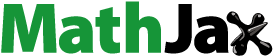
Abstract
Pretreated waste Streptomyces fradiae biomass was used as biosorbent to remove tetracycline (TC) from model aqueous solutions. The biosorption study was conducted in a batch system to evaluate the impact of initial pH, biosorbent dosage, initial TC concentration and contact time. Langmuir, Freundlich and Dubinin–Radushkevich (D–R) models were applied to describe the biosorption isotherm equilibrium studies. Langmuir and Freundlich models fitted the equilibrium data better than the D–R model. The monolayer biosorption capacity of the pretreated biomass for TC was 38.61 mg g−1. The experimental data were also tested in terms of biosorption kinetics using pseudo-first-order and pseudo-second order kinetics models. The results showed that the TC biosorption process followed well pseudo-second-order kinetics.
Introduction
In recent years, contaminants of emerging concern (or emerging contaminants), including pharmaceuticals, have been receiving considerable attention in view of their potential to negatively impact human health and ecosystems. The principal sources of these contaminants are the wastewater from the pharmaceutical industry and urban areas (domestic and hospital discharges), wastes from agriculture and aquaculture, as well inadequate disposal of expired medicines. Pharmaceuticals are regarded as ‘pseudo-persistent’ contaminants due to their continued introduction into the ecosystems, despite their continuous degradation and removal by various processes [Citation1–3]. According to Bush [Citation4], these compounds are divided into the following groups: anti-inflammatories and analgesics, antibiotics, antiepileptics, antidepressants, lipid lowering agents, antihistamines, β-blockers, and other substances (barbiturates, narcotics, antiseptics and contrast media).
Among the different pharmaceutical contaminants, antibiotics are the most frequently detected compounds in different types of waters (wastewater, surface water, drinking water, ground water) and solids (sludge, soil, and sediments) [Citation5]. Their intensive use in human and animal medicine has two consequences in terms of environmental impact: the presence of active residues and the formation of antibiotic resistant bacteria.
Special attention has been given to tetracyclines (TCs), аs they are the second most widely used antibiotics in veterinary, aquaculture, and human medicine, because of their low price and broad-spectrum antimicrobial activity against a variety of diseases [Citation5, Citation6]. TCs are relatively poorly absorbed by humans and animals, large fractions are excreted into the environment in active forms via the urine and feces [Citation7]. The significant stability of TCs in the aquatic environment is determined by their highly hydrophilic character and low volatility.
Tetracycline is the most frequently detected antibiotic in various environmental water samples [Citation8, Citation9]. Daghrir and Drogui [Citation5], Spongberg and Witter [Citation10] reported that the removal of TC in the wastewater treatment plants is incomplete and varied from 12% to 80%.
With the growing concern for the environment, human and animal health, different methods have been proposed for tetracycline removal from aqueous solutions, including membrane, photolysis, electrochemical, and photocatalytic processes [Citation11–15]. All of them have various limitations, such as high energy consumption, secondary pollution, and complicated removal processes.
An attractive alternative to the methods listed above is adsorption, due to its simplicity, safety, economic feasibility, and removal efficiency at low sorbent concentrations. A wide variety of adsorbents have been studied for tetracyclines removal from aqueous solutions: carbon-based materials (biochar, activated carbon, carbon nanotubes, graphene) [Citation16], silica [Citation17], pumice stone [Citation18], biopolymers (chitosan and modified alginate) [Citation19, Citation20], algae [Citation21, Citation22], metal-organic frameworks – MOFs [Citation23–25], magnetic nanocomposites [Citation26–28].
The choice of a suitable adsorbent is determined not only by its characteristics and efficiency for TC removal, but also by its cost.
From this point of view, biosorbents generated from waste microbial biomassеs are an interesting alternative for tetracycline antibiotics removal.
To our best knowledge, little information on microbial biomass used as a biosorbent for TC removal has been reported. For example, Erşan [Citation29] investigated the removal of TC, using Saccharomyces cerevisiae biomass immobilized on polypropylene and polystyrene from aqueous solutions in a batch system. Açıkel and Kip [Citation30] studied TC adsorption using biocomposites prepared by the immobilization of Candida yeasts (Candida lipolytica, Candida membranaefaciens, Candida tropicalis, Candida utilis) and Rhizopus delemar fungus in chitosan. However, ТC biosorption studies have not been carried out with waste microbial biomasses, but with specially cultivated yeast and fungal strains, which were then thermally killed.
The use of waste microbial biomasses shows a number of advantages in biosorption: low cost, absence of nutrient medium and maintenance of pure microbial cultures, high sorption and desorption rate, work over a wide pH range, use of simple equipment, rapid and easy regeneration of the biomass used. The application of dead waste microbial mycelium for wastewater treatment, could offer a solution to two ecological problems. On the one hand, the disposal of large amounts of waste biomasses is eliminated or decreased to a minimum. On the other hand, a new and economically effective technology for purification of industrial waters contaminated with contaminants of emerging concern is developed.
Special attention should be paid to the biomass of the genus Streptomyces. As today 80% of antibiotics and other bioactive compounds are produced by it, the waste biomass unfit for use originated as a by-product from the pharmaceutical industry has been studied as a potential biosorbent only for removal of heavy metals and dyes from aqueous solutions [Citation31, Citation32]. However, its applicability for the removal of antibiotics from waste waters is still unknown.
The aim of the present study is to evaluate for the first time the biosorption potential of pretreated waste Streptomyces fradiae biomass obtained after tylosin production, for TC removal from model aqueous solutions in batch mode.
The optimum biosorption conditions were determined as a function of pH, biomass dosage, contact time and initial tetracycline concentrations. Equilibrium, and kinetics studies were also carried out.
Materials and methods
Biomass preparation
Waste Streptomyces fradiae biomass was obtained from tylosin production, (‘Biovet’ - AD, Peshtera, Bulgaria). The waste mycelium was washed thoroughly with deionized water and dried in an oven at 80 °C for 12 h. This biomass was used in further biosorption experiments as the control sample. The control biomass (5 g) was pretreated in different ways as follows: stirred at 250 rpm for 6 h in 100 mL of 0.1 mol L−1 HCl; stirred at 250 rpm for 30 min in 100 mL methanol; stirred at 250 rpm for 30 min in 100 mL 95% (v/v) ethanol; stirred at 250 rpm for 30 min in 100 mL acetone; boiled at 110 °C for 15 min 100 mL of 1 mol L−1 NaOH. All the pretreated samples were collected by centrifugation at 4000 rpm (MPW-260R) for 10 min, washed with deionized water several times to neutral pH, and then dried at 80 °C for 12 h.
Reagents and equipment
All chemicals used in the study were of analytical reagent grade and were used without further purification. Stock TC solution (Sigma), 250 mg L−1, was prepared with deionised water. For the biosorption experiments, TC solutions of different concentrations (25, 50, 75 and 100 mg L−1) were prepared by adequate dilution of the stock solution with deionized water. All solutions were prepared daily. The concentration of TC in the solution before and after biosorption was determined using a UV–vis spectrophotometer (Amersham Biosciences Ultrospec 3300) at 355 nm in dilute acidic solution (0.1 mol L−1 HCl) by the calibration curve method. Fourier transform infrared (FT-IR) spectra of ethanol pretreated unloaded biomass and TC-loaded biomass prepared as KBr discs were recorded at 400–4000 cm−1 wavenumber range at a scan rate of 180 scans and a spectral resolution of 2 cm−1 using a Thermo Nicolet Avatar 330 FT-IR spectrometer (Thermo Electronic Corporation).
The point of zero charge (pHZPC) of the biomass was determined by the solid addition method [Citation33]. The experiment was done in series, as 0.1 g of the pretreated biomass and 50 mL of 0.1 mol L−1 solution of KNO3 with different pH values were transferred into 250 mL Erlenmeyer flasks. The pHs of the solutions were adjusted from 2.0 to 10.0 by adding either 0.1 mol L−1 HNO3 or 0.1 mol L−1 NaOH. The suspensions were agitated for 48 h at room temperature. The final pH values (pHf) of the solutions were then measured. The difference between the initial and final pH values (pHi–pHf) was plotted against the initial pH (pHi). The point of pHZPC corresponds to the intersection of the resulting curve with the x-axis.
Biosorption experiments
In order to evaluate the effect of pH, the biosorbent dosage, initial concentration of TC and contact time, a set of biosorption experiments were conducted in 250 mL Erlenmeyer flasks, at a constant agitation speed of 250 rpm in batch mode. The experiments were carried out by varying the initial pH of the solutions from 3.0 to 9.0; biosorbent dosage from 1 to 4 g L−1; initial concentration of TC from 25.0 to 100.0 mg L−1; contact time from 10 to 210 min. The temperature was kept constant at 25 ± 1 °C. The initial pH of the solution was adjusted with 0.1 mol L−1 HCl or 0.1 mol L−1 NaOH. At the end of the biosorption process, the biosorbent was separated from the solution by centrifugation at 4000 rpm (MPW-260R) for 10 min and the residual TC concentration was determined as described above.
The Erlenmeyer flasks in which the biosorption experiments were carried out were wrapped in aluminum foil to prevent TC exposure to sunlight and its photodegradation. Blank experiments without the addition of adsorbent were also conducted to confirm that no adsorption occurred on the glass vial wall. These experiments showed that the loss of TC was less than 2% during the whole biosorption process (data not shown).
All experiments were performed in triplicate. For all graphical representations, the mean values of the three independent experiments were considered, but the standard deviations within the triplicates were too small to be plotted as an error bar (< 1%).
TC biosorption capacity and removal efficiency were calculated according to the following equations:
(1)
(1)
(2)
(2)
where q is the uptake, mg g−1; V is the volume of the solution in the flask, L; Ci and Cf are the initial and final concentration of TC in the solution, mg L−1; W is the amount of biomass, g.
Results
Pretreatment effects
To investigate the effect of pretreatment on the TC uptake, the control biomass was treated with hydrochloric acid, sodium hydroxide and organic solvents (methanol, ethanol and acetone).
As demonstrated in , the highest TC uptakes were observed when the waste biomass was pretreated with ethanol and methanol, the calculated uptakes being 28.72, and 21.47 mg g−1, respectively. TC uptake for the control biomass was 12.55 mg g−1.
Figure 1. Effect of different pretreatments on the TC uptake of waste S. fraidae biomass (V = 100 mL; W = 1 g L−1; pH = 5.0; t = 180 min; Co = 50 mg L−1;): A – control; B – HCl; C – NaOH; D – CH3OH; E – C2H5OH; F – (CH3)2CO.
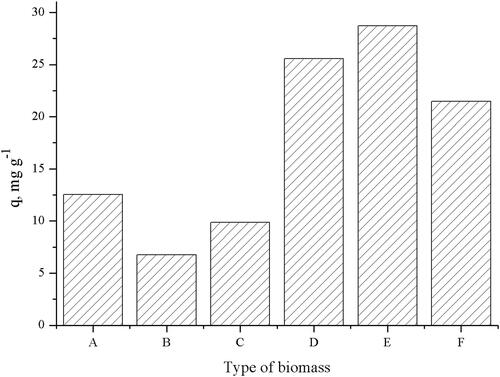
Since pretreatment of the biomass with ethanol enhanced TC biosorption by 129%, ethanol pretreated biomass was used for all further experiments.
FTIR analysis
The FT-IR spectra of ethanol-pretreated unloaded and TC-loaded biomass were taken to obtain information about the nature of possible interactions between the functional groups of the biomass and the TC molecule ().
The infrared spectrum of the unloaded biomass, (S1 in ), displays a number of peaks. The broad and strong band between 3500 and 3200 cm−1 was assigned to the overlapping of –OH and/or –NH stretching vibrations [Citation34, Citation35]. The bands in the range of 3000–2800 cm−1 are representative of symmetric (2926 cm−1) and asymmetric (2854 cm−1) stretching of CH3 and CH2 groups.
The peak at 1747 cm−1 was assigned to carbonyl stretch in carboxylate groups (un-ionised) or ester groups. The peak at 1647 cm−1 was attributed to amide I (-C-O stretching coupled with –N-H deformation). The peak at 1540 cm−1 is probably caused by –NH bending and –CN stretching vibrations of –C–(=O)–NH– group in its transforms and was assigned to amide II. At 1457 cm−1 –CH2 scissoring or –CH3 anti-symmetrical bending vibration are observed. The peaks at 1160 and 1063 cm−1 are indicative of P–O stretching and P–OH stretching vibrations, respectively [Citation31, Citation36, Citation37]. The FTIR spectrum of the TC free biomass showed that the main functional groups are carboxyl, hydroxyl, phosphate and/or amino groups. The spectrum obtained after TC biosorption (S2) revealed the presence of similar peaks with different intensities and forms. The peak at 3292 cm−1 lost its intensity and changed shape after TC biosorption, the peak at 1747 cm−1 shifted to 1700 cm−1 perhaps caused by the presence of the carbonyl group (-C = O) in the TC molecule. The peak at 1647 cm−1 was also with changed shape and intensity.
Effect of pH
The biosorption experiments were carried out with initial TC concentration of 50 mg L−1 and biomass concentration of 1 g L−1 by varying the pH of the solutions over a range of 3.0–9.0.
The uptake of TC by the pretreated biomass was almost constant at pH range from 4.0 to 6.0, where the removal efficiencies ranged from 58.12 to 57.5% (). At pH 7, a slight decrease in the removal efficiency was observed, and at pH 9.0 removal was 30.11%.
Figure 3. Effect of pH on TC biosorption ((A) efficiency of biosorption at different pHs; (B) point of zero charge of biomass).
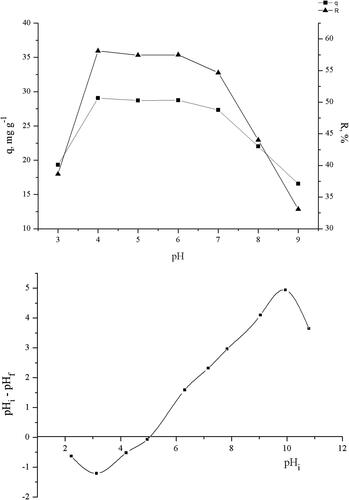
The point of zero charge of the pretreated waste Streptomyces fradie biomass was determined graphically as 5.07 (). This indicates that below this pH value the pretreated biomass acquires a net positive charge due to the protonation of functional groups, whereas above this pH value a negative charge exists on the surface of the studied biomass.
Next experimental studies were conducted at initial pH values 5.0.
Effect of biomass dosage
To examine the effect of biomass dosage (1–4 g L−1), batch sorption studies at a fixed initial concentration of 50 mg L−1, pH = 5.0, contact time of 180 min were carried out. The results are shown in .
The percentage of TC removal increased from 57.44 to 83.08% when the biomass dosage increased from 1.0 to 4.0 g L−1, but the TC uptake decreased from 28.72 to 10.38 mg g−1. An optimum biomass dosage of 2 g L−1 for further experiments was chosen.
Effect of contact time and initial TC concentration
The effect of contact time on the biosorption uptake of TC using pretreated S. fradiae biomass at different initial concentrations is illustrated in .
Figure 5. Effect of contact time and initial TC concentration on biosorption uptake onto pretreated biomass (1 – 25 mg L−1, 2–50 mg L−1, 3–75 mg L−1, 4–100 mg L−1; W = 2.0 g L−1, pH = 5.0).
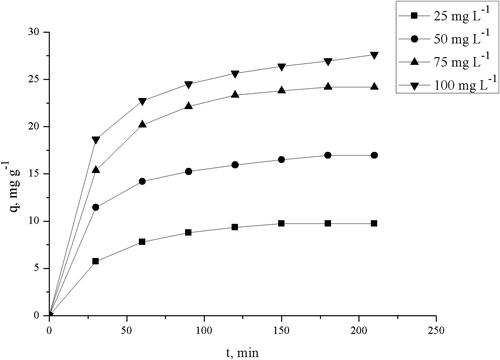
The results show that equilibrium was established within a contact time of 90, 120 and 150 min for 25, 50, 75 and 100 mg L−1 initial TC concentrations. The equilibrium can be attained within 150 min. The uptake capacities increased from 9.75 to 27.64 mg g−1 with the increase of the initial TC concentration from 25 to 100 mg L−1.
Equilibrium isotherm models
Langmuir, Freundlich and Dubinin–Radushkevich (D–R) adsorption models were used to describe the biosorption equilibrium. These models are simple, easily interpretable, and widely used in biosorption studies [Citation38–40].
The Langmuir model assumes monolayer coverage and constant binding energy between surface and adsorbate. The Langmuir isotherm model can be expressed as:
(3)
(3)
The linear expression of this equation can be written as:
(4)
(4)
where Ce is the equilibrium concentration of solute remaining in the solution (mg L−1), qe is the amount of adsorbate adsorbed per mass unit of adsorbent at equilibrium (mg g−1), qm is the maximum adsorption capacity (mg g−1). The essential characteristics of the Langmuir isotherm can be expressed in terms of a dimensionless constant or separation factor or equilibrium parameter, RL, which is defined as:
(5)
(5)
where Co is the highest initial TC concentration.
RL is used to describe the favourable nature of the adsorption process, where RL > 1 is unfavourable, RL = 0 is linear, 0 < RL < 1 is favourable, and RL = 0 is irreversible.
The Freundlich isotherm is an empirical equation and is shown to be satisfactory for low concentrations. The Freundlich isotherm model has the following linearized form:
(6)
(6)
where KF, (mg g−1)(L mg−1)1/n is the Freundlich constant and n indicates the affinity between the sorbent and sorbate.
Dubinin–Radushkevich isotherm model was applied to describe the biosorption nature (physical or chemical). The linearized form of this model is expressed as follows:
(7)
(7)
where qe (mol g−1) is the amount of sorbate by the sorbent at equilibrium, Ce (mol L−1) is the equilibrium concentration of solute, qD-R (mol g−1) is the maximum biosorption capacity, β (mol2(J2)−1) is related to biosorption energy, and ε is the Polanyi potential, that can be written as:
(8)
(8)
where T is the temperature in K, R is the universal gas constant (8.314 J mol−1K−1).
The mean free energy of biosorption E (kJ mol−1) can be calculated using the relationship:
(9)
(9)
If the magnitude of i is between 8 and 16 kJ mol−1, then the biosorption process is supposed to proceed via a chemisorption reaction, and for values of E < 8 kJ mol−1 the biosorption process is of physical nature.
The linearized plots of the three isotherm models are given in .
Figure 6. Langmuir (A), Freundlich (B) and Dubinin–Radushkevich (C) isotherm models – linearised forms.
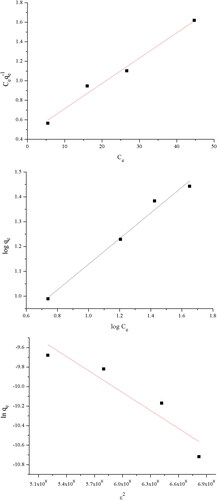
The calculated isotherm constants and correlation coefficients are given in .
Table 1. Langmuir, Freundlich and Dubinin–Radushkevich isotherm parameters of TC biosorption.
The Langmuir and Freundlich isotherm models showed good fit to the experimental data with high correlation coefficients. It can be concluded that the biosorption of TC on the pretreated Streptomyces fradiae biomass occurs on a monolayer and heterogeneous surface. The maximum capacity calculated from the Langmuir model was 38.61 mg g−1. The RL factor calculated for the highest initial concentration of TC was 0.149, indicating favourable biosorption of TC under the studied conditions. The value 1/n obtained from the Freundlich model was between 0 and 1, and represented beneficial biosorption. This model does not predict any saturation on the surface.
The Dubinin–Radushkevich isotherm model showed imperfect fit to the experimental data, the correlation coefficient was low −0.942. The mean free energy was calculated to be 9.02 kJ mol−1 and the maximum biosorption capacity was 75.9 mg g−1.
Biosorption kinetics
In this study, Lagergren’s pseudo-first-order and Ho’s pseudo-second-order models were applied to the experimental data in order to identify the biosorption kinetics of TC on the pretreated Streptomyces fradiae biomass [Citation41, Citation42].
The linear form of the pseudo-first-order rate equation by Lagergren is given as:
(10)
(10)
where qt (mg g−1) is the amount of biosorption at time t (min), qe is the amount of biosorption equilibrium (mg g−1), and k1 is the rate constant of pseudo first-order reaction (min−1).
The values of k1 and qe can be determined from the slope and the x-intercept of the linear plot of lg(qe – qt) versus t.
The linear form of the pseudo-second-order kinetic model can be presented as:
(11)
(11)
where k2 is the rate constant of pseudo-second order reaction (g mg−1 min−1).
The linear plot of t/qt against time is used to determine qe and k2 from the slope and the intercept.
The linear plots for the pseudo-first-order and the pseudo-second-order models for the biosorption of TC onto pretreated waste biomass are shown in and , respectively.
The rate constants, TC uptakes and correlation coefficients are presented in .
Table 2. Parameter values calculated using Lagergren and Ho’s models for the biosorption of TC on ethanol pretreated biomass.
Ho’s model fits better the kinetics of TC biosorption on the pretreated biomass as than the Lagergren model in terms of R2. Furthermore, the calculated equilibrium uptake, qecal, is in good agreement with the experimental values, qeexp (16.97 mg g−1). The calculated initial sorption rate is 0.75 mg g−1 min−1.
Discussion
It is well known that the cell wall of microbial cells plays a key role in the biosorption removal of different pollutants from aqueous solutions due to the presence of a great number of functional groups with different charge and geometry (carboxyl, hydroxyl, amine, sulfate, sulfhydryl, etc.) [Citation39].
Chemical treatment of microbial waste biomasses leads to the removal of unwanted organic compounds as well to improvement in the biosorption capacity. Alkaline treatment is known to disrupt microbial cell walls, exposing additional functional groups, leading to solubilization of certain cell constituents such as lipids and removal of soluble glucans and impurities on the microbial cells, which increase the availability of the binding sites and uptake for metal ions, dyes and other contaminants from aqueous solutions [Citation43–45]. In our study, this treatment led to a decrement in the biosorption uptake. The same effect was observed when hydrochloric acid was used.
The positive effect of organic solvents (methanol, ethanol and acetone) on the TC biosorption may be explained by the increase in the availability of the binding sites responsible for the biosorption process, as well as an improvement in the access of TC to the binding centres of biomass. A similar conclusion was made by Göksungur et al. [Citation46].
The FTIR spectra revealed that the major functional groups playing the role of active sites on the biosorbent surface in the TC uptake process included hydroxyl, carboxyl and/or amine groups.
The acidity of the medium is a key factor in the biosorption processes. The pH value of the solution strongly influences not only the aqueous chemistry of the TC molecule, but also the surface charge of the biosorbent.
The biosorption behaviour at various pHs could be explained with the effect the pH of the medium has on the surface charges and active sites of both TC and pretreated biomass [Citation47]. TC is a molecule with multiple ionizable functional groups: -dimethylammonium, -tricarbonylamide and -phenolic diketone. These functional groups undergo protonation–deprotonation reactions, forming cation species at pH < 3.3, zwitterion species between pH 3.3 and 7.7, or anion species at pH > 7.7 [Citation48].
Multiple simultaneous interactions between polar and charged functional groups of pretreated Streptomyces fraidae waste biomass and TC are possible, but they were less affected by the variation of pH in the range of 4.0–6.0. Generally, biosorption is probably due to electrostatic attraction. At pH 3.0, the removal efficiency of TC was lower (38.65%), which can be explained with the competition for biosorption sites between TC and H+ ions. When pH is above 7.7, the main TC species in the solution are negatively charged and the biomass is negative too (according the pHZPC). Thus, electrostatic repulsion occurs, and the removal efficiency decreases.
Similar conclusions were made by Erşan [Citation29], who found that the optimum pH for the removal of TC on free Saccharomyces cerevisiae biomass, immobilized biomass on polypropylene and polystyrene was 6.0. The values of the pHPZC were found to be 5.338, 5.495 and 5.416, respectively.
The biomass dosage is another important parameter because it determines the capacity of a biosorbent for a given initial concentration. The increase in TC removal with the biomass dosage was due to an increase in the adsorptive and active sites of the biomass. However, the biosorption capacity decreased. In actual practice, the optimal biomass concentration should be defined as the lowest concentration that still gives reasonable removal efficiency [Citation35]. In our study for optimal biomass concentration 2 g L−1, the removal efficiency was 67.89%.
The biosorption of TC by the pretreated waste Streptomyces fradiae biomass was studied as a function of contact time at different initial concentrations of TC, in order to decide whether equilibrium was reached. The experimental results show that the biosorption of TC occurred in two steps: an initial fast step, dependent on the initial concentration, and a slower second step, which continued until equilibrium was reached. The first step could be explained with the availability of enough free active binding sites on the biosorbent surface, but during the second step, the number of binding sites is limited, and the effectiveness decreased. In the studied concentration range of TC, the equilibrium can be attained within 150 min.
Erşan [Citation29] studied the effect of adsorption time on TC adsorption on free and immobilized Saccharomyces cerevisiae biomass by varying the contact time from 2.5 to 240 min. At 100 mg L−1 initial TC concentration, 150 min optimum removal time was found, whereas a longer contact time (240 min) caused desorption [Citation29]. Kip and Açıkel [Citation30] reported that biosorption equilibrium during TC removal by Candida utilis immobilized on chitosan was reached for 240 min.
The use of suitable isothermal models makes it possible to obtain information about the biosorbent-adsorbate affinity, the surface properties of the biosorbent, and the nature of the process. Analysis of equilibrium data is useful for the practical design and operation of biosorption systems.
The pretreated Streptomyces fradiae waste biomass showed higher maximum TC uptake capacity (qmax = 38.61 mg g−1) than other reported microbial biomasses: free and immobilized Saccharomyces cerevisiae and Candida utilis immobilized on chitosan. For Candida utilis biomass immobilized in chitosan, the maximum TC uptake was 2.309 mg g−1 (initial TC concentration 10–100 mg L−1, pH 4, contact time 240 min) [Citation30]. The maximum TC uptake capacities for free and immobilized Saccharomyces cerevisiae biomass were 14.830 and 18.346 mg g−1, respectively (initial TC concentration 10–150 mg L−1, pH 6, biosorbent dosage 0.05 g L−1, contact time 150 min) [Citation29]. In the study of Sayğılı and Güzel [Citation49] the equilibrium data for TC biosorption by tomato processing waste-activated carbon, were also represented by the Langmuir model, where the maximum biosorption capacity was 416.7 mg g−1 (initial TC concentration 200–800 mg L−1, pH 5.8, biosorbent dosage 0.2 g L−1, contact time 5 h). According to Zhu et al. [Citation50] the maximum tetracycline adsorption capacity of the GO/CA composite fibres predicted by the Langmuir model reached 131.6 mg g−1 (initial TC concentration 10–60 mg L−1, pH 5, biosorbent dosage 2.0 g L−1, contact time 180 min).
In fact, the studies of different authors are difficult to compare: the maximum biosorption capacity is determined under different operating conditions and with different adsorbents. Тhe high values of the maximum biosorption capacity indicated in the text refer to specially synthesized adsorbents: tomato processing waste-activated carbon [Citation49], and graphene oxide/calcium alginate composite fibre prepared by a freeze-drying method [Citation50].
The kinetics of biosorption provides important information for assessing the performance of the biosorbents. In this study, the most popular kinetics models were used: the pseudo-first-order and pseudo-second-order models. The studied biosorption process fitted well Ho’s model. The pseudo-second kinetic model is one of the most frequently used ones, and the big advantage of this model is its accuracy in describing the whole range of the experimental kinetic data [Citation18, Citation29, Citation32].
Conclusions
A novel, environmentally friendly, efficient and inexpensive biosorbent was prepared by chemical pretreatment of waste Streptomyces fradiae biomass obtained after tylosin production. The effectiveness of the biosorbent for the uptake of TC from aqueous solutions was evaluated. No significant influence of pH in the range of 4.0–7.0 on the uptake of TC was observed. The maximum biosorption capacity, under optimum experimental conditions calculated by the Langmuir model, was 39.61 mg g−1. The removal process was relatively fast and reached equilibrium within 150 min. The kinetics of TC biosorption followed a pseudo-second order model. The FTIR analysis revealed the presence of hydroxyl, carboxyl and amine groups on the pretreated biomass surface and possible interactions between the amine and hydroxyl groups and the TC molecule. The results obtained indicate that the pretreated waste Streptomyces fradiae biomass can potentially be used in removing TC antibiotic from aqueous solutions. Further experiments will be focused on the immobilization of biomass on different carriers, removal of TC and co-removal of TC and metal ions. It should be noted that the recovery and reuse of biomass is essential, because a continuous supply of a fresh sorbent will be difficult. Usually, after following several sorption/desorption cycles, having lost its sorption capacity, the waste solid biosorbent could be used for production of activated carbon.
Data availability
All data that support the findings reported in this study are available from the corresponding author upon reasonable request.
Disclosure statement
No potential conflict of interest was reported by the authors.
Funding
The authors received no financial support for the research and authorship of this article.
References
- Richardson BJ, Lam PKS, Martin M. Emerging chemicals of concern: pharmaceuticals and personal care products (PPCPs) in Asia, with particular reference to Southern China. Mar Pollut Bull. 2005; 50(9):913–920.
- Ashton D, Furlong ET, Meyer MT, et al. Pharmaceuticals, hormones, and other organic wastewater contaminants in US streams, 1999–2000: a national reconnaissance. Environ Sci Technol. 2002; 36:1202–1211.
- Paffoni C, Welte B, Gousailles M, et al. New molecules involved by the European directives: from wastewater to drinking water treatment plants. J Eur Hydrol. 2006; 37:21–38.
- Bush K. Antimicrobial agents. Curr Opin Chem Biol. 1997; 1(2):169–175.
- Daghrir R, Drogui P. Tetracycline antibiotics in the environment: a review. Environ Chem Lett. 2013;11(3):209–227.
- Chopra I, Roberts M. Tetracycline antibiotics: mode of action, applications, molecular biology, and epidemiology of bacterial resistance. Microbiol Mol Biol Rev. 2001; 65(2):232–260.
- Aghuw KN, MacGowan A. Pharmacokinetics and pharmacodynamics of the tetracyclines including glycylcyclines. J Antimicrob Chemother. 2006; 58(2):256–265.
- Luo Y, Xu L, Rysz M, et al. Occurrence and transport of tetracycline, sulfonamide, quinolone, and macrolide antibiotics in the Haihe River Basin. Environ Sci Technol. 2011; 45:1827–1833.
- Bai Y, Meng W, Xu J, et al. Occurrence, distribution and bioaccumulation of antibiotics in the Liao River Basin in China. Environ Sci Process Impacts. 2014; 16:586–593.
- Spongberg AL, Witter JD. Pharmaceutical compounds in the wastewater process stream in Northwest Ohio. Sci Total Environ. 2008;397(1–3):148–157.
- Li SZ, Li XY, Wang DZ. Membrane (RO-UF) filtration for antibiotic wastewater treatment and recovery of antibiotics. Sep Purif Technol. 2004;34(1–3):109–114.
- Kosutic K, Dolar D, Asperger D, et al. Removal of antibiotics from a model wastewater by RO/NF membranes. Sep Purif Technol. 2007;53(3):244–249.
- Jiao S, Zheng S, Yin D, et al. Aqueous photolysis of tetracycline and toxicity of photolytic products to luminescent bacteria. Chemosphere. 2008; 73:377–382.
- Zhang H, Liu F, Wu X, et al. Degradation of tetracycline in aqueous medium by electrochemical method. Asia-Pacific Jrnl of Chem Eng. 2009;4(5):568–573.
- Daghrir R, Drogui P, Ka I, et al. Photoelectro-catalytic degradation of chlortetracycline using Ti/TiO2 nano-structured electrodes deposited by means of a pulsed laser deposition process. J Hazard Mater. 2012; 199–200:15–24.
- Xiang Y, Xu Z, Wei Y, et al. Carbon-based materials as adsorbent for antibiotics removal: mechanisms and influencing factors. J Environ Manag. 2019; 237:128–138.
- Turku I, Sainio T, Paatero E. Thermodynamics of tetracycline adsorption on silica. Environ Chem Lett. 2007;5(4):225–228.
- Guler UA, Sarioglu M. Removal of tetracycline from wastewater using pumice stone: equilibrium, kinetic and thermodynamic studies. J Environ Health Sci Eng. 2014; 12(1):79.
- Kang J, Liu H, Zheng YM, et al. Systematic study of synergistic and antagonistic effects on adsorption of tetracycline and copper onto a chitosan. J Colloid Interface Sci. 2010; 344(1):117–125.
- Zhang X, Lin X, He Y, et al. Study on adsorption of tetracycline by Cu-immobilized alginate adsorbent from water environment. Int J Biol Macromol. 2019;124(1):418–428. 2019;
- Cavas L, Gokoglu M. Caulerpa scalpelliformis as an antibiotic carrier. Turk J Biochem. 2011; 36(2):93–101.
- Li WC, Wong MH. A comparative study on tetracycline sorption by Pachydictyon coriaceum and Sargassum hemiphyllum. Int J Environ Sci Technol. 2015;12(8):2731–2740.
- Chen X, Jiang X, Yin C, et al. Facile fabrication of hierarchical porous ZIF-8 for enhanced adsorption of antibiotics. J Hazard Mater. 2019; 367:194–204.
- Zhao R, Ma T, Zhao S, et al. Uniform and stable immobilization of metal-organic frameworks into chitosan matrix for enhanced tetracycline removal from water. Chem Eng J. 2020; 382:122893.
- Li K, Li JJ, Zhao N, et al. Removal of tetracycline in sewage and dairy products with high-stable MOF. Molecules. 2020; 25(6):1312.
- Ahamad T, Ruksana Chaudhary AA, et al. Fabrication of MnFe2O4 nanoparticles embedded chitosan-diphenylureaformaldehyde resin for the removal of tetracycline from aqueous solution. Int J Biol Macromol. 2019; 134:180–188.
- Li B, Ma J, Zhou L, et al. Magnetic microsphere to remove tetracycline from water: adsorption, H2O2 oxidation and regeneration. Chem Eng J. 2017; 330:191–201.
- Lin Y, Xu S, Jia L. Fast and highly efficient tetracyclines removal from environmental waters by graphene oxide functionalized magnetic particles. Chem Eng J. 2013; 225:679–685.
- Erşan M. Removal of tetracycline using new biocomposites from aqueous solutions. Desal Wat Treat. 2016;57(21):9982–9992.
- Kip F, Açıkel Ü. Removal of tetraclycine by biocomposites synthesized with immobilization of Rhizopus delamar and Candida types. J Fac Eng Archit Gazi Univ. 2019; 34(3):1417–1426.
- Sahmoune MN. Performance of Streptomyces rimosus biomass in biosorption of heavy metals from aqueous solutions. Microchem J. 2018; 141:87–95.
- Kirova G, Velkova Z, Stoytcheva M, et al. Biosorption of Pb(II) ions from aqueous solutions by waste biomass of Streptomyces fradiae pretreated with NaOH. Biotechnol Equip. 2015; 29(4):689–695.
- Mondal MK. Removal of Pb(II) from aqueous solution by adsorption using activated tea waste. Korean J Chem Eng. 2010; 27(1):144–151.
- Bayramoğlu G, Celik G, Arica MY. Biosorption of reactive blue 4 dye by native and treated fungus Phanerocheate chrysosporium: batch and continuous flow system studies. J Hazard Mater. 2006; 137:1689–1697.
- Veneu DM, Pino GAH, Torem ML, et al. Biosorptive removal of cadmium from aqueous solutions using a Streptomyces lunalinharesii strain. Miner Eng. 2012; 29:112–120.
- Cojocaru C, Diaconu M, Cretescu I, et al. Biosorption of copper (II) ions from aqua solutions using dried yeast biomass. Colloid Surface A Physicochem Eng Asp. 2009; 335(1-3):181–188.
- Han MH, Yun YS. Mechanistic understanding and performance enhancement of biosorption of reactive dyes tuffs by the waste biomass generated from amino acid fermentation process. Biochem Eng J. 2007;36(1):2–7.
- Romera E, González F, Ballester A, et al. Biosorption with algae: a statistical review. Crit Rev Biotechnol. 2006; 26:223–235.
- Gadd GM. Biosorption: critical review of scientific rationale, environmental importance and significance for pollution treatment. J Chem Technol Biotechnol. 2009;2009(84):13–28.
- Liu Y, Liu YJ. Biosorption isotherms, kinetics and thermodynamics. Sep Purif Technol. 2008;2008(61):229–242.
- Sağ Y, Aktay Y. Kinetic studies on sorption of Cr (VI) and Cu (II) ions by chitin, chitosan and Rhizopus arrhizus. Biochem Eng J. 2002; 12(2):143–153.
- Ho YS, McKay G. Pseudo-second order model for sorption processes. Process Biochem. 1999;34(5):451–465.
- Göksungur Y, Üren S, Güvenç U. Biosorption of copper (II) ions by caustic treated waste Baker’s yeast biomass. Turk J Biol. 2003; 27:23–29.
- Das N, Charumathi D, Vimala R. Effect of pretreatment on Cd2+ biosorption by mycelial biomass of Pleurotus florida. Afr J Biotechnol. 2007; 6(22):2555–2558.
- Patel R, Suresh S. Kinetic and equilibrium studies on the biosorption of reactive black 5 dye by Aspergillus foetidus. Bioresour Technol. 2008; 99(1):51–58.
- Göksungur Y, Üren S, Güvenç U. Biosorption of cadmium and lead ions by ethanol treated waste baker’s yeast biomass. Bioresour Technol. 2005; 96:103–109.
- Xu XR, Li XY. Sorption and desorption of antibiotic tetracycline on marine sediments. Chemosphere. 2010; 78(4):430–436.
- Hsu LC, Liu YT, Syu CH, et al. Adsorption of tetracycline on Fe (hydr)oxides: effects of pH and metal cation (Cu2+, Zn2+and Al3. +). R Soc Open Sci. 2018; 5(3):171941.
- Sayğılı H, Güzel F. Effective removal of tetracycline from aqueous solution using activated carbon prepared from tomato (Lycopersicon esculentum Mill.) industrial processing waste. Ecotoxicol Environ Saf. 2016; 131:22–29.
- Zhu H, Chen T, Liu J, et al. Adsorption of tetracycline antibiotics from an aqueous solution onto graphene oxide/calcium alginate composite fibers. RSC Adv. 2018;8(5):2616–2621.