, 5 μm, Agela Technologies Co. Ltd, China) and then subjected to mobile-phase elution in buffer A and buffer B (98% acetonitrile, 2% ddH2O (pH 10)) by the following protocol. The peptides were eluted at a flow rate of 0.7 mL min−1. Then, the acquired sample was re-dissolved with 20 μL 2% carbinol and 0.1% formic acid and centrifuged at 12,000 g for 10 min at room temperature; the supernatant was assimilated. The sample was loaded into an EASY-Spray column (12 cm × 75 μm, C18, 3 μm), and then eluted at a flow rate of 350 nL min−1 for 15 min at room temperature. The liquid chromatography eluent was directed to an electrospray ionisation source for quantitative time-of-flight MS analysis. Electrospray ionisation was performed for information-dependent acquisition in positive-ion mode with a spray voltage of 2.1 kV and a selected mass range of 350–1800 m/z. The Thermo Q-Exactive system was operated in data-dependent acquisition mode.
Analysis of protein mass spectrometry data
The MS/MS data were processed using Proteome Discoverer software (version 1.4) (Thermo Electron Co., USA) with default parameters to generate a peak list. The search parameters were as follows: trypsin as the digestion enzyme with allowance for a maximum of two missed cleavages; carbamido-methylation (C) as a static modification; Oxidation (M), N-Term K and iTRAQ 8Plex modification as a dynamic modification; precursor ion mass tolerance of 15 ppm; and fragment ion mass tolerance of 20 mmu.
Results and discussion
Effects of different surfactants on A. pullulans cell growth and PMLA production
The effects of different surfactants–sodium dodecyl sulphate (SDS), Triton X-100, Tween 60 and Tween 80, on A. pullulans cell growth and PMLA production are shown in . The results show that cell growth and PMLA production were significantly affected by adding different concentrations of Tween 60 and Tween 80, compared with the control. At 144 h, in the cultures grown with 2 g L−1 Tween 80, biomass and PMLA production reached 45.4 g L−1 and 31.36 g L−1, respectively, reflecting increases of 18.84% and 30.5% compared to the control. The biomass and PMLA production also showed increases of 11.85% and 20.86%, respectively, with the addition of 2 g L−1 Tween 60. However, biomass and PMLA production were strongly inhibited by the addition of SDS and Triton X-100. Previous results have shown that Tween 80 may be beneficial for PMLA biosynthesis at low concentrations [Citation5]. Therefore, the kinetics with the addition of 0.2% Tween 80 were further investigated in a 5 L stirred-tank fermentor.
Kinetics with the addition of 0.2%tween 80 in a 5 L stirred-tank fermentor
The comparison of the fermentation parameters of A. pullulans with and without the addition of 0.2% Tween 80 are shown in . PMLA formation during the first 96 h of fermentation following the addition of 0.2% Tween 80 increased faster compared to the control and the highest PMLA yield reached 32.11 g L−1, which was a 32.14% increase (). The biomass increased sharply during the first 96 h of fermentation with the addition of 0.2% Tween 80 and the highest biomass reached 44.85 g L−1, an increase of 16.91% compared to the control (). After 96 h of fermentation, the PMLA productivity and the ratio of PMLA to cell biomass concentration (Yp/x) was 0.32 g L−1·h and 1.52 g g−1 with the addition of 0.2% Tween 80 (), which were increases of 38.9% and 49%, respectively, compared to the control. Meanwhile, the rate of residual sugar consumption increased during the first 96 h of fermentation in the cultures with 0.2% Tween 80 (), and residual sugar was completely consumed at the end of fermentation in the 0.2% Tween 80 group. These results support previous findings [Citation5] that Tween 80 is beneficial for A. pullulans cell growth and PMLA biosynthesis at low concentrations. We speculate that Tween 80 may interfere with the permeability of cell membranes and, by doing so, enhances nutritional inputs from the surroundings to the cell body [Citation9,Citation19]. Adding Tween 80 may, therefore, be a feasible approach to adopt for producing greater amounts of PMLA. The following section, with the help of TEM and proteomic technology, will focus on the mechanism by which Tween 80 acts on PMLA production by A. pullulans.
Figure 2. Effects of Tween 80 on PMLA accumulation and cell growth during PMLA batch fermentation of A. pullulans in 5 L quadruple parallel fermentor: (a) PMLA concentration, (b) residual sugar concentration, (c) biomass, (d) PMLA productivity and (e) yield (the ratio of PMLA to cell biomass concentration). Note: Data represent mean values with standard deviation (±SD) from three replicates. If error bars are not visible, they are smaller than the symbol.
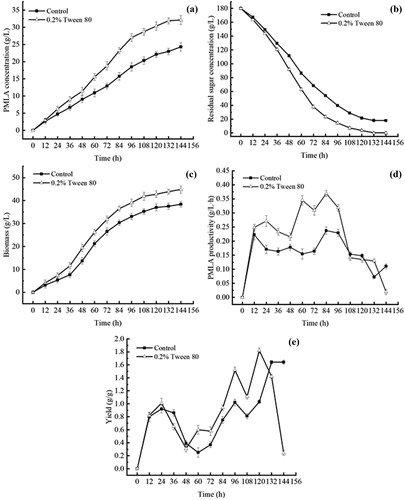
Effect of tween 80 on the cell membrane of A. pullulans
Effect of Tween 80 on cell membrane permeability of A. pullulans
Fatty acid profiles (% fatty acids) of A. pullulans cells are shown in . The results showed that the major fatty acids detected in A. pullulans cells were palmitic acid (C16:0), palmitoleic acid (C16:1), stearic acid (C18:0), oleic acid (C18:1n9c) and linoleic acid (C18:2n6c) () [Citation11]. With the addition of 0.2% Tween 80 the ratio of unsaturated/saturated fatty acids increased significantly, from 2.1% to 2.79%, which was an increase of 32.86% compared with the control. The change in the ratio of unsaturated/saturated fatty acids was mainly due to an increase in linoleic acid (C18:2n6c) with a concomitant decrease in palmitic acid (C16:0). This finding indicated that membrane permeability could be changed by the addition of Tween 80 [Citation10]. In addition, the unsaturated fatty acid levels in the membranes were consistent with an increase in their fluidity [Citation20,Citation21].
Table 1. Fatty acid composition of A. pullulans cells cultured with and without 2.0% Tween 80 for 120 h.
To further confirm the effect of Tween 80 on cell membrane permeability, changes in the fluorescence intensity of cells stained with PI was determined using flow cytometry (). PI is a nucleic acid dye that normally cannot pass through the cell membrane. However, when cell membrane permeability is altered, PI can cross the membrane and produce red fluorescence after binding to nucleic acids in the cell [Citation9,Citation22]. As shown in , with the addition of 0.2% Tween 80 the fluorescence intensity in A. pullulans cells increased significantly, from 8.74 to 12.19, an increase of 39.47% compared to the control. This increase in fluorescence intensity indicated that PI had permeated into A. pullulans cells across the cell membrane, further demonstrating that the addition of Tween 80 led to an increase in cell membrane permeability [Citation9].
Table 2. Effect of Tween 80 on cell membrane permeability of A. pullulans as assessed by flow cytometry.
These results showed that Tween 80 can interfere with the permeability of cell membranes, which might enhance cells’ ability to take up essential components from their surroundings, such as vitamins and oxygen [Citation9,Citation11,Citation19] and to release PMLA into the extracellular fluid.
Effect of Tween 80 on cell membrane morphology of A. pullulans
After the addition of 0.2% Tween 80, the cell membrane of A. pullulans was observed by TEM (). The cell membrane morphology of A. pullulans treated with 0.2% Tween 80 was clearly different from that of the control. The cell membrane of the control cells was regular, clear, round and compact, whereas the cell membrane of A. pullulans following treatment with 0.2% Tween 80 was irregular, loose, inwardly concave and folded. These observations indicated that Tween 80 damaged the cell membrane of A. pullulans to a certain extent [Citation9]. The morphological changes in the cell membrane of A. pullulans may have occurred because Tween 80 can interact with the lipid components of a membrane’s phospholipid bilayer and solubilize biomolecules [Citation9]. As seen in , the addition of Tween 80 indeed resulted in changes in the composition and content of unsaturated fatty acids in the cell membrane.
Proteome analysis of A. pullulans cultivated with the addition of 0.2% tween 80
According to the proteome analyses, compared to the control group, the expression of key enzymes in A. pullulans metabolic pathways was changed by the addition of 0.2% Tween 80 (; ). Twenty-three differentially expressed key proteins, including enzymes related to energy metabolism, glycolysis, the pentose phosphate pathway (PPP), cell self-rescue and cell defence, were identified with the addition of Tween 80 ().
Figure 4. Effects of adding Tween 80 on the metabolic pathway of A. pullulans. PMLA: Poly(β-L-malic acid); FMN: Flavin mononucleotide; FeS: iron-sulfur clusters; CoQ: Coenzyme Q; Cyt c: Cytochrome c.
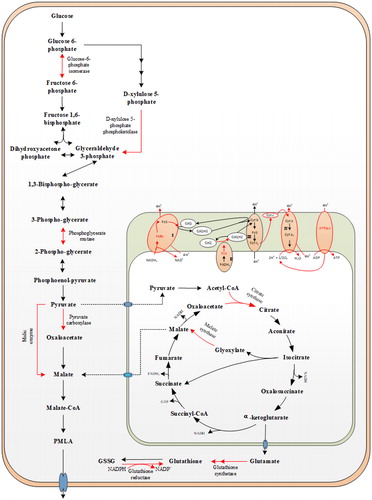
Table 3. Changes in protein expression after addition of Tween 80.
Analyses of differentially expressed proteins related to growth and PMLA production in A. pullulans
Expressions of proteins related to carbon metabolism and energy metabolism varied greatly with the addition of Tween 80. Expressions of glucose-6-phosphate isomerase, phosphoglycerate mutase and D-xylulose 5-phosphate phosphoketolase, which are key enzymes in glycolysis and the pentose phosphate pathway, were up-regulated by 1.528-, 1.615- and 1.239-folds, respectively (). Glucose-6-phosphate isomerase (GPI), also known as phosphoglucose isomerase, was identified as the second glycolytic enzyme, which catalyses the interconversion of glucose-6-phosphate to fructose-6-phosphate [Citation23,Citation24]. D-xylulose 5-phosphate phosphoketolase is a lyase, which uses inorganic phosphate (Pi) to split D-xylulose-5-phosphate into D-glyceraldehyde-3-phosphate and acetylphosphate [Citation25] and plays a central role in the PPP [Citation26]. Phosphoglycerate mutase (PGAM) is a protein critical for glycolysis [Citation27], which catalyses the conversion of 3-phosphoglycerate (3-PG) to produce 2-phosphoglycerate (2-PG). Our experimental results showed that adding Tween 80 could increase the expression of these key enzymes of glycolysis and PPP () and enhance the pathways of glycolysis and PPP (). These pathways not only generate carbon skeletons, maintaining high levels of glycolytic intermediates to support anabolic reactions in cells [Citation28], but also provide NADPH and NADH, which are required for the synthesis of GSH and adenosine triphosphate (ATP) [Citation29].
PMLA is a novel polymer composed of MA subunits linked by ester bonds. The biosynthesis of MA may involve three different pathways, including the oxidative tricarboxylic acid (TCA) cycle, the reductive TCA pathway and the glyoxylate shunt pathway [Citation30,Citation31]. Expressions of proteins related to PMLA synthesis metabolism varied greatly with the addition of Tween 80. Expressions of citrate synthase and malate synthase, which are key enzymes in the glyoxylate shunt pathway, were up-regulated by 1.205- and 1.264-folds, respectively (). Citrate synthase is an enzyme that this cycle has in common with the citrate cycle, while malate synthase, which catalyses the formation of MA from glyoxylate and acetyl-CoA, is an enzyme exclusive to the glyoxylate shunt pathway [Citation32]. These results showed that adding Tween 80 enhanced the glyoxylate shunt pathway, strengthened the metabolic flow of carbon in the glyoxylate shunt for PMA synthesis (). Meanwhile, the expressions of malic enzyme and pyruvate carboxylase were up-regulated by 1.201- and 1.200-folds, respectively (). Pyruvate carboxylase, which is a key enzyme in the reductive TCA pathway, catalyses the transformation of pyruvate into oxaloacetate by CO2-fixation, followed by the reduction of oxaloacetate to MA [Citation33]. These metabolic pathways for the production of MA from glucose were enhanced, and therefore PMLA synthesis was also enhanced with the addition of Tween 80.
Expressions of NADH-ubiquinone oxidoreductase, succinate dehydrogenase [ubiquinone] iron-sulphur subunit, cytochrome c hemelyase, cytochrome c oxidase (COX) and ATP synthase related to the respiratory chain were up-regulated by 1.595-, 1.202-, 1.250-, 1.828- and 1.200-folds, respectively, with the addition of Tween 80 (). NADH:ubiquinone oxidoreductase, respiratory complex I, is the main entry point for electrons from NADH into the respiratory chains of most mitochondria [Citation34]. Succinate dehydrogenase, respiratory complex II, is the only enzyme that is involved in both the TCA and in respiration via the electron transport chain [Citation35]. Cytochrome c hemelyase (CCHL) is an enzyme found in the mitochondria of many eukaryotes and is used for heterologous expression of mitochondrial holocytochrome c. Yeast CCHL has been shown to manufacture enough cytochrome c and cytochromec1 for cell survival in yeast [Citation36]. The principal physiological role of mitochondrial cytochrome c is electron transfer during oxidative phosphorylation [Citation29]. COX is the terminal enzyme of the mitochondrial respiratory chain, which catalyses the transfer of electrons from reduced cytochrome c to molecular oxygen [Citation37] and plays a crucial role in cellular respiration and energy generation [Citation38]. Mitochondrial ATP synthase is the enzyme responsible for the synthesis of more than 90% of the ATP produced by mammalian cells under aerobic conditions [Citation39]. Our experimental results showed that adding Tween 80 increased the expression of key enzymes in the respiratory chain and enhanced the pathways of the respiratory chain (). Oxidative phosphorylation (OXPHOS) was enhanced and more ATP was produced. Cells rely on both glycolysis and OXPHOS to produce ATP [Citation40]. Thus, the addition of Tween 80 enhanced cellular energy metabolism () and supported cellular energy demands, which are fundamental requirements for biological processes, such as cell proliferation and stress resistance [Citation41]. Meanwhile, the synthesis of PMLA requires the consumption of energy in the form of ATP. We speculate that the synthesis of PMLA very likely involves an enzyme complex consisting of a malyl-AMP ligase and a malyl-tranferase (polymerase), analogous to a non-ribosomal peptide synthetase, and that the activity of the malyl-AMP ligase requires ATP and Mg2+ ions [Citation1].
Our experimental results showed that carbon metabolism, PMLA synthesis metabolism and energy metabolism were all enhanced with the addition of Tween 80. We speculate that Tween 80 could interfere with the permeability of the cell membrane, which might enhance cellular uptake of essential components including glucose, vitamins and oxygen. An increased glucose uptake rate with the addition of Tween 80 could be beneficial for glucose metabolic pathways, such as glycolysis and PPP; our results showed that cell metabolic rates increased, as demonstrated by the increased rate of sugar consumption () and PMLA productivity (). These pathways not only generate carbon skeletons, which maintain high levels of glycolytic intermediates to support anabolic reactions in cells, but also provide NADPH and NADH, which are required for the synthesis of GSH and ATP. Adding Tween 80 strengthened the metabolic flow of carbon in the glyoxylate shunt and reductive TCA pathways for PMLA synthesis and thus, PMLA synthesis was increased. Moreover, the permeability of the cell membrane was enhanced, which could also be beneficial for releasing PMLA into the extracellular fluid. The level of oxygen uptake rate (OUR) is raised with the addition of Tween 80 [Citation5,Citation42], which is likely to enhance OXPHOS. As a result, cellular energy metabolism was intensified, which in turn supported cellular energy demands for cell proliferation, PMLA synthesis and stress resistance. Thus, the addition of Tween 80 could facilitate the growth and PMLA production of A. pullulans and improve cell survival under stress conditions.
Analysis of differentially expressed proteins related to cell self-rescue and defence
Expressions of proteins related to cell self-rescue and defense varied greatly with the addition of Tween 80. The expressions of catalase, glutathione reductase, glutathione S-transferase, glutathione synthetase and heat shock 70 kDa protein were up-regulated by 1.324-, 1.222-, 2.001-, 1.218- and 1.260-folds, respectively (). The expression of glutathione synthase was up-regulated () and synthesis pathway of GSH was enhanced (). Meanwhile, oxidized glutathione (GSSG) was reduced to glutathione (GSH) by glutathione reductase (). It is speculated that GSH, glutathione synthetase, glutathione reductase (GR) and NADPH comprise a GSH-dependent reduction system, which plays an important role in maintaining cell viability through intracellular red-ox equilibrium, in addition to protecting cells from low pH, halide, oxidative stress and osmotic stress [Citation29,Citation43], and assists in protein repair and DNA synthesis [Citation44]. The glutathione S-transferases (GSTs) are a family of multi-functional enzymes that play critical roles in the detoxification of xenobiotics and the protection of tissues against oxidative damage [Citation45]. Catalases detoxify H2O2 by reducing it to water and oxygen [Citation46]. The Hsp70 system is involved in the isolation and transfer of polypeptide chains from aggregates to the Hsp100 chaperone, which further unfolds them by translocation through its central channel. The concerted action of chaperones counteracts the aggregation process. This mechanism of protein quality control (together with the proteolytic machinery necessary for removing an excess of damaged proteins) significantly increases a cell’s chance of surviving stress conditions [Citation47]. Plasma membrane ATPase (PM H+-ATP-ase), a family of P-type ATPases, consist of a functional polypeptide with ten transmembrane domains and three cytosolic domains and play a pivotal role in guard cell physiology [Citation48].
Our experimental results showed that, compared to the control group, adding Tween 80 could promote the synthesis of GSH, which plays a critical role in the detoxification of xenobiotics and the protection of tissues against oxidative damage. The expressions of stress proteins with the addition of Tween 80 were up-regulated, which ensured correct protein folding under harsh environments. Therefore, adding Tween 80 could enhance the resistance of A. pullulans to environmental stresses and protect cells from oxidative stress.
Conclusions
In this study, the effects of surfactants on the production of PMLA by A. pullulans were investigated. Among the various surfactants examined, the addition of Tween 80 (2 g L−1) at the beginning of the cell growth stage was found to be favourable to mycelial growth and PMLA production. TEM and flow cytometry showed that cell membrane permeability was increased, and thus, improving the secretion of PMLA and the uptake of nutrients and oxygen. Proteomic analysis showed that the key pathways of PMLA synthesis (glycolysis, the glyoxylate shunt pathway and the reductive TCA pathway) were intensified. Furthermore, cellular energy metabolism was also improved, supporting increased cellular energy demands for cell proliferation, PMLA synthesis and stress resistance. Importantly, GSH synthesis and the capacity for anti-oxidation were raised, helping to protect cells from low pH, halide, oxidative stress and osmotic stress by maintaining the viability of cells through intracellular red-ox equilibrium. These research results will be helpful for finding a cost effective and feasible approach for producing larger quantities of PMLA.
Disclosure statement
The authors declare that they have no conflict of interest.
Funding
This work was supported by the Foundation of Tianjin Municipal Science and Technology Commission, PR China under grant numbers 17PTGCCX00190, 17PTSYJC00080, 17YFCZZC00310 and 16YFXTSF00460, the Foundation of Tianjin Engineering Research Center of Microbial Metabolism and Fermentation Process Control, PR China under grant number ZXKF20180301 and the Foundation of Engineering Research Center of Food Biotechnology, Ministry of Education, PR China under grant number SPZX10-18.
References
- Chi Z, Liu G-L, Liu C-G. Poly(β-l-malic acid) (PMLA) from Aureobasidium spp. and its current proceedings. Appl Microbiol Biotechnol. 2016;100:3841–3851.
- Li Y, Chi Z, Wang G-Y, et al. Taxonomy of Aureobasidium spp. and biosynthesis and regulation of their extracellular polymers. Crit Rev Microbiol. 2015;41:228–237.
- Cao W, Luo J, Qi B, et al. β-poly(l-malic acid) production by fed-batch culture of Aureobasidium pullulans ipe-1 with mixed sugars. Eng Life Sci. 2014;14:180–189.
- Ding H, Portilla-Arias J, Patil R, et al. The optimization of polymalic acid peptide copolymers for endosomolytic drug delivery. Biomaterials. 2011;32:5269–5278.
- Tu G, Wang Y, Ji Y, et al. The effect of Tween 80 on the polymalic acid and pullulan production by Aureobasidium pullulans CCTCC M2012223. World J Microbiol Biotechnol. 2015;31:219–226.
- Prajapati VD, Jani GK, Khanda SM. Pullulan: an exopolysaccharide and its various applications. Carbohydr Polym. 2013;95:540–549.
- Cao W, Luo J, Zhao J, et al. Intensification of β-poly(l-malic acid) production by Aureobasidium pullulans ipe-1 in the late exponential growth phase. J Ind Microbiol Biotechnol. 2012;39:1073–1080.
- Zhang H, Cai J, Dong J, et al. High-level production of poly (β-l-malic acid) with a new isolated Aureobasidium pullulans strain. Appl Microbiol Biotechnol. 2011;92:295–303.
- Liang Y, Zhu L, Gao M, et al. Influence of Tween-80 on the production and structure of water-insoluble curdlan from Agrobacterium sp. Int J Biol Macromol. 2018;106:611–619.
- Taoka Y, Nagano N, Okita Y, et al. Effect of Tween 80 on the growth, lipid accumulation and fatty acid composition of Thraustochytrium aureum ATCC 34304. J Biosci Bioeng. 2011;111:420–424.
- Sheng L, Zhu G, Tong Q. Mechanism study of Tween 80 enhancing the pullulan production by Aureobasidium pullulans. Carbohydr Polym. 2013;97:121–123.
- Xia Z. Effect of Tween 80 on the production of curdlan by Alcaligenes faecalis ATCC 31749. Carbohydr Polym. 2013;98:178–180.
- Breuil C, Saddler JN. Comparison of the 3,5-dinitrosalicylic acid and Nelson-Somogyi methods of assaying for reducing sugars and determining cellulase activity. Enz Microb Technol. 1985;7:327–332.
- Wang Y, Song X, Zhang Y, et al. Effects of nitrogen availability on polymalic acid biosynthesis in the yeast-like fungus Aureobasidium pullulans. Microb Cell Fact. 2016;15:146. [cited 2019 Jun 06]
- Ingrid BH. Flow cytometric analysis of the LE cell phenomenon. Autoimmunity. 2004;37:37–44.
- Bligh EG, Dyer WJ. A rapid method of total lipid extraction and purification. Can J Biochem Physiol. 1959;37:911–917.
- Shen Y, Liang J, Li H, et al. Hydroxypropyl-β-cyclodextrin-mediated alterations in cell permeability, lipid and protein profiles of steroid-transforming Arthrobacter simplex. Appl Microbiol Biotechnol. 2015;99:387–397.
- Bradford MM. A rapid and sensitive method for the quantitation of microgram quantities of protein utilizing the principle of protein-dye binding. Anal Biochem. 1976;72:248–254.
- Taoka Y, Nagano N, Okita Y, et al. Effect of addition of Tween 80 and potassium dihydrogenphosphate to basal medium on the isolation of marine eukaryotes, thraustochytrids. J Biosci Bioeng. 2008;105:562–565.
- Dorrance AM, Graham D, Dominiczak A, et al. Inhibition of nitric oxide synthesis increases erythrocyte membrane fluidity and unsaturated fatty acid content. Am J Hypertens. 2000;13:1194–1202.
- Mansour M. Plasma membrane permeability as an indicator of salt tolerance in plants. Biol Plant. 2013;57:1–10.
- Buitendijk J. Nuclear DNA content in twelve species of Alstroemeria L. and some of their hybrids. Ann Bot. 1997;79:343–353.
- Schulz LC, Bahr JM. Potential endocrine function of the glycolytic enzyme glucose-6-phosphate isomerase during implantation. Gen Comp Endocrinol. 2004;137:283–287.
- Lucarelli G, Rutigliano M, Sanguedolce F, et al. Increased expression of the autocrine motility factor is associated with poor prognosis in patients with clear cell–renal cell carcinoma. Medicine. 2015;94:e2117.
- Meile L, Rohr LM, Geissmann TA, et al. Characterization of the D-xylulose 5-phosphate/D-fructose 6-phosphate phosphoketolase gene (xfp) from Bifidobacterium lactis. J Bacteriol. 2001;183:2929.
- Petrareanu G, Balasu MC, Zander U, et al. Preliminary X-ray crystallographic analysis of the D-Xylulose 5-phosphate phosphoketolase from Lactococcus lactis. Acta Crystallogr Sect F Struct Biol Cryst Commun. 2010;66:805–807.
- Strachan J, Vidale P, Hodges K, et al. Reversible acetylation of a critical enzyme regulates glycolysis: regulation of glycolytic enzyme phosphoglycerate mutase-1 by Sirt1 protein-mediated deacetylation. J Biol Chem. 2012;287:3859.
- Lunt SY, Vander Heiden MG. Aerobic glycolysis: meeting the metabolic requirements of cell proliferation. Annu Rev Cell Dev Biol. 2011;27:441–464.
- Yin H, Zhang R, Xia M, et al. Effect of aspartic acid and glutamate on metabolism and acid stress resistance of Acetobacter pasteurianus. Microb Cell Fact. 2017;16:109.
- Zelle RM, de Hulster E, van Winden WA, et al. Malic acid production by Saccharomyces cerevisiae: engineering of pyruvate carboxylation, oxaloacetate reduction, and malate export. Appl Environ Microbiol. 2008;74:2766–2777.
- Yang J, Yang W, Feng J, et al. Enhanced polymalic acid production from the glyoxylate shunt pathway under exogenous alcohol stress. J Biotechnol. 2018;275:24–30.
- Serrano JA, Camacho M, Bonete MJ. Operation of glyoxylate cycle in halophilic archaea: presence of malate synthase and isocitrate lyase in Haloferax volcanii. FEBS Lett. 1998;434:13–16.
- Tang R-R, Chi Z, Jiang H, et al. Overexpression of a pyruvate carboxylase gene enhances extracellular liamocin and intracellular lipid biosynthesis by Aureobasidium melanogenum M39. Process Biochem. 2018;69:64–74.
- Friedrich T, Dekovic DK, Burschel S. Assembly of the Escherichia coli NADH:ubiquinone oxidoreductase (respiratory complex I). Biochim Biophys Acta - Bioenerg. 2016;1857:214–223.
- Keohane CE, Steele AD, Fetzer C, et al. Promysalin elicits species-selective inhibition of Pseudomonas aeruginosa by targeting succinate dehydrogenase. J Am Chem Soc. 2018;140:1774–1782.
- Kleingardner JG, Bren KL. Comparing substrate specificity between cytochrome c maturation and cytochrome c heme lyase systems for cytochrome c biogenesis. Metallomics. 2011;3:396.
- Shoubridge EA. Cytochrome c oxidase deficiency. Am J Med Genet. 2001;106:46–52.
- Cai X, Haider K, Lu J, et al. Network analysis of a proposed exit pathway for protons to the P-side of cytochrome c oxidase. Biochim Biophys Acta - Bioenerg. 2018;1859:997–1005.
- Srivastava AP, Luo M, Zhou W, et al. High-resolution cryo-EM analysis of the yeast ATP synthase in a lipid membrane. Science. 2018;360:aas9699.
- Zheng J. Energy metabolism of cancer: glycolysis versus oxidative phosphorylation (review). Oncol Lett. 2012;4:1151–1157.
- Malecki M, Bitton DA, Rodríguez-López M, et al. Functional and regulatory profiling of energy metabolism in fission yeast. Genome Biol. 2016;17:240.
- Brar SK, Verma M, Barnabé S, et al. Impact of Tween 80 during Bacillus thuringiensis fermentation of wastewater sludges. Process Biochem. 2005;40:2695–2705.
- Smirnova GV, Oktyabrsky ON. Glutathione in Bacteria. Biochemistry (Moscow). 2005;70:1199–1211.
- Jones DP. Redefining oxidative stress. Antioxid Redox Signal. 2006;8:1865–1879.
- Chen W, Chao G, Singh KB. The promoter of a H2O2-inducible, Arabidopsis glutathione S-transferase gene contains closely linked OBF- and OBP1-binding sites. Plant J. 1996;10:955–966.
- Davies K. Oxidative stress, antioxidant defenses, and damage removal, repair, and replacement systems. IUBMB Life. 2000;50:279–289.
- Liberek K, Lewandowska A, Zietkiewicz S. Chaperones in control of protein disaggregation. Embo J. 2008;27:328–335.
- Inoue SI, Kinoshita T. Blue light regulation of stomatal opening and the plasma membrane H+-ATPase. Plant Physiol. 2017;174:531.