Abstract
The pollen fertility of photoperiod/temperature sensitive genic male sterile (P/TGMS) wheat is controlled by light and/or temperature. Circular RNA (circRNA) and long non-coding RNA (lncRNA) are known to participate in the development of anthers in plants, but their impact on male sterility in the P/TGMS line is not well understood. In this study, we carried out high-throughput sequencing to investigate the differential expression of lncRNAs and circRNAs and their biological functions in anthers of photo-thermosensitive genic male sterile (PTGMS) wheat line BS366-42L during the transition phase of male fertility under four different photoperiod and temperature treatments. Eight lncRNAs, 40 mRNAs and three circRNAs were screened out and thought as essential candidates that closely related to male sterility. Gene ontology (GO) and Kyoto Encyclopedia of Genes and Genomes (KEGG) analyses were performed to predict the potential functions of differentially expressed RNAs. The results indicated that carbohydrate-related metabolism was important for male sterility in the wheat PTGMS line BS366-42L. lncRNA/circRNA-mRNA-miRNA (ceRNA) integrate networks were constructed to reflect their complex inner association with male sterility. Our study provides a systematic perspective on the potential function of RNAs in male fertility in PTGMS lines of wheat.
Keywords:
Introduction
Wheat (Triticum aestivum L.) is one of the most important grain and forage crops throughout the world, and it comprises one-fifth of the total energy consumed by humans and serves as the major food source for one-third of the global population [Citation1]. Hybrid breeding is a potentially disruptive technology that is widely used in plant production to improve the yield per area, enhance yield stability and produce other favourable agronomic traits [Citation2]. In recent years, hybrid breeding has had remarkable success in several allogamous species, such as maize, sunflower, sorghum, sugar beet and rye, but it has not been fully exploited in autogamous crops [Citation3]. Remarkably, hybrid wheat currently accounts for less than 1% of the total wheat acreage planted in the world [Citation4]. P/TGMS can respond to light and temperature owing to its fertility. The features of transformable fertility enable the P/TGMS lines to propagate via self-pollination under environmental conditions that restore male fertility and outcross with restorer lines for hybrid seed production under conditions that suppress male fertility [Citation5,Citation6]. The discovery and successful utilization of P/TGMS greatly promotes the process of scale development of hybrid wheat [Citation5].
Recently, non-coding RNA (ncRNA), including microRNA (miRNA), long non- coding RNA (lncRNA) and circular RNA (circRNA), have been a focus of research in plants and animals [Citation7]. Many studies have shown that lncRNAs can regulate genes at the transcriptional and post-transcriptional levels by acting as signals, decoys, scaffolds and guides [Citation8, Citation9]. In addition, lncRNA as bait could bind miRNAs and block the interaction between miRNAs and their target genes. For example, in Brassica rapa, lncRNA IPS1 (INDUCED BY PHOSPHATE STARVATION1) could competitively bind to miR399, resulting in up-regulated expression of its targets gene PHO2 [Citation10]. Circular RNA (circRNA), another novel type of non-coding RNA, is produced from precursor mRNAs (pre-mRNAs) through back-splicing. CircRNAs form covalently closed loop structures with a process in which a 3′ splicing acceptor site is joined to a 5′ splicing donor site. These closed loop structures enable them to avoid degradation by RNase R [Citation11]. Recent studies have also shown that circRNAs participate in plant responses to biotic and abiotic stresses. For example, the circRNAs in Arabidopsis can respond to heat and low-light and high-light stresses [Citation12].
The pollen sterility of the PTGMS line is controlled by temperature and/or photoperiod [Citation6,Citation7]. Recently, many studies have shown that non-coding RNAs are involved in plant fertility. For example,the overexpression of tae-miR167 in Arabidopsis, could negatively regulate target mRNAs AtARF6 and AtARF8, and cause abnormalities in anther, inducing male sterility phenotypes [Citation13]. In addition, the photoperiod-sensitive male sterility (PSMS) gene pms3, cloned from a PSMS rice line (Nongken 58S), encodes a long non-coding RNA designated LDMAR that is required for normal male fertility of the rice plant under long-day condition[Citation14]. However, there are few reports on the connection of lncRNA, miRNA and circRNA on male sterility in crops. Therefore, researching into the activity of lncRNA and circRNA may lead to significant developments in pollen and pollen sterility in wheat PTGMS lines.
Materials and methods
Plant materials, growth conditions and sample collection
The wheat (Triticum aestivum L.) PTGMS line BS366-42L was used in this study. All the plants were planted and managed until the four-leaf stage as described by Bai et al. (2017) [Citation7]. The plants were randomly transferred to four light and temperature treatments in artificial climate incubators: 12 °C (10 L/14D and 14 L/10D, L: light; D: dark) and 20 °C (10 L/14D and 14 L/10D, L: light; D: dark) at a relative humidity of 60–80% for the entire reproductive period of 10 d. Anthers from more than 50 different plants, including those from the whole process of meiosis, were collected from each treatment. All the samples were rapidly frozen in liquid nitrogen and stored in at −80 °C until the RNA was extracted. The seed setting rate was calculated as described by Yuan et al. (2020) Citation15]:
RNA sequencing and transcript assembly
Sequencing libraries were generated using ribosomal RNA (rRNA)-depleted RNA according to the manufacturer’s instructions. The constructed libraries were then sequenced on an Illumina HiSeqTM 2500 (125 bp PE). The genome of high quality reads was mapped using HISAT (v. 2.0.6) undefined. Filtered clean reads were processed to map to the wheat reference genome (IWGSC RefSeq v. 1.0) using TopHat software undefined [Citation17]. Cufflinks was used to assemble the transcript assembly and estimate the abundance undefined [Citation18]. The data reported in this article have been deposited in the National Genomics Data Center (NGDC) Genome Sequence Archive (GSA) database under the GSA accession no CRA003350 (https://bigd.big.ac.cn).
Prediction of lncRNA and circRNA and analysis of function
The novel transcripts (>200 bp) were processed to identify long noncoding RNAs (lncRNAs) based on four computational approaches, including the Coding Potential Calculator (CPC, score <0) [Citation19], Coding-Non-Coding Index (CNCI score <0) [Citation20], Coding Potential Assessment Tool (CPAT score <0) [Citation21] and Pfam (E value < 0.01). The remaining transcripts were considered to be reliably expressed lncRNAs. CIRI software [Citation22] was used to identify the circRNA. The levels of expression of circRNAs were reflected by mapped back-splicing junction reads per million mapped reads (RPM).
To understand the function and interactions among these RNAs, Gene Ontology (GO) terms and the Kyoto Encyclopedia of Genes and Genomes (KEGG) were performed on DEGs, parent genes of circRNA and targets of lncRNA using the DAVID tool undefined [Citation23]. P values and q values were used to test the reliability of the analysis.
Construction of the lncRNA/circRNA-miRNA-mRNA network
It was reported that mRNAs, lncRNA and circRNA could regulate the same miRNA to play important roles on biological processes in plant development [Citation24]. To understand the connections among miRNAs, mRNAs, lncRNA and circRNA and explain the function of these non-cording RNAs in fertility conversion for a wheat PTGMS line in more detail, we performed interactions analysis between miRNAs and the differentially expressed mRNAs/genes (DEGs), lncRNAs (DELs) and circRNAs (DECs) using miRanda software [Citation25]. The mRNA/miRNA-lncRNA-circRNA network maps were visualized using Cytoscape software (v. 3.6.1) (http://cytoscape.org/).
Quantitative real-time PCR validation
Quantitative real-time PCR (qRT-PCR) was performed using a SYBR Premix Ex TaqTM Kit (TaKaRa, Dalian, Japan) on a CFX96 Touch™ Real-Time PCR Detection System (Bio-Rad Laboratories, Hercules, CA, USA), following the manufacturer’s instructions. The protocol used to perform qPCRs involved pre-denaturation at 95 °C for 2 min, followed by 40 cycles of 95 °C for 15 s and 60 °C for 30 s. The results were analyzed using the 2-ΔΔCt method [Citation26], and the mean values with standard errors (±SE) of three biological replicates are presented. Wheat 18S gene served as the reference control. The primers used in this study are listed in Supplemental Table S1. All the experiments were performed in triplicate.
Results
Phenotypic characteristics of fertility of PTGMS wheat line BS366
Iodine stain tests were performed to characterize the features of fertility in BS366 under four conditions: 12 °C (10 L/14D and 14 L/10D, L: light; D: dark) and 20 °C (10 L/14D and 14 L/10D). The pollen grains were stained fully black at 20 °C (10 L/14D and 14 L/10D), whereas all the wrinkled, inadequately stained and completely aborted characteristics at 12 °C (10 L/14D) and partial black staining at 12 °C (14 L/10D) indicated that the changes in environment could induce the conversion of male fertility. The rate of pollen iodine staining subjected to the four conditions (12 °C 10 L/14D, 12 °C 14 L/10D, 20 °C 10 L/14D and 20 °C 14 L/10D) were 4%, 24%, 81% and 90%, respectively (). Moreover, the seed setting rates were also studied. The averaged seed setting rate from these four conditions were 7.76%, 21.96%, 71.66% and 75.11%, respectively, which was consistent with the results of iodine staining of the pollen (, Supplemental Table S2). These results indicated that light and temperature might play important roles in the transformation of fertility.
Figure 1. Phenotypic characteristics of pollen fertility and seed setting rate in PTGMS wheat line BS366. (A–D): KI–I2 staining of pollen under four conditions including 12 °C 10 L/14D, 12 °C 14 L/10D, 20 °C 10 L/14D and 20 °C 14 L/10D. Scale bars = 1 mm; (E): Statistics of iodine staining rates and seed setting rates.
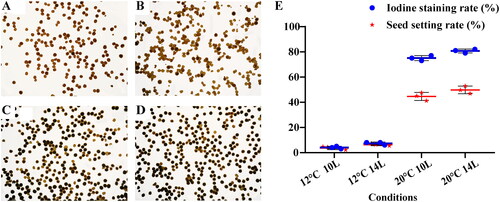
Predictions and properties of lncRNAs and circRNAs
Given the crucial roles of lncRNAs and circRNAs in response to temperature and light changes in plants, Illumina sequencing was performed, which aimed to find essential candidates. After removing the redundant and low quality reads (Supplemental Table S3), a total of 2,629 lncRNAs and 1,043 circRNAs (Supplemental Table S4, ) were identified from all the chromosomes (Supplemental Table S5, ). Eight types, including antisense/sense genic exonic, genic intronic, intergenic downstream and intergenic upstream lncRNAs (), and four kinds of circRNAs including intergenic type, intronic type, antisense type and exonic type () were identified. The minority of lncRNAs (4.11%) were antisense genic exonic type, and other types ranged from 10.45% to 16.54% (). The majority of circRNAs were intergenic type (54%), followed by intronic circRNAs (41%), and the minority (4.11%) were antisense genic exonic type (Figure D). Besides, the majority (31.28%) of lncRNAs ranged from 200 to 400 bp, and approximately a quarter of the identified circRNAs (28.95%) were more than 2,000 bp (), which corresponds with the features of lncRNAs and circRNAs in other species [Citation27].
Figure 2. Comparison of lncRNAs and circRNAs. (A): LncRNAs from Pacbio data by using CPC, CNCI, CPAT and Pfam. (B): Length distribution of isolated lncRNAs and circRNAs. (C and D): Classification analysis of isolated lncRNAs and circRNAs, respectively. (E): Statistics of identified lncRNAs and circRNAs on each chromosome in PTGMS wheat line BS366.
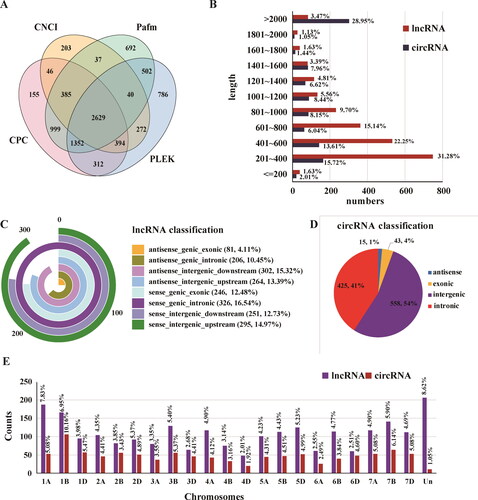
Analysis of differentially expressed mRNAs, lncRNAs and circRNAs
To investigate the function of the isolated lncRNAs and circRNAs, which could respond to light and temperature, during the conversion of fertility in the PTGMS line BS366, DEGs, DELs and DECs were screened out and analyzed subsequently. A total of 2,341, 3,883, 6,426 and 6,694 DEGs (); 113, 131, 204 and 215 DELs (); and 94, 150, 152 and 84 DECs () were identified in 12 °C 14 L vs. 10 L, 10 L 20 °C vs. 12 °C, 14 L 20 °C vs. 12 °C and 20 °C 14 L vs. 10 L comparable groups, respectively. In order to understand the different effects between light period and temperature more deeply, two group comparison analyses were performed. In total, 378 DEGs, 24 DELs and 15 DECs were considered as photoperiod-related candidates (), and 4,789 DEGs, 137 DELs and 48 DECs were thought as thermo-related candidates (). There were barely detectable differences in the numbers between up- and down-regulated photoperiod-related DEGs, DELs and DECs (), particularly in the photoperiod-related DELs and DECs. Down-regulated DEGs and DELs comprised a larger amount than that of the downregulated ones in the thermo-related DEGs and DELs (), while downregulated DECs comprised less than half of those that were upregulated ().
Figure 3. Differentially expressed mRNAs, lncRNAs and circRNAs. The mRNAs, lncRNAs and circRNAs differentially expressed in four different light and temperature conditions (A-C), in photoperiod-induced male sterility groups (D-F) and thermo-induced male sterility groups (G-I). Note: Up- and down-regulated genes from overlapped genes of each group were analyzed with a bar graph.
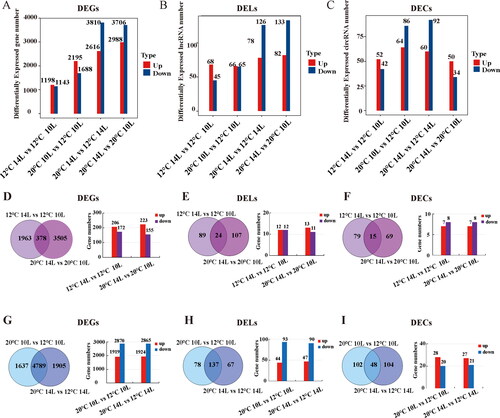
Functional analysis of differentially expressed mRNAs, lncRNAs and circRNAs
Based on the expression profiles analysis, we found that highly significant patterns of expression were apparent in photoperiod- () and thermo-induced () male sterility-related DEGs, DELs and DECs. To obtain a better understanding of the mechanisms involved in light- and temperature-induced male sterility, we performed GO enrichment and KEGG pathway analyses for photoperiod and thermo-induced male sterility-related DEGs, targets of DELs and hosts of DECs, respectively. The top significantly enriched GO terms are shown in . The most obvious photoperiod-induced male sterility-related DEGs that were associated with biological processes were response to hydrogen peroxide, followed by response to reactive oxygen species. The red or far-red light signalling pathway and regulation of long-day photoperiodis were also enriched in the GO terms for DEGs. For photoperiod-induced male sterility-related targets of DELs, enriched GO terms included 3′-UTR-mediated mRNA destabilization, protein lipoylation, reductive pentose-phosphate cycle, photorespiration, cell cycle and cell division. For photoperiod-induced male-sterility related host of DECs were associated with the regulation of reactive oxygen species, metabolic process response to superoxide, programmed cell death, response to ozone, response to ethylene, response to osmotic stress and nitric oxide biosynthetic process.
Figure 4. Hierarchical cluster and functional analysis of photoperiod-induced overlapped DEGs, DELs and DECs. Heatmap of overlapping DEGs (A), DELs (B) and DECs (C). Enriched biological process of DEGs (D), DELs (E) and DECs (F). Gene expression is represented by colours, with brighter red for higher values and brighter blue for lower values.
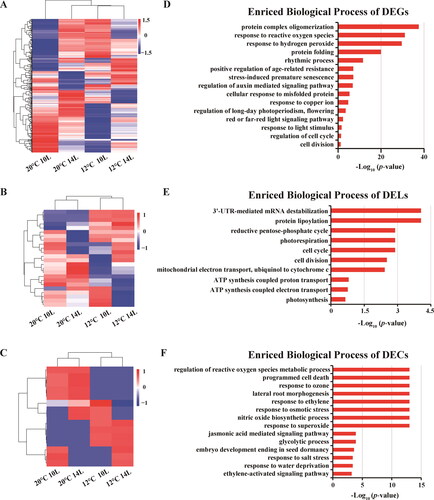
Figure 5. Hierarchical cluster and functional analysis of themo-induced overlapped DEGs, DELs and DECs. Heatmap of overlapping DEGs (A), DELs (B) and DECs (C). Enriched biological process of DEGs(D), DELs (E) and DECs (F). Gene expression is represented by colours, with brighter red for higher values and brighter blue for lower values.
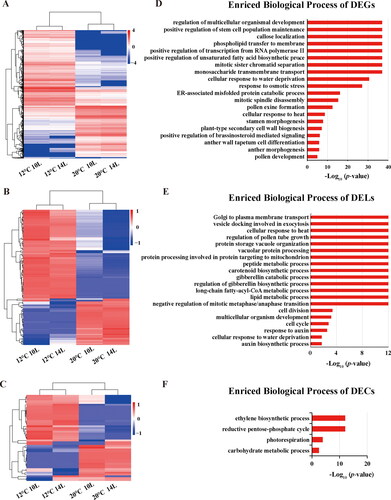
In addition, it was found that the GO terms including anther morphogenesis, anther wall tapetum cell differentiation, pollen exine formation and mitotic sister chromatid separation were enriched in thermo-induced male sterility-related DEGs (). The targets of DELs for thermo-induced male sterility-related that were enriched included the auxin biosynthetic process, cellular response to water deprivation, response to auxin, regulation of gibberellin biosynthetic process, gibberellin catabolic process and regulation of pollen tube growth (). The carbohydrate metabolic process, photorespiration, reductive pentose-phosphate cycle and ethylene biosynthetic process were enriched for the thermo-induced male sterility-related hosts of DELs ().
The KEGG pathway of photoperiod-induced male sterility-related DEGs, targets of DELs and hosts of DECs were enriched in glucose-related biosynthesis and metabolism, including carbon metabolism, galactose metabolism, fructose and mannose metabolism and glycolysis/gluconeogenesis (Supplemental Figure S1). Unlike the enriched KEGG pathway of photoperiod-induced male sterility-related DEGs, the targets of DELs and hosts of DECs, thermo-induced male sterility-related DEGs, targets of DELs and hosts of DECs were primarily enriched in cell proliferation and differentiation and cell signal transduction-related pathways, such as the Wnt signalling pathway, p53 signalling pathway, mitogen-activated protein kinase (MAPK) signalling pathway, oocyte meiosis and cell cycle (Supplemental Figure S2).
Analysis of photo-thermo respond DEGs, DELs and DECs
In order to further identify and characterize the male sterility-related genes, DEGs, DELs and DECs from photoperiod- and thermo-induced male sterility were combined and analyzed. A total of 40 DEGs, eight DELs and three DECs were identified from four conditions (). Highly significant patterns of expression were also apparent in these RNAs (), particularly in the DECs. Three DECs annotated as intergenic genes revealed highly significant patterns of expression in four conditions (). GO analysis was also performed on these RNAs. The results showed that the enriched GO terms on these DEGs were closely associated with male sterility, including UDP-galactose transmembrane transporter activity, starch biosynthetic process and the auxin-activated signalling pathway (). The enriched GO terms for the targets of DELs were primarily associated with chaperone binding, octanoyl transferase activity and lipoyl (octanoyl) transferase activity in molecular function, photosynthesis cell division and cell cycle in molecular function ().
Figure 6. Hierarchical cluster and functional analysis of photo-thermo responsive DEGs, DELs and DECs. Venn analysis showing male sterility-related differentially expressed mRNAs (A), lncRNAs (B) and circRNAs (C). The cluster heat map of male sterility-related differentially expressed mRNAs (D), lncRNAs (F) and circRNAs (H), red colour represents upregulated, blue colour represents downregulated genes, and heavier colour represents higher fold change. GO analysis of male sterility-related differentially expressed mRNAs (E), lncRNAs (G).
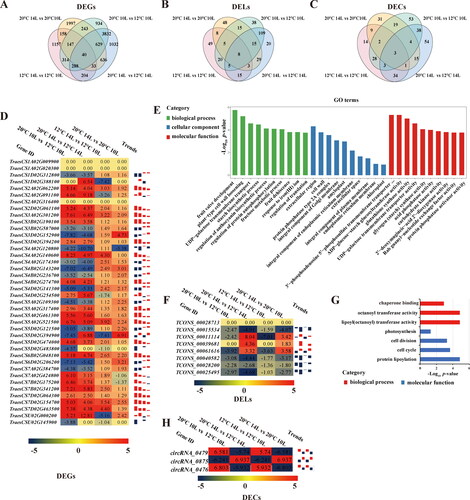
Construction of three lncRNA/circRNA-miRNA-mRNA regulatory networks
In this study, we constructed male sterility-related ceRNA networks from the genes that were regulated by both photoperiod and temperature to clarify the functions of DELs and DECs that were identified. The results showed that one lncRNA could regulate many genes in different ways, and one gene could be regulated by many lncRNAs. For example, lncRNA (TCONS_00028200) could regulate four mRNAs (TraesCS4B02G274700, TraesCS7B02G175200, TraesCS7D02G064300 and TraesCS7A02G384700) by tae-miR531 (). Similarly, one circRNA can ‘sponge’ many miRNAs and then regulate mRNAs. In this study, circRNA_0875 (Chr7A:47534636_47536054_-) attracted tae-miR9673-5p, tae-miR10520, tae-miR9652-3p and tae-miR10518, thus, regulating their associated mRNAs ().
Figure 7. Network analysis of male sterility-related lncRNA-miRNA-mRNA (A) and circRNA-miRNA-mRNA (B). Validation of the expression of the male sterility-related ceRNA network (TraesCS5A02G109300, TraesCS5A02G521500, TraesCS5D02G121500, TraesCSU02G145900, circRNA_0479, circRNA_0476, TCONS_00011114 and tae-miR10518) by qPCR (C). (D): The correlation between the transcriptome data and the expression levels detected by qPCR for the selected genes.
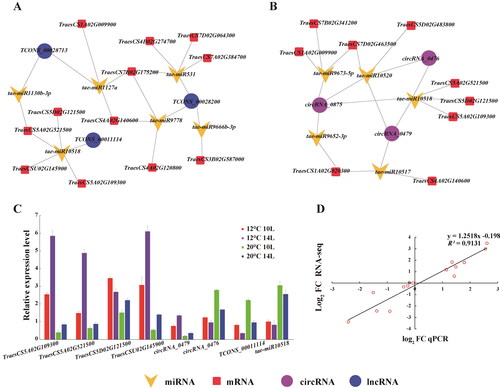
To investigate the function of circRNAs and lncRNAs during the transformation to fertility in more detail, the genes enriched in GO:0000302 (response to reactive oxygen species) from photoperiod-induced DEGs and GO:0048657 (anther wall tapetum cell differentiation) from thermo-induced DEGs were selected to construct lncRNA/circRNA-miRNA-mRNA regulatory networks. As shown in , lncRNA TCONS_00042550 was a ceRNA of one miRNA (tae-miR10516) that targeted five mRNAs (TraesCS3D02G114900, TraesCS4D02G145500, TraesCS3D02G114700, TraesCS3B02G131000 and TraesCS3A02G113000) (). In GO:0000302, circRNA-miRNA-mRNA was simple; one circRNA bound to one miRNA and regulated one mRNA (). In GO:0048657, 19 lncRNAs, five miRNAs and two mRNAs were involved in this lncRNA-miRNA-mRNA ceRNA network (). In addition, eight circRNAs, seven miRNAs and two mRNAs were involved in the circRNA-miRNA-mRNA that was related in the differentiation of anther wall tapetum cell (GO:0048657) (). The expression of these RNAs is shown in , D, F and H. It was found that these RNAs in GO:0000302 were downregulated in low temperature conditions of photoperiod-induced male sterility conditions ().
Figure 8. Construction of the lncRNA/circRNA- mRNA-miRNA regulatory network of GO:0000302 (response to reactive oxygen species (A, C) and GO: 0048657 (anther wall tapetum cell differentiation) (E, G). Blue circle nodes represent lncRNA, red square nodes represent mRNAs and yellow chevron nodes represent miRNA. (B, D, F and H): Expression of RNAs of constructed lncRNA/circRNA- mRNA-miRNA regulatory network.
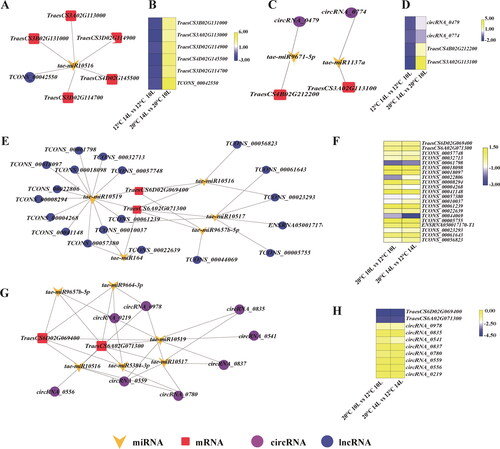
Verification of transcripts and identification of male sterility-related lncRNAs and circRNAs
From the male sterility-related lncRNAs/circRNAs- mRNAs networks, we selected one lncRNA, two circRNAs and four mRNAs that were regulated by the same miRNA (tae-miR10518) to verify the level of expression () and validate the reliability of our transcriptome data (). TraesCS5A02G109300, TraesCS5A02G521500 and TraesCSU02G145900 were upregulated at 12 °C 14 L but downregulated at 20 °C 14 L. However, circRNAs, lncRNAs and miRNAs showed opposite profiles of expression (). In addition, the Pearson’s correlation coefficient between the data generated from the two platforms was high (R2 = 0.9131), indicating that the expression of RNA selected was consistent with the expression observed from sequencing ().
Discussion
The control of male fertility is central to the hybrid seed production for monoclinous crops, including rice and wheat. PTGMS wheat lines are important materials in two-line hybrid systems because of their fertility features. The plants appear to be almost entirely sterile when they are planted in a sterile environment, but they become fertile when planted in a fertile environment [Citation15]. In a previous study, we found that the fertility of PTGMS wheat line BS366 was controlled by temperature and light period Citation7]. Recently, many studies have indicated that some non-coding RNAs regulate anther development and have some relationship to male sterility. Ding et al.[Citation14] found that sufficient amounts of a long-day-specific male-fertility-associated lncRNA (LDMAR) transcript is required for the normal development of pollen of plants grown under long-day conditions in photoperiod-sensitive male sterility (PSMS) rice. Conversely, a low expression of LDMAR will cause premature programmed cell death (PCD) in developing anthers, causing PSMS [Citation14]. Bai et al. Citation7]proposed a possible regulatory model by miRNAs on signalling pathways during the transition to fertility in wheat PTGMS line BS366. MiRNAs as negative regulators regulated their targets, affecting the transition to fertility in the PTGMS line Citation7]. CircRNA is a new hot spot of research and has been widely studied in humans but not in plants; systematic studies on plants are just beginning [Citation28]. It has been confirmed that the circRNA functioned primarily through a miRNA ‘sponge’ and their correlated mRNAs. Previous studies for circRNAs primarily focussed on their roles in anti-stress processes in plants [Citation29]. The fertility conversion for the PTGMS line is a very complex processes, which involves thousands of genes and multiple layers of regulation. To our knowledge, this study is the first to compare the difference in the profiles of expressions of mRNAs, lncRNAs and circRNAs in light- and temperature-induced male sterility in wheat PTGMS line BS366 to identify the key factors involved in the conversion to pollen fertility. Additionally, a potential ncRNA-miRNA-mRNA regulatory network was constructed to provide new insights into the molecular mechanism of the conversion to pollen fertility in wheat PTGMS.
Light is one of the key environmental regulators of multiple developmental processes, including plant morphogenesis, growth, development, physiological metabolism and male sterility [Citation30]. In this study, we found that photoperiod-induced male sterility-related genes were primarily involved in some male sterility-related GO terms, such as the response to reactive oxygen species, response to hydrogen peroxide, regulation of auxin mediated signalling pathway, cell division and programmed cell death. Many studies showed that male sterility is associated with reactive oxygen species (ROS) [Citation31], presumably because excessive ROS can cause serious damage to cells, including protein denaturation, lipid peroxidation, DNA mutation and PCD [Citation32]. Mitochondria provide energy to all of the activities that occur in cells via respiration. Moreover, they generate ROS, including the superoxide anion radical (O2−) and hydrogen peroxide (H2O2). The superfluous accumulation of ROS disturb the redox state balance in plant cells and can disrupt the normal function of mitochondria, resulting in damage to proteins and nucleic acids, lipid peroxidation, necrocytosis, which are deleterious to the growth of plant cells [Citation33]. In addition, respiration-associated metabolism, such as ATP synthesis, is also affected. In this study, in addition to the GO terms, such as the response to hydrogen peroxide, regulation of reactive oxygen species metabolic process and response to superoxide directly associated with ROS, there are many other GO terms, such as ATP synthesis-coupled electron transport and response to stresses in photoperiod-induced male sterility GO analytical terms (), that were consistent with those described in previous studies [Citation34]. We also constructed a lncRNA/circRNA-miRNA-mRNA regulatory network that was associated with the response to reactive oxygen species (GO:0000302) from photoperiod-induced male sterility-related genes (). Five heat shock protein-related genes and TCONS_00042550 were regulated by tae-miR10516 (). Many studies showed that heat shock proteins (Hsps) are involved in anther and pollen development[38]. For example, TMS1, an Hsp40 protein, is required for normal pollen tube growth under heat shock environments in Arabidopsis. In addition, studies showed that the absence of phytochromes could rapidly induce the levels of expression of HSPs [Citation36]. In this study, we also found that the red or far red light signalling pathway, regulation of long-day photoperiodism, flowering and response to light stimulus were eliminated in enriched GO terms, indicating that phytochromes are involved in male sterility. Phytochromes, including phytochrome A (PHYA), PHYB and PHYC, serve as important photoreceptors that can respond to red and far-red light to regulate photomorphogenesis [Citation37]. In rice, the phyAphyBphyC triple mutant and phyAphyB double mutant were less fertile than the wild-type (WT), while the single mutants displayed normal fertility [Citation38].
Additionally, carbohydrate metabolism and photosynthesis-related genes were significantly affected in the phyA phyB double mutant that is involved in male sterility [Citation38]. In this study, a KEGG pathway analysis showed that a large number of genes were enriched in carbon- and sugar-related metabolism, particularly in photoperiod period-induced DEGs, DELs and DECs, such as starch and sucrose metabolism, carbon metabolism and carbon fixation in photosynthetic organisms (). Sugars play the key roles in the development of anthers. Studies have shown that starch is the most abundant sugar in the endothecium at the early stage of rice anther development [Citation39]. It then decreases after meiosis. Additionally, the contents of reducing sugars will increase, while those of non-reducing sugars decrease during the maturation of rice anthers [Citation39][39]. It has also been found that a water deficit has a significant inhibitory effect during later stages of anther development. Thus, this could be the reason why many DEGs, DELs and DECs were enriched in GO terms, including cellular response to water deprivation and response to osmotic stress ( and ). Previous studies revealed that male sterility induced by abiotic stress treatments was caused by impaired carbohydrate metabolism in many plant species. Thus, we deduced that the photoperiod treatments that were abnormal led to a functional deficiency for phytochromes, which dissipated a large amount of carbohydrates and energy, and the male sterility of the PTGMS line resulted from the imbalance in carbohydrate metabolism. The anther wall is an important place for sugar synthesis, secretion, storage, mobilization and regulation [Citation40]. In addition, it was reported that glycosyltransferases are involved in primexine formation and exine patterning in pollen walls [Citation41]. Primexine can provide a substrate on the developing microspore for sporopollenin deposition, polymerization and patterning in pollen wall formation [Citation42,Citation43]. In this study, anther wall tapetum cell differentiation-related DEGs (GO:0048657) were observed to be enriched in thermo-induced male sterility DEGs ( and ). The lncRNA/circRNA-miRNA-mRNA showed that tae-miR164 was involved in both the lncRNA and circRNA network. Bai et al. (2017) revealed that tae-miR164 with its target could regulate the fertility transformation in the PTGMS line [Citation7].
Conclusions
We compared the structural and expressional features of mRNAs, lncRNAs and circRNAs in the photoperiod- and thermo-induced male sterility in wheat PTGMS line BS366. The results showed that ncRNAs were involved in male sterility by modulating lncRNA- and circRNA-associated ceRNA networks. This study provides new insights into the genetic basis of male sterility in a wheat PTGMS line, and additional research is merited to validate the ceRNA mechanisms of ncRNAs and mRNAs.
Ethical standards
We declare that these experiments comply with the ethical standards in China
Supplemental Material
Download Zip (1.2 MB)Disclosure statement
The authors declare that they have no conflict of interest.
Data availability
All data that support the findings reported in this study are available from the corresponding author upon reasonable request.
Additional information
Funding
References
- Wang Z, Li J, Chen S, et al. Poaceae-specific MS1 encodes a phospholipid-binding protein for male fertility in bread wheat. Proc Natl Acad Sci U S A. 2017; 114(47):12614–12619.
- Xu S, Zhu D, Zhang Q. Predicting hybrid performance in rice using genomic best linear unbiased prediction. Proc Natl Acad Sci U S A. 2014; 111(34):12456–12461.
- Longin C, Mühleisen J, Maurer H, et al. Hybrid breeding in autogamous cereals. Theor Appl Genet. 2012; 125(6):1087–1096.
- Titan P, Iskra J. Interspecific crosses involving Rf3 gene carriers as potential sources of R lines for D2 type cytoplasm based hybrid wheat system. Cereal Res Commun. 2021;:1–10.
- Chang Z, Chen Z, Wang N, et al. Construction of a male sterility system for hybrid rice breeding and seed production using a nuclear male sterility gene. Proc Natl Acad Sci U S A. 2016; 113(49):14145–14150.
- Ru ZG, Zhang LP, Hu TZ, et al. Genetic analysis and chromosome mapping of a thermo-sensitive genic male sterile gene in wheat. Euphytica. 2015; 201(3):321–327.
- Bai JF, Wang YK, Wang P, et al.. Uncovering male fertility transition responsive mirna in a wheat photo-thermosensitive genic male sterile line by deep sequencing and degradome analysis. Front Plant Sci. 2017; 8:1370.
- Heo JB, Sung S. Vernalization-mediated epigenetic silencing by a long intronic noncoding RNA. Science. 2011;331(6013):76–79. doi:10.1126/science.1197349. 21127216
- Liu J, Wang H, Chua N-H. Long noncoding RNA transcriptome of plants. Plant Biotechnol J. 2015;13(3):319–328. doi:10.1111/pbi.12336. 25615265
- Huang L, Dong H, Zhou D, et al. Systematic identification of long non-coding RNAs during pollen development and fertilization in Brassica rapa. Plant J. 2018; 96(1):203–222.
- Sablok G, Zhao H, Sun X. Plant circular RNAs (circRNAs): transcriptional regulation beyond miRNAs in plants. Mol Plant. 2016;9(2):192–194.
- Pan T, Sun X, Liu Y, et al. Heat stress alters genome-wide profiles of circular RNAs in arabidopsis. Plant Mol Biol. 2018; 96(3):217–229.
- Kaneko M, Inukai Y, Ueguchi-Tanaka M, et al. Loss-of-function mutations of the rice GAMYB gene impair alpha-amylase expression in aleurone and flower development. Plant Cell. 2004;16(1):33–44.
- Ding J, Lu Q, Ouyang Y, et al. A long noncoding RNA regulates photoperiod-sensitive male sterility, an essential component of hybrid rice. Proc Natl Acad Sci U S A. 2012; 109(7):2654–2659.
- Yuan SH, Bai JF, Guo HY, et al. QTL mapping of male sterility-related traits in a photoperiod and temperature-sensitive genic male sterile wheat line BS366. Plant Breed. 2020; 139(3):498–507.
- Kim D, Langmead B, Salzberg SL. HISAT: a fast spliced aligner with low memory requirements. Nat Methods. 2015; 12(4):357–360.
- Trapnell C, Pachter L, Salzberg SL. TopHat: discovering splice junctions with RNA-Seq. Bioinformatics. 2009; 25(9):1105–1111.
- Trapnell C, Roberts A, Goff L, et al. Differential gene and transcript expression analysis of RNA-seq experiments with TopHat and Cufflinks. Nat Protoc. 2012; 7(3):562–578.
- Kong L, Zhang Y, Ye Z-Q, et al. CPC: assess the protein-coding potential of transcripts using sequence features and support vector machine. Nucleic Acids Res. 2007;35(Web Server issue):W345–349.
- Sun L, Luo H, Dechao B, et al. Utilizing sequence intrinsic composition to classify protein-coding and long non-coding transcripts. Nucleic Acids Res. 2013;41(17):e166.
- Wang L, Park HJ, Dasari S, et al. CPAT: Coding-Potential Assessment Tool using an alignment-free logistic regression model. Nucleic Acids Res. 2013; 41(6):e74.
- Gao Y, Wang J, Zhao F. CIRI: an efficient and unbiased algorithm for de novo circular RNA identification. Genome Biol. 2015;16:4.
- Huang DW, Sherman BT, Lempicki RA. Systematic and integrative analysis of large gene lists using DAVID bioinformatics resources. Nat Protoc. 2009; 4(1):44–57.
- Liu S, Wu L, Qi H, et al. LncRNA/circRNA–miRNA–mRNA networks regulate the development of root and shoot meristems of Populus. Ind Crop Prod. 2019; 133:333–347.
- Pasquinelli AE. MicroRNAs and their targets: recognition, regulation and an emerging reciprocal relationship. Nat Rev Genet. 2012; 13(4):271–282.
- Livak KJ, Schmittgen TD . Analysis of relative gene expression data using real-time quantitative PCR and the 2(-Delta Delta C(T)) method. Methods. 2001; 25(4):402–408.
- Xiang L, Cai C, Cheng J, et al. Identification of circularRNAs and their targets in gossypium under verticillium wilt stress based on RNA-seq. Peer J. 2018; 6:e4500.
- Liu T, Zhang L, Chen G, et al. Identifying and characterizing the circular RNAs during the lifespan of arabidopsis leaves. Front Plant Sci. 2017; 8:1278.
- Wang J, Yang Y, Jin L, et al. Re-analysis of long non-coding RNAs and prediction of circRNAs reveal their novel roles in susceptible tomato following TYLCV infection. BMC Plant Biol. 2018;18(1):104.
- Chen M, Chory J. Phytochrome signaling mechanisms and the control of plant development. Trends Cell Biol. 2011; 21(11):664–671.
- Solís MT, Chakrabarti N, Corredor E, et al. Epigenetic changes accompany developmental programmed cell death in tapetum cells. Plant Cell Physiol. 2014; 55(1):16–29.
- Baxter A, Mittler R, Suzuki N. ROS as key players in plant stress signalling. J Exp Bot. 2014; 65(5):1229–1240.
- Shadel GS, Horvath TL. Mitochondrial ROS signaling in organismal homeostasis. Cell. 2015; 163(3):560–569.
- Liu Z, Shi X, Li S, et al. Oxidative stress and aberrant programmed cell death are associated with pollen abortion in isonuclear alloplasmic male-sterile wheat. Front Plant Sci. 2018; 9:595.
- Sun W, Hui XX, Lu X, et al. The rice phytochrome genes, PHYA and PHYB, have synergistic effects on anther development and pollen viability. Sci Rep. 2017;7(1):6439.
- Yang K-Z, Xia C, Liu X-L, et al. A mutation in thermosensitive male sterile 1, encoding a heat shock protein with DNAJ and PDI domains, leads to thermosensitive gametophytic male sterility in arabidopsis. Plant J. 2009; 57(5):870–882.
- Takano M, Inagaki N, Xie X, et al. Distinct and cooperative functions of phytochromes A, B, and C in the control of deetiolation and flowering in rice. Plant Cell. 2005; 17(12):3311–3325.
- Gu J-W, Liu J, Xue Y-J, et al. Functions of phytochrome in rice growth and development. Rice Sci. 2011; 18(3):231–237.
- Sheoran IS, Saini HS. Drought-induced male sterility in rice: Changes in carbohydrate levels and enzyme activities associated with the inhibition of starch accumulation in pollen. Sexual Plant Reprod. 1996; 9(3):161–169.
- Castro AJ, Clément C. Sucrose and starch catabolism in the anther of Lilium during its development: a comparative study among the anther wall, locular fluid and microspore/pollen fractions. Planta. 2007; 225(6):1573–1582.
- Li WL, Liu Y, Douglas CJ . Role of glycosyl transferases in pollen wall primexine formation and exine patterning. Plant Physiol. 2017; 173(1):167–182. 02016.
- Ariizumi T, Toriyama K. Genetic regulation of sporopollenin synthesis and pollen exine development. Annu Rev Plant Biol. 2011; 62(1):437–460.
- Quilichini TD, Grienenberger E, Douglas CJ. The biosynthesis, composition and assembly of the outer pollen wall: a tough case to crack. Phytochemistry. 2015;113(2):170–182.