Abstract
The aim of this study was to examine the effect and mechanism of SENP1 in the protection of vascular endothelial cells by propofol during ischemia-reperfusion (IR) injury. Thirty-eight patients undergoing minor lower limb orthopedic surgery under general anesthesia were included, and divided evenly into sevoflurane group and propofol group. Peripheral blood was collected before fixing tourniquets (T0 time point) and one hour after releasing tourniquets (T1 time point). Contents of endothelin, vWF and malondialdehyde were determined by enzyme-linked immunosorbent assay to evaluate vascular injury. Quantitative real-time polymerase chain reaction was used to determine mRNA expression; western blotting, to determine protein expression; CCK-8 assay, Transwell assay and flow cytometry, to examine the proliferation, migration and apoptosis of human umbilical vein endothelial cells (HUVECs); western blotting and laser scanning confocal microscopy, to analyze autophagy of HUVECs; protein immunoprecipitation, to examine the relationship between SENP1 and the SUMOylation of autophagy-associated VPS34 protein. The results showed that the contents of peripheral blood vessel injury markers and the expression of SENP1 were elevated when vascular injury was induced by IR in patients undergoing orthopedic surgery, whereas propofol reduced these levels. Inhibition of SENP1 expression reduced injury of HUVECs induced by IR. Silencing expression of SENP1 promoted autophagy of HUVECs. SENP1 promoted deSUMOylation of VPS34, leading to reduced autophagy of HUVECs. The study demonstrates that propofol activates autophagic activity of HUVECs by inhibiting SENP1 expression and attenuates vascular endothelial injury induced by IR in orthopedic surgery. This effect may be related to SENP1 regulating the deSUMOylation of VPS34.
Introduction
Good blood circulation plays an extremely important role in maintaining the metabolism and biological functions of tissues and organs [Citation1,Citation2]. Temporary ischemia of local histiocytes often occurs in clinical operations. After the recovery of blood reperfusion under certain conditions, the dysfunction of histiocyte metabolism and structural damage are not alleviated but aggravated [Citation3]. This phenomenon of further aggravation of ischemic injury after blood reperfusion is called ischemia-reperfusion (IR) injury (IRI) [Citation4]. Upper and lower limb operations account for a large proportion in clinical orthopedic surgeries. In order to reduce intraoperative blood loss and maintain a clear surgical field, the tourniquet is widely used in limb surgery [Citation5,Citation6]. However, IRI induced by orthopedic tourniquets results in injury of primary limbs, seriously affecting the prognosis of patients [Citation7]. Mild patients may manifest late muscle fibrosis, contracture and limb dysfunction [Citation8]. Severe cases may have necrosis of limbs, or secondary damages of distant organs such as liver and brain [Citation9]. Vascular injury is the earliest and most basic pathological change in IRI [Citation10]. Therefore, how to alleviate or avoid IRI in orthopedic surgery has become one of the important issues related to the prognosis of patients.
Propofol is a fast and short-acting intravenous anesthetic widely used in induction and maintenance of anesthesia [Citation11,Citation12]. The anesthesia induction of propofol is fast, the recovery and metabolism of patients are rapid, and the incidence of nausea and vomiting after operation is low [Citation13]. It is reported that propofol has a good protective effect on IR-induced tissue and cell injuries in brain, kidney and blood vessels [Citation14,Citation15]. Blood vessels are an important place for nutrient exchange between tissue cells and blood, and vascular injury is also a basic pathological change in IRI [Citation16]. Vascular endothelial injury occurs first in organ IRI, and the proliferation, migration and apoptosis of vascular endothelial cells are significantly changed [Citation17,Citation18]. In addition, vascular endothelial cell injury directly leads to the infiltration of inflammatory cells and inflammatory molecules in blood vessels, which aggravates the injury of tissues and cells [Citation19]. Therefore, it is very important to study the molecular mechanism of IRI of vascular endothelial cells.
Small ubiquitin-like modifier (SUMO) plays an important role in regulating the function and localization of proteins [Citation20]. SUMOylation is a dynamic and reversible covalent modification process [Citation21]. It is reported that SUMOylation can be reversed by SUMO-specific cysteine proteases (SENPs) [Citation22,Citation23]. SENPs play important roles in maintaining the dynamic balance of SUMOylation and ensuring the normal physiological functions of cells and organisms [Citation24]. Abnormal expression of SENPs can destroy the balance of SUMO in vivo and cause the occurrence and development of tumors [Citation25]. SENP proteins include six proteins, namely SENP1, SENP2, SENP3, SENP5, SENP6 and SENP7. Their roles in IR-induced vascular injury are unknown. It is reported that SENP1 protein is involved in the regulation of IRI [Citation26]. In the present study, we investigate whether SENP1 is involved in the process of vascular injury in orthopedic surgery.
Subjects and methods
Subjects
A total of 38 patients undergoing minor orthopedic surgery of lower limbs under general anesthesia (1.5–2 h) at Longgang Orthopedics Hospital of Shenzhen between January 2017 and December 2018 were included in the study. Among the 38 patients, 22 were males and 16 were females (age range, 32- to 65-year old; mean age, 41.3-year old). The 38 patients were divided randomly into sevoflurane group (n = 19) and propofol group (n = 19). None of the patients had severe heart and lung diseases, hepatorenal insufficiency, important organ dysfunction, autoimmune diseases, or long history of drug intake.
After preoperative fasting and abstinence, the patients entered the operating room, and their electrocardiogram (ECG), invasive blood pressure (IBP), peripheral capillary oxygen saturation (SpO2), anal temperature and central venous pressure (CVP) were routinely monitored. The operations were performed by the same group of surgeons. Patients in the propofol group received anesthesia induction by intravenous infusion of 2.5 mg/kg propofol, 1 μg/kg remifentanil and 0.2 mg/kg cisatracurium besilate, and anesthesia maintenance by intravenous infusion of 4 mg/(kg h) propofol and 0.2–0.3 μg/(kg min) remifentanil. Patients in the sevoflurane group received anesthesia induction by inhalation of 8% sevoflurane (fresh gas flow rate, 5 L/min) and intravenous infusion of 1–2 μg/kg remifentanil and 0.2 mg/kg cisatracurium besilate, and anesthesia maintenance by intravenous infusion of 0.2–0.3 μg/(kg min) remifentanil and inhalation of 2–3% sevoflurane. Cerebral state index (CSI) was monitored in all patients and bispectral index (BIS) was maintained at 50–60 by adjusting the propofol infusion rate or the sevoflurane inhalation concentration. Tourniquets were fixed at the upper 1/3 of thighs. The pressure was set at the value of systolic blood pressure plus 100 mmHg before operation. After 60 min, the pressure was relaxed for 10 min. Peripheral blood (10 mL) was collected before fixing tourniquets (T0 time point) and one hour after releasing tourniquets (T1 time point) for subsequent tests.
Ethics statement
All procedures performed in this study were approved by the Ethics Committee of Longgang Orthopedics Hospital of Shenzhen. Written informed consent was obtained from all patients or their families.
Quantitative real-time polymerase chain reaction
Peripheral blood (250 μL) was directly lysed with 750 μL TRIzol LS reagent (Thermo Fisher Scientific, Waltham, MA, USA). Total RNA was extracted using the phenol–chloroform method. The concentration and quality of RNA was measured using ultraviolet spectrophotometry (Nanodrop ND2000, Thermo Scientific, Waltham, MA, USA). Then, cDNA was obtained by reverse transcription from 1 μg RNA and stored at −20 °C. Reverse transcription of mRNA was performed using TIANScript II cDNA First Strand Synthesis Kit (Tiangen, Beijing, China).
SuperReal PreMix (SYBR Green) quantitative real-time polymerase chain reaction (qRT-PCR) kit (Tiangen, Beijing, China) was used to detect mRNA expression of SENP1, using glyceraldehyde 3-phosphate dehydrogenase (GAPDH) as internal reference. The sequences for SENP1 were 5'- GGTTCCGGTTCGGACTTTGT -3' (forward) and 5'- GGTCTTTCGGGTTTCGAGGT -3' (reverse). The sequences for GAPDH were 5'- AGGAGCGAGACCCCACTAACAT -3' (forward) and 5'- GTGATGGCATGGACTGTGGT -3' (reverse). The reaction system (20 μL) was composed of 10 μL SYBR Premix EXTaq, 0.5 μL upstream primer, 0.5 μL downstream primer, 2 μL cDNA and 7 μL ddH2O. PCR conditions were: initial denaturation at 95 °C for 10 min; denaturation at 95 °C for 1 min and annealing at 60 °C for 30 s (40 cycles) (iQ5; Bio-Rad, Hercules, CA, USA). The 2-ΔΔCq method was used to calculate the relative expression of SENP1 mRNA against GAPDH. Each sample was tested in triplicate.
Cells
Human umbilical vein endothelial cells (HUVECs; Cell Bank, Chinese Academy of Sciences, Shanghai, China) were cultured in DMEM (Dulbecco's Modified Eagle Medium) supplemented with 10% fetal bovine serum (FBS), 100 IU/mL penicillin and 100 IU/mL streptomycin at 37 °C, 5% CO2, and 70% humidity. HUVECs were passaged every three days, and those in logarithmic growth were collected for experiments.
HUVECs (1 × 106) were seeded into 6-well plates, and divided into normal control group, IR group and propofol group. The cells in normal control group were cultured DMEM supplemented with 10% FBS, 100 IU/mL penicillin and 100 IU/mL streptomycin at 37 °C, 5% CO2, and 70% humidity. The cells in the IR group were cultured in low-glucose and serum-free DMEM placed in an airtight container. After ventilation with mixed gases of 5% CO2 and 95% N2 for 30 min, the container was sealed and placed in an incubator with 5% CO2 at 37 °C. Fourteen hours later, the medium was discarded, and 100 μL DMEM supplemented with 10% fetal bovine serum (FBS), 100 IU/mL penicillin and 100 IU/mL streptomycin were added into each well for culture at 37 °C and 5% CO2 for 4 h. The cells in the propofol group were treated according to the same protocol as the IR group, and the difference was that 12.3 μmol/L propofol was present during the culture of 14 h.
To transfect HUVECs, the cells (2 × 105) in logarithmic growth were seeded onto 24-well plates one day before transfection, and cultured in antibiotics-free DMEM supplemented with 10% FBS until reaching 70% confluency. In the first vial, 1.5 µL SENP1 small interference RNA (siRNA; 20 pmol/µL; Hanbio, Shanghai, China) was mixed with 50 µL Opti Mem medium (Thermo Fisher Scientific, Waltham, MA, USA). In the second vial, 1 µL Lipofectamine 2000 (Thermo Fisher Scientific, Waltham, MA, USA) was mixed with 50 µL Opti Mem medium. After standing still for 5 min, the two vials were combined for additional waiting at room temperature for 20 min. Then, the mixtures were added onto cells in respective groups. Six hours later, the medium was replaced with DMEM containing 10% FBS. After cultivation for 48 h, the cells were collected for further assays.
CCK-8 assay
HUVECs were seeded at a density of 2,000/well in 96-well plates. At 0, 24, 48 and 72 h, 20 μL CCK-8 reagent (5 g/L; Beyotime, Shanghai, China) was added onto the cells. On the last day, 150 μL CCK-8 reaction solution was added and the cells were incubated at 37 °C for 2 h. Then, the absorbance of each well was measured at 490 nm for plotting cell proliferation curves. Each group was tested in three replicate wells and the values were averaged.
Transwell assay
Transwell chambers (Corning Inc., Corning, NY, USA) were used to evaluate the migration ability of HUVECs. The cells (5 × 105) from each group were seeded into the upper chamber containing 200 μL serum-free DMEM medium. In addition, 500 μL DMEM medium supplemented with 10% FBS was added into the lower chamber. After 24 h, the chamber was removed and the cells in the upper chamber were wiped off. After being fixed with 4% formaldehyde for 10 min, the membrane was stained using Giemsa method for microscopic observation of five random fields (200×). The number of transwell cells was calculated for the evaluation of cell migration ability. All procedures were carried out on ice with pipetting tips being cooled at 4 °C.
Flow cytometry
HUVECs (1 × 106) in each group were washed with precooled phosphate-buffered saline twice and subjected to flow cytometry using ANXN V FITC APOPTOSIS DTEC KIT I (BD Biosciences, Franklin Lakes, NJ, USA) following the manufacturer’s manual to detect cell apoptosis. Cells with ANNEXIN V-positive values were early apoptotic cells, those with PI-positive values were necrotic cells, and those with double positive values were late apoptotic cells.
Western blotting
HUVECs (1 × 106) were trypsinized and collected, and then lysed with precooled Radio-Immunoprecipitation Assay (RIPA) lysis buffer (600 μL; 50 mmol/L Tris-base, 1 mmol/L ethylenediaminetetraacetic acid (EDTA), 150 mmol/L NaCl, 0.1% sodium dodecyl sulfate, 1% TritonX-100, 1% sodium deoxycholate; Beyotime Institute of Biotechnology, Shanghai, China) for 30 min on ice. The mixture was centrifuged at 12,000 g and 4 °C for 10 min. The supernatant was used to determine protein concentration by bicinchoninic acid (BCA) protein concentration determination kit (RTP7102, Real-Times Biotechnology Co., Ltd., Beijing, China). The samples were then mixed with 5× sodium dodecyl sulfate loading buffer before denaturation in boiling water bath for 10 min. Afterwards, the samples (20 µg) were subjected to 10% sodium dodecyl sulfate-polyacrylamide gel electrophoresis at 100 V. The resolved proteins were transferred to polyvinylidene difluoride membranes on ice (250 mA, 1 h) and blocked with 5% skimmed milk at room temperature for 1 h. Then, the membranes were incubated with rabbit anti-human SENP1 (1:1000; Abcam, Cambridge, UK), LC3B (1:1000; Abcam, Cambridge, UK), p62 (1:1000; Abcam, Cambridge, UK) or GAPDH (1:4000; Abcam, Cambridge, UK) monoclonal primary antibodies at 4 °C overnight. After extensive washing five times for 5 min with phosphate-buffered saline with Tween 20, the membranes were incubated with goat anti-rabbit horseradish peroxidase-conjugated secondary antibody (1:4,000; Abcam, Cambridge, UK) for 1 h at room temperature before washing five times for 5 min with phosphate-buffered saline with Tween 20. Then, the membrane was developed with enhanced chemiluminescence detection kit (Beyotime, Shanghai, China) for imaging. Image lab v3.0 software (Bio-Rad, Hercules, CA, USA) was used to acquire and analyze imaging signals. The relative contents of target proteins were expressed against GAPDH.
Enzyme-linked immunosorbent assay
Endothelin, vWF and malondialdehyde (MDA) kits (R&D Systems, Minneapolis, MN, USA) were used to determine their concentrations. In microplates, standards (50 μL) and samples (10 μL serum and 40 μL diluent) were added into predefined wells, while blank wells were left empty. In the wells for standards and samples, horseradish peroxidase-labelled conjugates (100 μL) were added before sealing the plates for incubation at 37 °C for 1 h. After washing the plates five times, substrates A (50 μL) and B (50 μL) were added into each well. After incubation at 37 °C for 15 min, stop solution (50 μL) was added into each well, and the absorbance of each well was measured at 450 nm within 15 min.
Protein immunoprecipitation
293T cells were co-transfected p-CMV-FLAG-SUMO1 and p-CMV-HA-VPS34, or p-CMV-FLAG-SUMO1 and p-CMV-myc-SENP1, and cultured at 37 °C and 5% CO2 for 48 h. Cell sediments were collected, and 106 cells were mixed with 1 mL lysis buffer containing 1% phenylmethyl sulfonyl fluoride before being kept on ice for 30 min with shaking. Then, the mixture was centrifuged at 4 °C and 12,000 g for 15 min before collecting the supernatant. The supernatant with proteins was mixed with 1 μL antibodies (anti-Flag), and gently shaken at 4 °C overnight. During shaking, 10 μL protein A/G agarose beads were washed with lysis buffer, and centrifuged at 3,000 g for 3 min before removing the supernatant. The beads were washed three times. Then, the beads were transferred into cell lysates incubated with antibodies before gentle shaking at 4 °C for 2 h. After the beads were coupled with antibodies, the mixture was centrifuged at 4 °C and 2,000 g for 1 min. After removing the supernatant, the beads were mixed with 1 mL lysis buffer before centrifugation at 4 °C and 2,000 g for 1 min. This washing step was repeated 3–4 times before completely removing the supernatant. Then, 5× sodium dodecyl sulfate loading buffer was added onto the beads and boiled at 100 °C for 5 min. The proteins captured on the beads were examined by Western blotting.
Laser scanning confocal microscopy
HUVECs in each group were seeded onto culture plates at a density of 1 × 105/well. When reaching 70% confluency, the cells were infected with Lv-RFP-LC3B lentivirus (titer, 1 × 108; Hanbio, Shanghai, China) at MOI = 20. Forty-eight hours later, the cells were observed under a laser confocal microscope (SP8; Leica, Wetzlar, Germany), and five fields were selected randomly. Green and red vesicles represented autophagosomes. After autophagosomes fused with lysosomes, green fluorescence disappeared, and only red fluorescence remained. The number of autophagosomes in HUVECs was counted to evaluate the autophagy activity.
Statistical analysis
The results were analyzed using SPSS 20.0 statistical software (IBM, Armonk, NY, USA). The data are expressed as means values with standard deviations (±SD). Data were tested for normality. Multigroup measurement data were analyzed using one-way analysis of variance (ANOVA). In case of homogeneity of variance, Least Significant Difference and Student–Newman–Keuls methods were used; in case of heterogeneity of variance, Tamhane’s T2 or Dunnett’s T3 method was used. Comparison between two groups was carried out using Student’s t-test. p < .05 indicated statistically significant differences.
Results and discussion
The contents of peripheral blood vessel injury markers and the expression of SENP1 are elevated when vascular injury is induced by IR in patients undergoing orthopedic surgery, while propofol reduces these levels
Tourniquets are widely used in orthopedic surgery, but they are easy to induce IRI of limbs [Citation27]. Blood vessels are the earliest site of IRI, and their basic pathological changes are the injuries of vascular endothelial cells [Citation28]. Propofol is a widely used intravenous anesthetic in clinical practice, and it is reported that propofol has the functions of protecting tissues and organs [Citation11]. However, few studies have been performed on the cytoprotective effect and mechanism of propofol in orthopedic surgery. In orthopedic surgery, the removal of tourniquet to restore blood supply to limbs often leads to IRI, and the first place to occur is blood vessels [Citation29]. Endothelin, vWF and MDA are markers of vascular injury, and have diagnostic value for the occurrence of vascular injury [Citation30,Citation31]. To determine the contents of peripheral blood vessel injury markers endothelin, vWF and MDA, as well as the expression of SENP1, we performed enzyme-linked immunosorbent assay (ELISA) and qRT-PCR, respectively. The data showed that the contents of endothelin, vWF and MDA of the sevoflurane group at T1 time point were significantly higher than those at T0 time point (p < .05 for all; . Similarly, the contents of endothelin, vWF and MDA of propofol group at T1 time point were significantly higher than those at T0 time point (p < .05 for all; ). Of note, the contents of endothelin, vWF and MDA of the propofol group at T1 time point were significantly lower than those of sevoflurane group at T1 time point, respectively (p < .05 for all; ). In addition, the level of SENP1 in peripheral blood from the sevoflurane group at T1 time point was significantly higher than that at T0 time point (p < .05), and the level of SENP1 in peripheral blood from the propofol group at T1 time point was also significantly higher than that at T0 time point (p < .05). The level of SENP1 in peripheral blood from the propofol group at T1 time point was significantly lower than that from the sevoflurane group at T1 time point (p < .05; ). The results suggest that the contents of peripheral blood vessel injury markers and the expression of SENP1 are elevated when vascular injury is induced by IR in patients undergoing orthopedic surgery, while propofol reduces these levels.
Figure 1. Contents of peripheral blood vessel injury markers (endothelin, vWF and MDA) and relative expression of SENP1 mRNA in patients with tourniquet-induced orthopedic IR injury. (A, B) Values at T0 versus T1 time points in sevoflurane group (A) and propofol group (B); values in sevoflurane group versus propofol group at T0 and T1 time points (C). *, p < .05. Note: Peripheral blood (10 mL) was collected before fixing tourniquets (T0 time point) and 1 h after releasing tourniquets (T1 time point). ELISA was used to determine the contents of endothelin, vWF and MDA in peripheral blood of patients. qRT-PCR was used to determine the expression of SENP1 mRNA in peripheral blood of patients.
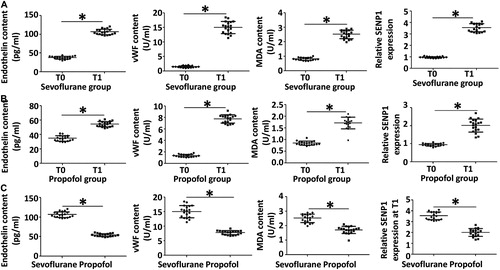
Inhibition of SENP1 expression reduces the injury of HUVECs induced by IR
SENP1 is an important protein in the SENP family, which exerts its biological function by deSUMOylation [Citation32]. SENP1 plays important roles in the occurrence, development and metastasis of tumors. For example, overexpression of SENP1 inhibits the neurogenic capacity of osteosarcoma stem cells and increases their sensitivity to HSVtk/GCV [Citation33]. In addition, p53 mutant activates GTPase RAC1 through SUMOylation pathway, and promotes the occurrence and development of tumors [Citation34]. To further test whether SENP1 exerts its role in HUVECs, we constructed an IR model of HUVECs and carried out qRT-PCR, Western blotting, CCK-8 assay, Transwell assay and flow cytometry. qRT-PCR and Western blotting showed that SENP1 mRNA and protein levels in HUVECs of the IR group were significantly higher than those of the normal control group (p < .05 for both), and propofol treatment of the IR group significantly decreased the expression of SENP1 mRNA and protein (p < .05 for both; ). After silencing of SENP1 mRNA expression with its siRNA, the expression of SENP1 protein in the siR-SENP1 group was significantly lower than that in the siR-NC group (p < .05; ). CCK-8 assay showed that the absorbance of HUVECs in the siR-SENP1 group was significantly higher than that in the siR-NC group at 24, 48 and 72 h (p < .05 for all; ). Transwell assay showed that the number of membrane-crossing cells in the siR-SENP1 group was significantly higher than that in the siR-NC group (p < .05; ). Flow cytometry showed that the apoptotic rate of HUVECs in the siR-SENP1 group was significantly lower than that in the siR-NC group (p < .05; ). The results indicate that inhibition of SENP1 expression reduces the injury of HUVECs induced by IR.
Figure 2. Effect of SENP1 in the inhibition of IR injury by propofol. (A, B) Expression of SENP1 mRNA (A) and protein (B) in HUVECs of normal control (NC), IR, and propofol groups. *, p < .05. (C) Expression of SENP1 protein in HUVECs transfected with siR-negative control (siR-NC) and siR-SENP1. *, p < .05. (D) Proliferation of HUVECs transfected with siR-NC and siR-SENP1, as determined by CCK-8 assay. *, p < .05 compared with siR-NC group. (E) Migration of HUVECs transfected with siR-NC and siR-SENP1, as determined by Transwell assay. *, p < .05. (F) Apoptotic rate of HUVECs transfected with siR-NC and siR-SENP1, as determined by flow cytometry. *, p < .05.
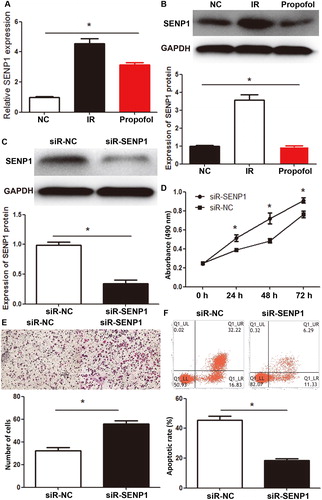
Silencing the expression of SENP1 promotes the autophagy of HUVECs
Autophagy is a highly conserved biological process. By stabilizing the internal environment through the phagocytosis of proteins, nucleic acids and organelles, autophagy reduces cell injuries by stress [Citation35,Citation36]. It is shown that propofol can activate autophagy and exert its cytoprotective effect [Citation37]. However, it is not clear whether SENP1 exerts its function by regulating autophagy. To examine the effect of SENP1 on the autophagy of HUVECs, we determined the expression of autophagy markers, LC3B II and p62 proteins, by Western blotting, and observed autophagosomes using laser scanning confocal microscopy. The data showed that the expression of LC3B II protein in HUVECs of the siR-SENP1 group was higher than that of the siR-NC group, while the expression of p62 in the siR-SENP1 group was lower than that of the siR-NC group (). In addition, the number of autophagosomes in HUVECs of the siR-SENP1 group was significantly higher than that in the siR-NC group (p < .05) (). The results suggest that silencing the expression of SENP1 promotes the autophagy of HUVECs.
Figure 3. Effect of SENP1 on the autophagy of HUVECs transfected with siR-NC and siR-SENP1. (A) Expression of autophagy markers, LC3B II and p62 proteins, in HUVECs transfected with siR-NC and siR-SENP1, as determined by Western blotting. *, p < .05 compared with siR-NC group for the same protein. (B) Number of autophagosomes in HUVECs transfected with siR-NC and siR-SENP1, as determined by laser scanning confocal microscopy. *, p < .05.
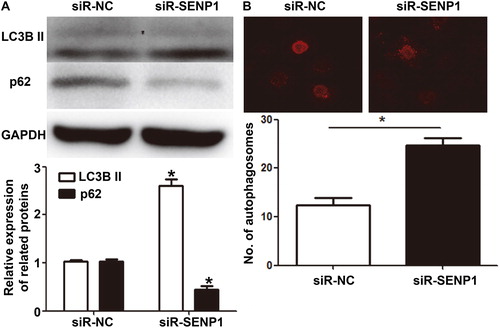
SENP1 promotes the deSUMOylation of VPS34, leading to reduced autophagy of HUVECs
SENP1 plays a role by regulating the deSUMOylation of target proteins, and SENP1 may regulate SUMOylation of autophagy-related proteins. VPS34 is a key gene for autophagosome formation, and SUMOylation participates in regulating the function of VPS34. To examine the mechanism by which SENP1 reduces autophagy, immunoprecipitation was used. The data showed one band for HUVECs treated with only VPS34, but two bands for HUVECs co-transfected with VPS34 and SUMO1 overexpressing plasmids. Moreover, only one band was observed for HUVECs co-transfected with VPS34, SUMO1 and SENP1 overexpressing plasmids, and the molecular weight of this band was also reduced (). The result indicates that SENP1 promotes the deSUMOylation of VPS34, leading to reduced autophagy of HUVECs.
Figure 4. Immunoprecipitation analysis of the effect of SENP1 on SUMOylation of VPS34 by SUMO1. One band was observed for HUVECs treated with only VPS34, but two bands were observed for HUVECs co-transfected with VPS34 and SUMO1 overexpressing plasmids. Moreover, only one band was observed for HUVECs co-transfected with VPS34, SUMO1 and SENP1 overexpressing plasmids, and the molecular weight of this band was also reduced.
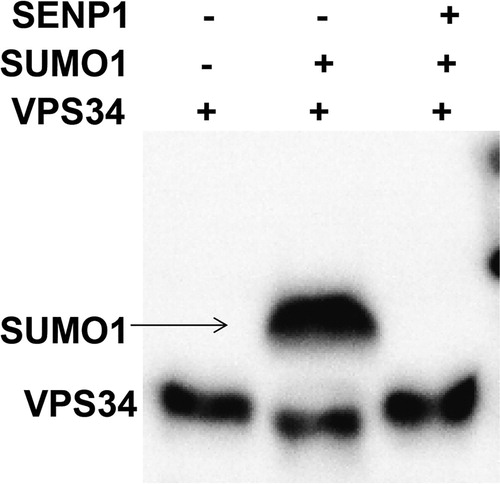
Conclusions
The present study demonstrates that propofol attenuates IR-induced vascular injury by inhibiting SENP1 expression. SENP1 may affect the autophagic activity of vascular endothelial cells through deSUMOylation of VPS34, thus, affecting the degree of vascular endothelial cell injury during IR. By regulating the post-translational SUMOylation of VPS34, we can alleviate injuries caused by IR during surgery.
Author contributions
The final version of the manuscript has been read and approved by all authors, and each author believes that the manuscript represents honest work. YQ and JX collaborated to design the study. YQ performed experiments. YQ and JX analyzed the data. Both authors collaborated to interpret results and develop the manuscript.
Ethical approval and consent to participate
All procedures performed in the current study were approved by the Ethics Committee of Longgang Orthopedics Hospital of Shenzhen. Written informed consent was obtained from all patients or their families.
Consent for publication
Written informed consents for publication of any associated data and accompanying images were obtained from all patients or their parents, guardians or next of kin.
Acknowledgement
The authors wish to thank Dr. Xiangdi Yu from Department of Anesthesiology, Guizhou Provincial People’s Hospital.
Availability of data and materials
The datasets used and/or analyzed during the current study are available from the corresponding author on reasonable request.
Disclosure statement
No potential conflict of interest was reported by the authors.
References
- Gregori-Pla C, Blanco I, Camps-Renom P, et al. Early microvascular cerebral blood flow response to head-of-bed elevation is related to outcome in acute ischemic stroke. J Neurol. 2019;266(4):990–997.
- Koduru SV, Leberfinger AN, Pasic D, et al. Cellular based strategies for microvascular engineering. Stem cell Rev Rep. 2019;15(2):218–240.
- Bromage DI, Taferner S, He Z, et al. Stromal cell-derived factor-1alpha signals via the endothelium to protect the heart against ischaemia-reperfusion injury. J Mol Cell Cardiol. 2019;128:187–197.
- Li Y, Feng D, Wang Z, et al. Ischemia-induced ACSL4 activation contributes to ferroptosis-mediated tissue injury in intestinal ischemia/reperfusion. Cell Death Differ. 2019;26:2284–2299.
- Pinar HU, Pinar A, Mavioğlu Ö, et al. Effect of hydroxyethyl starch 130/0.4 on ischemia-reperfusion determinants in minor lower extremity surgery with tourniquet application. J Clin Anesth. 2015;27(2):105–110.
- Bihari A, Cepinskas G, Forbes TL, et al. Systemic application of carbon monoxide-releasing molecule 3 protects skeletal muscle from ischemia-reperfusion injury. J Vasc Surg. 2017;66(6):1864–1871.
- Ejaz A, Laursen AC, Kappel A, et al. Tourniquet induced ischemia and changes in metabolism during TKA: a randomized study using microdialysis. BMC Musculoskelet Disord. 2015;16(1):326.
- Huang T, Wang W, Tu C, et al. Hydrogen-rich saline attenuates ischemia-reperfusion injury in skeletal muscle. J Surg Res. 2015;194(2):471–480.
- Halladin NL, Zahle FV, Rosenberg J, et al. Interventions to reduce tourniquet-related ischaemic damage in orthopaedic surgery: a qualitative systematic review of randomised trials. Anaesthesia. 2014;69(9):1033–1050.
- Jiang Y, Xie H, Tu W, et al. Exosomes secreted by HUVECs attenuate hypoxia/reoxygenation-induced apoptosis in neural cells by suppressing miR-21-3p. Am J Transl Res. 2018;10(11):3529–3541.
- Ushirozako H, Yoshida G, Kobayashi S, et al. Impact of total propofol dose during spinal surgery: anesthetic fade on transcranial motor evoked potentials. J Neurosurg Spine. 2019;8:1–9.
- Miller KA, Andolfatto G, Miner JR, et al. Clinical Practice Guideline for Emergency Department Procedural Sedation With Propofol: 2018 Update. Ann Emerg Med. 2019;73(5):470–480.
- Hannam JA, Mitchell SJ, Cumin D, et al. Haemodynamic profiles of etomidate vs propofol for induction of anaesthesia: a randomised controlled trial in patients undergoing cardiac surgery. Br J Anaesth. 2019;122(2):198–205.
- Chang YC, Xue WJ, Ji W, et al. The protective effect of propofol against ischemia-reperfusion injury in the interlobar arteries: reduction of abnormal Cx43 expression as a possible mechanism. Kidney Blood Press Res. 2018;43(5):1607–1622.
- Wu H, Zhou J, Ou W, et al. TAK1 as the mediator in the protective effect of propofol on renal interstitial fibrosis induced by ischemia/reperfusion injury. Eur J Pharmacol. 2017;811:134–140.
- Cahill PA, Redmond EM. Vascular endothelium - Gatekeeper of vessel health. Atherosclerosis. 2016;248:97–109.
- Luscher TF, Noll G. Endothelium dysfunction in the coronary circulation. J Cardiovasc Pharmacol. 1994;24(Suppl 3):S16–S26.
- Hohneck AL, Fries P, Stroder J, et al. Effects of heart rate reduction with ivabradine on vascular stiffness and endothelial function in chronic stable coronary artery disease. J Hypertens. 2019;37(5):1023–1031.
- Aekthammarat D, Pannangpetch P, Tangsucharit P. Moringa oleifera leaf extract lowers high blood pressure by alleviating vascular dysfunction and decreasing oxidative stress in L-NAME hypertensive rats. Phytomedicine. 2019;54:9–16.
- Heinrich J, Drewniok C, Neugebauer E, et al. The YoaW signal peptide directs efficient secretion of different heterologous proteins fused to a StrepII-SUMO tag in Bacillus subtilis. Microb Cell Fact. 2019;18(1):31.
- Salahuddin S, Fath EK, Biel N, et al. Epstein-Barr virus latent membrane protein-1 induces the expression of SUMO-1 and SUMO-2/3 in LMP1-positive lymphomas and cells. Sci Rep. 2019;9(1):208.
- Kunz K, Piller T, Muller S. SUMO-specific proteases and isopeptidases of the SENP family at a glance. J Cell Sci. 2018;131(6):jcs211904.
- Baik H, Boulanger M, Hosseini M, et al. Targeting the SUMO pathway primes all-trans retinoic acid-induced differentiation of nonpromyelocytic acute myeloid leukemias. Cancer Res. 2018;78(10):2601–2613.
- Wei B, Huang C, Liu B, et al. Mitotic phosphorylation of SENP3 regulates DeSUMOylation of chromosome-associated proteins and chromosome stability. Cancer Res. 2018;178(9):2171–2178.
- Jin ZL, Pei H, Xu YH, et al. The SUMO-specific protease SENP5 controls DNA damage response and promotes tumorigenesis in hepatocellular carcinoma. Eur Rev Med Pharmacol Sci. 2016;20(17):3566–3573.
- Gu J, Fan Y, Liu X, et al. SENP1 protects against myocardial ischaemia/reperfusion injury via a HIF1alpha-dependent pathway. Cardiovasc Res. 2014;104(1):83–92.
- Goolsby CA, Kellermann AL, Kirsch TD. Just-in-time instructions for layperson tourniquet application. JAMA Surg. 2019;154(4):363.
- Zhang G, Zou X, Miao S, et al. The anti-oxidative role of micro-vesicles derived from human Wharton-Jelly mesenchymal stromal cells through NOX2/gp91(phox) suppression in alleviating renal ischemia-reperfusion injury in rats. PLoS One. 2014;9(3):e92129.
- Drysch M, Wallner C, Schmidt SV, et al. An optimized low-pressure tourniquet murine hind limb ischemia reperfusion model: inducing acute ischemia reperfusion injury in C57BL/6 wild type mice. PLoS One. 2019;14(1):e0210961.
- Wu Y, Liu W, Zhou Y, et al. von Willebrand factor enhances microvesicle-induced vascular leakage and coagulopathy in mice with traumatic brain injury. Blood. 2018;132(10):1075–1084.
- Wang Y, Che J, Zhao H, et al. Osthole alleviates oxidized low-density lipoprotein-induced vascular endothelial injury through suppression of transforming growth factor-beta1/Smad pathway. Int Immunopharmacol. 2018;65:373–381.
- Wang C, Tao W, Ni S, et al. SENP1 interacts with HIF1alpha to regulate glycolysis of prostatic carcinoma cells. Int J Biol Sci. 2019;15(2):395–403.
- Liu F, Li L, Li Y, et al. Overexpression of SENP1 reduces the stemness capacity of osteosarcoma stem cells and increases their sensitivity to HSVtk/GCV. Int J Oncol. 2018;53(5):2010–2020.
- Yue X, Zhang C, Zhao Y, et al. Gain-of-function mutant p53 activates small GTPase Rac1 through SUMOylation to promote tumor progression. Genes Dev. 2017;31(16):1641–1654.
- Birgisdottir AB, Mouilleron S, Bhujabal Z, et al. Members of the autophagy class III phosphatidylinositol 3-kinase complex I interact with GABARAP and GABARAPL1 via LIR motifs. Autophagy. 2019;15(8):1333–1355.
- Liu Y, Wang J. C9orf72-dependent lysosomal functions regulate epigenetic control of autophagy and lipid metabolism. Autophagy. 2019;15(5):913–914.
- Yang M, Wang Y, Liang G, et al. Alzheimer's disease presenilin-1 mutation sensitizes neurons to impaired autophagy flux and propofol neurotoxicity: role of calcium dysregulation. J Alzheimers Dis. 2019;67(1):137–147.