Abstract
This study examined the effect of lactulose, galactooligosaccharide, fructooligosaccharide, inulin, and β-glucan on the probiotic strain Lactobacillus delbrueckii subsp. bulgaricus L14, cultivated in an in vitro gastrointestinal system model. We analyzed the degree of hydrolysis of the studied prebiotic oligosaccharides in condition of simulated gastric fluid. The results showed that lactulose had the highest resistance, galactooligosaccharide underwent hydrolysis, and fructooligosaccharide was the most sensitive. Among the polysaccharides, fructose was released from inulin and glucose from β-glucan. Short-chain oligosaccharides and metabolites derived from studied prebiotic oligosaccharides and polysaccharides, supported the growth of probiotic strain L14, which showed the highest growth with fructooligosaccharides and β-glucan as carbohydrate sources. The profile of the activated enzymes secreted by the probiotic strain L14, indicated their inducible character. Beta-galactosidase was activated in the presence of lactulose and GalOS, inulinase was activated in the presence of inulin and fructooligosaccharides, and β-glucosidase was activated in the presence of β-glucan fragments. Analysis of the produced organic and short-chain fatty acids showed that the typical representative of the homofermentative lactobacilli Lb. delbrueckii subsp. bulgaricus L14 changes its metabolism from a homofermentative to a heterofermentative type, best expressed in the presence of lactulose, galactooligosaccharide, and β-glucan.
Introduction
In the context of resolving the health problems of modern society, many scientific reports have postulated the positive effects of prebiotics in combating physiological disorders caused by oxidative stress [Citation1–3]. The mechanism of action of prebiotics is related to the growth and development of beneficial microflora in the host gastrointestinal tract and production of short-chain fatty acids (SCFAs) [Citation4–6]. They support the normal functioning of the intestinal mucosa, stimulate the immune system by suppressing the production of pro-inflammatory cytokines, and improve glucose homeostasis, etc. [Citation6]. There are different types of prebiotics, but mainly they are of carbohydrate origin and are oligosaccharides. The properties of prebiotics depend on their monosaccharide composition, the type of glycosidic bonds, the degree of polymerization (DP), and the degree of methylation. The most common monosaccharides in the composition of oligosaccharides are fructose, xylose, glucose, and galactose [Citation7].
Lactulose is a disaccharide of galactose and fructose and can be obtained by isomerization of lactose. In 1957 it was proved that lactulose possesses prebiotic features and promotes growth of fecal bacteria from the genus Bifidobacterium and for this reason, until the present day, lactulose is known as a ‘bifidogenic factor’ [Citation8, Citation9]. Lactulose has been used in the treatment of constipation, hepatic encephalopathy, and chronic kidney disease etc. [Citation10–12]. Low-dose lactulose can be used as a prebiotic [Citation9, Citation13].
Galactooligosaccharides (GalOS) are composed of galactose moieties with glucose or galactose attached at the reducing end. Commercial GalOS are produced by enzymatic transgalactosylation of glucose, galactose or lactose attached via β-(1, 2, 3, 4, or 6) linkages, with a DP 2 to 8 [Citation14].
Fructooligosaccharides (FOS) and inulin belong to the group of fructans. Inulin is a polydisperse fructan composed of fructose residues linked by β-(2-1) linkages. It has a varying DP from 2 to 60. Each chain has a terminal glucose molecule linked by α-(1-2) linkage [Citation7, Citation14]. Unlike inulin, FOS are composed of oligomers with shorter chain with a DP between 2 and 10 and can be produced by hydrolysis of inulin using endoinulinases [Citation14].
Beta-glucans are complex polysaccharides comprised of glucose units linked together by (β-1,3), (β-1,4) or (β-1,6) glycosidic bonds [Citation15]. They are found mainly in plants and provide diversity of beneficial effects altering lipid and glucose metabolism, anti-inflammatory, reducing cholesterol, cardiovascular and diabetes risk [Citation16, Citation17].
According to the Food and Agriculture Organization/World Health Organization and updated by Hill et al. probiotics are defined as ‘living microorganisms that, when administered in adequate amount, confer a health benefit on the host’, and include both bacteria (Lactobacillus, Bifidobacterium etc.) and yeasts (e.g. Saccharomyces) genera [Citation18, Citation19]. In recent years, there has been growing interest in studying how the gut microbiota impact the human health and its functions in the collaboration with the protective mechanisms of the host’s immune system against unfavorable effect of pathogens [Citation20, Citation21]. A lot of environmental factors, including antibiotics, disease, type of diet, as well as the mode of delivery and early feeding can influence the human microbiome, and the effects of prebiotic supplementation [Citation6].
The assimilation of prebiotic oligosaccharides and their metabolism by the beneficial microflora of the gastrointestinal tract is an individual factor due to the personal nature of the human microbiome and is also influenced by environmental factors. The study of the possibilities for prevention of dysbiosis in the gastrointestinal tract, as well as recovery of existing dysbiosis through the application of probiotics/prebiotics and symbiotics is a key factor in maintaining the health status of a person. The limited possibilities for in vivo experiments with the participation of volunteers have led to the development of various models of in situ analyses in conditions simulating in vitro gastrointestinal tract (GIT) systems. Data from such experiments would provide much more realistic information than strictly laboratory experiments, about the interaction of probiotics and prebiotic oligosaccharides under conditions of GIT, including their uptake, metabolism, and production of metabolites by the probiotic bacteria.
In this study, we explored the effect of lactulose, GalOS, FOS, inulin, and β-glucan on the probiotic strain Lactobacillus delbrueckii subsp. bulgaricus L14, after transformation in different parts of the GIT using simulated conditions in an in vitro gastrointestinal system model.
Materials and methods
Bacterial strain
To study the prebiotic potential, we used Lb. delbrueckii subsp. bulgaricus L14 strain from the collection of the Department of General and Applied Microbiology at Sofia University, Bulgaria. The strain was cultured for 24h on MRS (de Mann Rogosa Sharpe broth, Merck, Germany) media [Citation22] at 37 °C.
Prebiotic oligosaccharide and polysaccharides
In this study, we used the following oligosaccharides and polysaccharides: lactulose (Calbiochem, USA) contained lactulose 97.5%, galactooligosaccharide (GalOS) (Yakult, Japan) contained 2% DP2, 48% DP3, 38% DP4, and 12% DP5, fructooligosaccharide (FOS) (Orafti, Belgium) contained 5% glucose, fructose, and sucrose, inulin (Orafti, Belgium), and β-glucan (Orafti, Belgium).
In vitro gastro-intestinal digestion model
The in vitro model simulating gastrointestinal digestion was performed according to the INFOGEST method [Citation23]. Our experiment included simulated gastric fluid (SGF) and simulated intestinal fluid (SIF). Schematic representation of our experiment is shown in .
Figure 1. Scheme of simulated gastrointestinal digestion model involving simulated gastric fluid and simulated intestinal fluid and performed analysis.
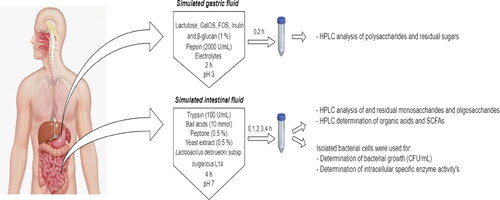
Bioreactors were sterilized in an autoclave at 121 °C for 20 min. Oligosaccharides and polysaccharides with a concentration between 10 and 15 mg/mL in sterilized water were prepared. For SGF, solutions of prebiotics, porcine pepsin solution (final concentration of 2000 U/mL), electrolytes composed by KCl (final concentration of 6.9 mmol/L, KH2PO4 (final concentration of 0.9 mmol/L), NaHCO3 (final concentration of 25 mmol/L), NaCl (final concentration of 47.2 mmol/L), MgCl2 x (H2O)6 (final concentration of 0.12 mmol/L) and (NH4)2CO3 (final concentration of 0.5 mmol/L) were mixed. Water was added to a final solution volume of 400 mL with pH 3.0. The mixture was incubated at 37 °C with continuous agitation (100 rpm) using a Winpact FS-05 system (Major Science, Taiwan) equipped with 500 mL vessels (Cat. FS-V-AS5). Samples were collected at 0 and 2 h.
For SIF to be obtained, the remaining SGF solutions were mixed with dissolved porcine trypsin solution (100 U/mL), peptone and yeast extract solution in a concentration of 5 mg/mL (0.5% w/v) and bile salts in a concentration of 10 mmol/L. The pH value was adjusted to 7.0 with 1 mol/L NaOH. Finally, activated bacterial culture of Lb. delbrueckii subsp. bulgaricus L14 was added. Bacterial cells were cultured for 24 h on MRS at 37 °C, to cell growth of 1x106 CFU/mL. After that, the biomass was removed by centrifugation at 5000 rpm (MPW-351R refrigerated Laboratory Centrifuge, Germany) for 20 min and washed once with sterile saline (0.9% NaCl) solution. The cells were inoculated in bioreactors at a concentration 103–104 CFU/mL. Samples were taken at 0, 1, 2, 3, and 4 h of fermentation reaction.
Microbial growth
The samples after collection from the bioreactors were centrifuged at 9000 rpm for 20 min at 4 °C. Supernatants were used for analysis of residual sugars and metabolites. Bacterial cells were washed twice with 0.05 mol/L sodium acetate buffer pH 7.5 and centrifuged.
Bacterial growth was measured by determination of colony-forming units per milliliter (CFU/mL). Bacterial cells grown in MRS (De Man, Rogosa, and Sharpe) broth (Merck) with 2% agar-agar for 24 h at 37 °C. They were seeded with Eddy jet 2 W- spiral plater system (IUL, Spain) using 50 µL of sample. Colony-forming units per milliliter were defined on automatic colony counter Sphere Flash (IUL, Spain).
Analysis of carbohydrates
Similar to our earlier study [Citation24], the monosaccharide and oligosaccharide composition was determined by a Shimadzu HPLC system (Shimadzu Corp., Japan) linked with Nexera X2, SIL-30AC autosampler, CTO-20AC thermostat and a RID-20A detector Shimadzu (Shimadzu Corp., Japan). Ten microliters of sample were injected and eluted into a Tracer 5 µm 15 × 0.46 column (Tecknohroma, Spain), acetonitrile:water (65:35, v/v) mobile phase, flow rate of 0.78 mL/min and temperature of 35 °C. The results were analyzed with LabSolution, Nexera-XR-RF software. The registered peaks of the samples were estimated using reference monosaccharide (Monosaccharides Kit, Sigma-Aldrich, cat. No. 47267), kestose (Sigma-Aldrich, cat. No. 72555) and oligosaccharide standards (lactulose, Calbiochem, cat. No.427584-50GM; kestotetraose, Megazym, cat. No. O-KTE; kestopentaose, Megazym, cat. No. O-KPE; galactobiose, Megazym, cat. No. O-GBI).
Analysis of metabolites
As we have described before [Citation24] organic and SCFAs were identified by a Konik-Tech HPLC system (Konik, Barcelona, Spain), with a UV Detector (Konik-tech, λ = 210 nm). An Aminex HPX-87H (Bio-Rad, USA) 5 μm column (250 × 4.6 mm) was used, isocratic mobile phase containing 0.005 mol/L H2SO4, flow rate of 0.6 mL/min, column temperature of 40 °C. The identification of the peaks was performed based on the retention times compared to the standards of organic and short-chain fatty acids (lactic acid, Sigma-Aldrich, cat. No. 252476-100 mL; acetic acid, Sigma-Aldrich, cat. No. 33209-2.5 L; propionic acid, Fluka, cat. No. P1386- 1 L; butyric acid, Sigma-Aldrich, cat. No. B103500-500 mL).
Enzyme analysis
For enzyme activity assays, Lb. delbrueckii subsp. bulgaricus L14 cells were collected by centrifugation and lysed with 1 mL disintegrating buffer (containing 50 mmol/L sodium acetate buffer pH 7.5, 30 mmol/L NaCl and 2% glycerol) in a UP 50 H Ultrasonic Processor (Hielscher, Ultrasoud Technology, Germany). Then, samples were centrifuged, and the supernatants were used to measure the activity of α-galactosidase, β-galactosidase, α-glucosidase, β-glucosidase, and inulinase.
The α-galactosidase activity of Lb. delbrueckii subsp. bulgaricus L14 was determined by the method of Petek et al. (1969) [Citation25] with slightly changes done by us in our previous study [Citation24], as the amount of p-nitrophenol (pNP) released by the degradation of pNP-α-D-galactopyranoside substrate (Sigma-Aldrich). The reaction mixture contained 250 μL of 5.5 mmol/L pNP-α-D-galactopyranoside substrate in 50 mmol/L KH2PO4 buffer (pH 6.8) and 100 μL of the bacterial lysate. The total volume was brought up to 450 μL with distilled water and incubated for 20 min at 37 °C. The reaction was stopped by the addition of 2 mL of 1 mol/L Na2CO3. The amount of the released pNP was measured spectrophotometrically at 405 nm.
The β-galactosidase activity was determined by the method of Lim & Chae (1989) [Citation26], based on the amount of o-nitrophenol (oNP) released by the degradation of oNP-β-D-galactopyranoside substrate (Sigma-Aldrich). The reaction mixture contained 250 μL of 5.5 mmol/L oNP-β-D-galactopyranoside substrate in 50 mmol/L KH2PO4 buffer (pH 6.8), 100 μL of the bacterial lysate and 100 μL distilled water. The mixture was incubated for 20 min at 37 °C. The reaction was stopped by the addition of 2 mL of 1 mol/L Na2CO3. The amount of the released oNP was measured spectrophotometrically at 405 nm.
The α-glucosidase activity was assayed by the method of Dewi et al. (2007) [Citation27] with some adjustments made in our earlier experiment [Citation24], based on the amount of pNP released by the degradation of pNP-α-D-glucopyranoside substrate (Sigma-Aldrich). The reaction mixture included 250 μL of 5.5 mmol/L pNP-α-D-glucopyranoside substrate in 50 mmol/L KH2PO4 buffer (pH 6.8) and 100 μL of the bacterial lysate. The total volume was brought up to 450 μL with distilled water and was incubated for 20 min at 37 °C. The reaction was stopped by the addition of 2 mL of 1 mol/L Na2CO3. The amount of the released pNP was measured spectrophotometrically at 405 nm.
The β-glucosidase activity of probiotic strain Lb. delbrueckii subsp. bulgaricus L14 was determined by the method of Martin & Akin (1988) [Citation28], based on the amount of pNP released by the degradation of pNP-β-D-glucopyranoside substrate (Sigma-Aldrich). The reaction mixture was composed of 250 μL of 5.5 mmol/L pNP-β-D-glucopyranoside substrate in 50 mmol/L KH2PO4 buffer (pH 6.8) and 100 μL of the bacterial lysate. The total volume was brought up to 450 μL with distilled water and incubated for 20 min at 37 °C. The reaction was stopped by the addition of 2 mL of 1 mol/L Na2CO3. The amount of the released pNP was measured spectrophotometrically at 405 nm.
The inulinase activity was determined via a modified method of Miller (1959) [Citation29], by the amount of fructose released from degradation of the 0.2% inulin substrate in 10 mmol/L acetate buffer (pH 4.6). The reaction mixture was composed of 2 mL of 0.2% inulin substrate, 2 mL of acetate buffer (10 mmol/L, pH 4.6) and 0.5 mL of bacterial lysate. The mixture was incubated for 20 min at 37 °C. The enzymatic reaction was stopped by boiling for 10 min. The reaction mixture was analyzed to determine the amount of fructose by the DNSA method [Citation29]. The amount of released fructose was measured spectrophotometrically at 575 nm.
The protein content in all samples was determined by the method of Bradford (1976) [Citation30] using bovine serum albumin (Sigma-Aldrich) as a standard.
Spectrophotometric analyzes were performed on a Beckman Coulter DU 800 spectrophotometer (USA), and all experiments were performed in triplicate.
Statistical analysis
Microsoft Excel statistical package was used for data analysis and graphical representation. Data from performed experiments were expressed as mean values ± SD.
Results and discussion
Structural changes of prebiotic oligosaccharides and polysaccharides in condition of SGF
The first step in our experiments was to analyze the degree of hydrolysis of several prebiotic oligosaccharides (lactulose, GalOS, and FOS), as well as the polysaccharides inulin and β-glucan in condition of SGF. The changes in the carbohydrate composition in the SGF were reported by comparing the results at 0 and 2 h of incubation ().
Figure 2. Degree of the hydrolysis of studied prebiotic oligosaccharides and polysaccharides in conditions simulating gastric fluid after period of 2 h (1% lactulose (A), 1% GalOS (B), 1% FOS (C), 1% inulin (D), and 1% β-glucan (E)).
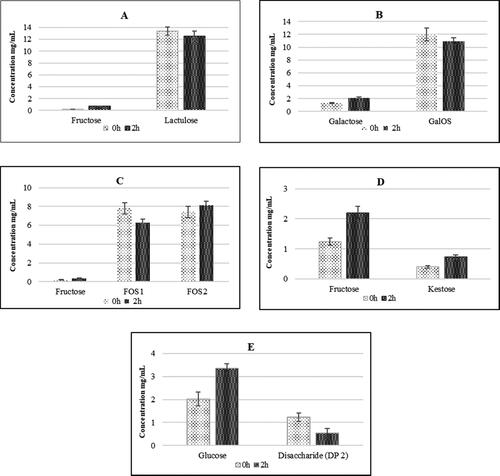
Lactulose underwent partial hydrolysis after 2 h, with detection of fructose, as a result of the rupture of the glyosidic bond between galactose and fructose (). Partial hydrolysis of GalOS and an increase of 30% in the amount of galactose was detected after 2 h of treatment (). However, GalOS showed a high degree of resistance to SGF, which preserves their structure and prebiotic potential. In the next experiment, with the participation of FOS with different degrees of polymerization (DP 4 and 6), there was limited hydrolysis of FOS1 (DP 4) due to increased fructose content. FOS2 (DP 6) was more resistant to hydrolysis under the studied conditions (). Our results showed that the inulin-type FOS we used are relatively less resistant to the acidic conditions of gastric juice, but the relatively short exposure time protects them from hydrolysis.
Inulin type of polysaccharide showed more than 70% resistance in SGF conditions during 2h of treatment. Our results showed that in acidic pH, such as in the stomach, fructose, and the trisaccharide kestose were released. Their levels increased proportionally to the duration of the hydrolytic process (). It is known that in acidic conditions (at pH < 4) the structure of inulin splits into separate fructose units and inulin-type fructooligosaccharides [Citation31, Citation32]. A previous study has demonstrated the specificity of the probiotics strain in inulin utilization [Citation33].
Beta-glucan showed relatively good resistance to gastric juice during an incubation period of 2 h (). This, on the one hand, provides information for the possible activation of the small intestine microbiome, but, on the other hand, preserves the immune-stimulating effect of β-glucan reported by a number of authors [Citation34, Citation35].
Structural changes of prebiotic oligosaccharides and polysaccharides in condition of SIF
In the conditions of SIF, only 34% of the initial quantity of lactulose was hydrolyzed after 4 h of incubation (). Mainly fructose units from lactulose were consumed by the probiotic strain Lb. delbrueckii subsp. bulgaricus L14. The reason for the observed changes can be sought not only in the altered physiological conditions, but also in stimulation of the metabolism of present lactic acid bacteria, part of the beneficial microflora.
Table 1. Residual oligosaccharide concentrations and percent of their hydrolysis obtained after 1% lactulose, GalOS and FOS fermentation in conditions simulating SIF in the presence of Lb. delbrueckii subsp. bulgaricus L14.*
The dynamics of the utilization of GalOS in the SIF showed a drastic reduction of oligosaccharides by more than 70% after 4 h of incubation (). The gradual increase in the concentration of galactose is probably the result of poor utilization by the probiotic strain L14 present in the in vitro system (). The studied GalOS contain fractions with different degrees of polymerization from 4 to 8. On the one hand, the obtained results show that GalOS is significantly more hydrolyzed in SIF conditions, in the presence of Lb. delbrueckii subsp. bulgaricus L14. The probable reason is the stimulation of the production of the enzyme β-galactosidase by the probiotic strain, which hydrolyzes the glycosidic bonds in the structure of GalOS releasing galactose units. On the other hand, the increased concentration of galactose at the end of the incubation period indicates that the strain has difficulty absorbing this carbohydrate in the first 4 h of incubation. GalOS are one of the first oligosaccharides with a proven prebiotic effect. They are synthesized as a result of a transgalactosidase reaction in the presence of lactose in varying concentrations [Citation36, Citation37]. They have different degrees of polymerization, but are very similar in structure [Citation38, Citation39]. GalOS can significantly stimulate the growth of Bifidobacterium and Lactobacillus [Citation40].
Table 2. Residual monosaccharide concentrations obtained after 1% lactulose, GalOS and FOS fermentation in conditions simulating SIF in the presence of Lb. delbrueckii subsp. bulgaricus L14.*.
Our results demonstrated that 18% of FOS2 with DP 6 and 8% of FOS1 with DP 4 were hydrolyzed in the studied conditions of SIF (). The obtained fructose was absorbed by strain L14 more clearly on the 4th hour () under conditions of SIF. It is most likely that this probiotic strain secretes inulinases that hydrolyze the glycosidic bonds in the FOS.
The metabolism of inulin under conditions simulating small intestines showed that the hydrolytic process continued and the level of fructose increased due to hydrolysis of the present kestose end inulin (). The explanation for the changes in the structure of inulin probably lies in the secretion of hydrolytic enzymes, particularly inulinase from the probiotic strain Lb. delbrueckii subsp. bulgaricus L14.
Table 3. Residual monosaccharide and oligosaccharide concentrations obtained after 1% inulin and β-glucan fermentation in conditions simulating SIF in the presence of Lb. delbrueckii subsp. bulgaricus L14.*
Beta-glucan is a high-molecular weight polysaccharide that is difficult to hydrolyze by the enzymes in the GIT. Its partial hydrolysis, which was observed in stomach simulating conditions, benefits the growth of the intestinal microbiota. In conditions simulating the processes in the small intestine, the hydrolysis and absorption of the glucose units obtained from the β-glucan and other oligosaccharides was confirmed. The glucose levels increased by 29% on average within 4 h in conditions imitating SIF. This most probably results from the action of glucohydrolases secreted by the respective lactic acid bacteria. For the same reason there was an increase in the quantity of disaccharides of the cellobiose type cleaved from the main β-glucan chain. Larger oligosaccharide fragments were not reported ().
Utilization and metabolism of prebiotics by Lb. delbrueckii subsp. bulgaricus L14 in SIF
Growth kinetics of Lb. delbrueckii subsp. bulgaricus L14
During the cultivation of Lb. delbrueckii subsp. bulgaricus L14 in medium supplemented with 1% of lactulose as a sole carbohydrate source, we observed weak growth from 0 h (3.8 × 105 log CFU/mL) to 2 h (4 × 105 log CFU/mL) followed by a decrease to 3 × 105 log CFU/mL. This could be due to the consumption of the free fructose released by acidic hydrolysis during the SGF phase. When this fructose is depleted, the cell growth starts do decline.
As a result of the partial hydrolytic processes of GalOS in conditions of SGF, monosaccharide and disaccharide residues were obtained. They were easily digested by the probiotic strain L14 used in the first 2 h of incubation. Their depletion leads to a reduction in cell growth and its maintenance at a low level for up to 4 h of the process ().
Figure 3. Dynamics of cell growth of Lb. delbrueckii subsp. bulgaricus L14 strain, in the presence of different oligosaccharides in conditions simulating the small intestine fluid.
*Values are means of three measurements ± SD.
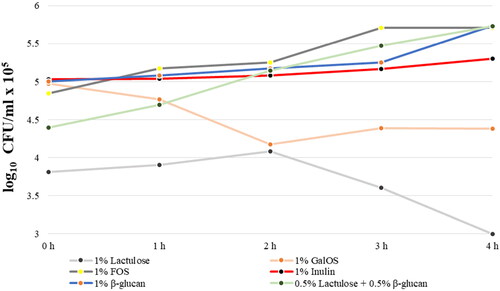
In the presence of FOS, the number of colonies increased from 4.8 to 5.3 log CFU/mL × 105 in the first 2 h in simulated small intestinal condition, followed by exponential growth to 5.7 × 105 log CFU/mL.
In conditions of SGF, partial hydrolysis of inulin occurred. Respectively, during the next step simulating the small intestine, strain L14 started to consume the available small amounts of fructose and FOS which are products of the hydrolysis during the SGF phase. During the SIF phase, fructose and FOS are consumed, which led to an increase in the cell biomass from 5 × 105 to 5.3 × 105 log CFU/mL after 4 h of incubation (). Moreover, the cell counts did not show a rapid decrease at the end of the cultivation period. This is probably due to the presence of undigested inulin, which is further involved in metabolic processes after utilization of absorbed fructose molecules and FOS in the first hours of cultivation.
In the presence of β-glucan, the CFU/mL values increased gradually from 5 × 105 log CFU/mL at the beginning of the SIF phase to 5.7 × 105 log CFU/mL at 4 h of cultivation (). In our experiment, in conditions simulating small intestines, the minimal amounts of glucose and cellobiose obtained in the in vitro stomach contributed to the adaptation of the inoculated probiotic strain in the first three hours of the incubation. The number of bacteria increased to 5.7 × 105 log CFU/mL at the fourth hour of the incubation (). The process of adaptation can be explained with induced glucanases responsible for hydrolysis of β-glucan.
The development of prebiotics as food supplements in search of maximum effect on the human intestinal microbiota arouses scientific interest in studying the combinatorial effects of two or more prebiotics on the beneficial microflora. In our last experiment, we tested the effect of the combination of 0.5% lactulose and 0.5% β-glucan on the metabolism of the probiotic strain L14 under conditions simulating the small intestine. There was a gradual increase in the number of bacteria throughout the incubation period (). This can be explained by the presence of much more easily digestible monosaccharides, due to the hydrolytic processes in conditions that mimic the SGF. The final CFU/mL values (4 h, 5.7 × 105) did not exceed the ones with each of the prebiotics alone, which is probably related to the strain’s capacity to utilize carbohydrates.
Changes in enzyme activities of probiotic strain Lb. delbrueckii subsp. bulgaricus L14
In this set of experiments, we determined the enzyme activities of α-galactosidase, β-galactosidase, α-glucosidase, β-glucosidase, and inulinase from probiotic strain Lb. bulgaricus L14 produced in the presence of different carbohydrate sources (). In the presence of lactulose, β-galactosidase activity was detected until 3 h of cultivation (0.46 ± 0.05 U/mg). The activities of α-galactosidase (0.04 ± 0.009 U/mg) and α-glucosidase (0.03 ± 0.01 U/mg) were low at 3 and 2 h of incubation, respectively. All of the tested enzymes showed no detectable activity at 4 h of incubation. Nevertheless, a study of Fara et al. reported that Lb. delbrueckii subsp. bulgaricus CRL450 has high β-galactosidase activity and synthesizes GalOS from lactose and lactulose [Citation41]. When the purified oligosaccharide was tested, bacterial growth was decreased. However, the microorganisms demonstrated metabolic activity which was proved by carbohydrate consumption and lactate production [Citation41].
Table 4. Dynamics of changes in the specific activity of the enzymes from Lb. delbrueckii subsp. bulgaricus L14 in the presence of different oligosaccharides in conditions simulating the SIF.*
On the one hand, the basic knowledge of the enzyme activities of Lactobacillus, can lead to development of a new design of more effective probiotics, and on the other, it can also aim to identify potential enzymatic indicators of the main metabolic activity, when evaluating their influence on the gastrointestinal tract. By glycotechnology, prebiotics can be designed to contain certain monosaccharides and/or a combination of linkages that induce or enhance the specific glycosidase activity of probiotic strains of Lactobacillus or other probiotic microorganisms [Citation42, Citation43].
The activity of β-galactosidase showed a similar trend when the carbohydrate source was GalOS, with the highest activity after 4 h of cultivation in SIF condition (0.13 ± 0.08 U/mg). This probably resulted from induction of the enzyme by non-hydrolyzed GalOS present in the medium. On the other hand, data showed induction of α-glucosidase (0.2 ± 0.04 U/mg) by the third hour and absence of α-galactosidase activity ().
The prebiotic potential of FOS is due to the specific glycoside linkages between the fructose monomers, which are difficult to hydrolyze or not at all degradable by the enzymes secreted in the human GIT [Citation44]. The metabolic profile data of Lb. bulgaricus L14 in SIF conditions in the presence of 1% FOS showed an induction of inulinase activity after 2 h of incubation (0.05 U/mg protein) with a maximum at 3 h (0.2 U/mg protein) of incubation, whereas the activities of β-galactosidase, α-galactosidase and β-glucosidase were rather negligible.
In the presence of inulin in SIF, strain L14 showed weak inulinase activity, best manifested at 4 h of incubation (0.11 ± 0.01 U/mg). We explained this by the depletion of the minimum available amounts of easily digestible fructose and FOS and the presence of high molecular weight inulin, which requires hydrolysis by inulinase type enzymes. Inulin is a polyfructosan of plant origin [Citation45]. It belongs to the first generation of prebiotics and together with GalOS and FOS is the most researched prebiotic. The prebiotic effect of inulin is due to its β-(2-1) bonds, which remain indigestible by host enzymes. The change in the composition of the intestinal microflora by inulin and FOS is due to their selective fermentation by Bifidobacterium. They produce intracellular inulinase to hydrolyze the β-(2-1) bond [Citation14]. Along with this, it is known that under acidic conditions (at pH < 4) its structure is cleaved into single fructose units and inulin-type FOS. The strain specificity of probiotics in the utilization of inulin has also been demonstrated [Citation32]. These preliminary data and the missing information about the degree of inulin hydrolysis in simulated GIT conditions served as a basis for conducting these experiments.
With β-glucan, there was partial hydrolysis in SGF conditions, and this favors the growth of the microbiota in the intestinal tract. The data on β-glucosidase activity from the probiotic strain confirmed that the increase in cell growth was initially a consequence of the uptake of the available glucose units and subsequently of the hydrolysis of the available cellobiose. The studied strain showed high activity of β-glucosidase during the whole period of incubation in SIF (1.33 ± 0.18 U/mg at 4 h).
The results of the tested enzymes produced by Lb. bulgaricus L14 cultivated in medium with 0.5% lactulose and 0.5% β-glucan clearly showed induction of the production of β-galactosidase as a result of the presence of lactulose and β-glucosidase, responsible for the hydrolysis of β-glucan. The activity of β-galactosidase (5.72 ± 0.7 U/mg) was about 20 times higher than β-glucosidase (0.25 ± 0.05 U/mg) (). This can be explained by the much more accessible molecule of lactulose for utilization from the studied strain.
According to Tzortzis et al. (2004) [Citation42], the increased activities of specific enzymes in probiotic bacteria is associated with faster and more efficient hydrolysis of prebiotics. Selective growth stimulation in in vitro conditions does not necessarily lead to an increased number in Bifidobacterium or introduced probiotic strains of Lactobacillus in vivo [Citation46]. A prebiotic can increase the metabolic activity of microorganisms from the beneficial microbiota, as well as the added probiotics, without increasing the microbial number in the colon. It is important to understand what enzymes are induced or enhanced during the utilization of certain carbohydrates, acting as a biomarker of overall metabolic activity in the colon when prebiotic is ingested. In their study, Tannock et al. (2004) [Citation46] postulated that β-galactosidase could be used as a biomarker of metabolic activity in the colon to assess the impact of prebiotics in vivo. However, β-galactosidase may not be a suitable biomarker for all potential prebiotics and in particular for α-linked glucooligosaccharides.
Production of organic acids and SCFAs
When cultivated on different oligosaccharides, the studied strain produced different amounts of lactic, acetic and butyric acid ().
Table 5. Organic and short-chain fatty acids concentrations obtained after utilization of different oligosaccharides in conditions simulating SIF in the presence of Lb. delbrueckii subsp. bulgaricus L14.
In the presence of 1% lactulose in the medium, probiotic strain L14 metabolized mainly fructose and partially galactose to D-lactate (17.7 ± 1.68 mmol/L) after 1 h of cultivation. The ratio of D-lactic/L-lactic acid was 17:5, which remained within the same ranges to the end of the experiment. An important point is the production of acetate at 2 h of cultivation (about 1.6 mmol/L), which increased to 14.93 ± 1.29 mmol/L at 4 h. A very low concentration of butyrate was measured at 3 h. The results showed that even in SIF conditions, the typical representative of the homofermentative lactobacilli Lb. delbrueckii subsp. bulgaricus L14 changed its metabolism from a homofermentative to a mixed type. The mechanism inducing the metabolic shift to mixed fermentation is not elucidated in details, and most likely this shift can be identified by studying the expression of genes responsible for the synthesis of specific enzymes of the metabolic pathways [Citation47]. Fasting can be one of the factors that change the metabolism from homofermentative to mixed type [Citation48, Citation49].
The profile of D/L-lactic acids and SFCAs production during the metabolism of GalOS differed from those of lactulose. At the beginning of the cultivation, mainly acetate and a small quantity of L-lactate were produced. After that, the quantity of D-lactate rapidly increased and acetate was non-detectable. Again heterofermentative type of metabolism was observed, but the ratio of D-and L-lactate/acetate was changed.
When monitoring the final metabolites obtained as a result of cultivation of strain L14 in the presence of FOS, the main metabolite was D-lactate. In limited amounts, butyrate was detected in the first two hours of cultivation, which is probably due to activated metabolic processes in the inoculated probiotic strain. Fructans are widely used as a food additive, as well as in combination with probiotics, resulting in a synergistic, and symbiotic effect. Their physiological effects depend on the length of the polysaccharide chain. Fructooligosaccharides are metabolized faster and lead to the production of more butyrate compared to inulin [Citation14, Citation50], which was also observed in our experiment. No production of acetate was detected during the cultivation in FOS medium.
There is evidence from previous studies that various short-chain carbohydrates with different monosaccharide composition and molecular weights are also metabolized by the major bacterial species inhabiting the animal and human GIT [Citation51]. Fructans are fermented very well by Clostridium, and very few species are able to ferment oligosaccharides and polysaccharides with complex structures, such as xylooligosaccharides, arabinoxylan oligosaccharides and pectin oligosaccharides. Bacteria from Bifidobacterium use carbohydrates with low DP first, and Bacteroides first use those with a higher degree of polymerization [Citation51]. Therefore, the structure of carbohydrates and the bacterial species present in the intestinal ecosystem are probably an important factor in controlling their fermentation and obtaining metabolites important for human health status. Here, we must add the strictly individual nature of the microbiome in the human GIT. Kaplan and Hutkin’s study involving 28 species of lactic acid bacteria (LAB) showed that they have the ability to metabolize FOS, 12 of 16 species of Lactobacillus and 7 of 8 species of Bifidobacterium were capable of fermenting FOS, and 8 of the studied Enterobacteriaceae did not metabolize FOS [Citation52]. The probiotic strain Lb. plantarum WCFS1 isolated from human saliva can metabolize various carbohydrates such as monosaccharides (glucose, galactose, and mannose), disaccharides (sucrose, lactose, and trehalose) and trisaccharides (raffinose and melezitose [Citation53].
The inulin metabolism demonstrated similar characteristics. The ratio between D-lactate and L-lactate was 10:1. A certain amount of short-chain fatty acid butyrate was observed at the beginning of the process, which was absent at later stages of cultivation. Additional metabolic transformations of the butyrate probably occur. It is noteworthy that the presence of inulin and its lower molecular weight derivatives initiate a typical homofermentative process in the studied strain L14.
Strain Lb. delbrueckii subsp. bulgaricus L14 again showed a change from homofermentative to heterofermentative metabolism during fermentation of 1% β-glucan. The ratio of D-lactate to L-lactate was about 3:1 at the third hour of the process. At the 4th hour, there was a drastic change in the profile of short-chain fatty acids with a predominance of L-lactate, the presence of acetate and very small amounts of D-lactate.
When cultivated on medium with 0.5% lactulose and 0.5% β-glucan, probiotic strain L14 showed more homofermentative metabolism with a ratio of D-lactate/L-lactate of 5:1.
Oligosaccharides promote the growth and development of LAB [Citation54, Citation55]. Hypothetically, those LAB in the host GIT, with their specific enzymes for utilization of prebiotic substrates and proper transport systems, have potential to compete with other microorganisms from the complex community in the intestinal tract.
The fermentation of dietary fibers and prebiotic oligosaccharides by intestinal bacteria leads to production of acetate, propionate and butyrate, which are SCFAs. They are absorbed in the intestine, where butyrate is used as an energy source for the epithelial cells of the colon, while the remaining SCFAs enter the portal circulatory system [Citation56].
Propionate is used in the liver as a substrate for lipids and glucose synthesis, whereas acetate is primarily utilized for the cholesterol synthesis in peripheral tissues [Citation57]. The absence of these SCFAs as an energy source in the colon is implicated in the pathogenesis of intestinal diseases [Citation58]. Previous studies demonstrated the beneficial role of Lactobacillus in the treatment of inflammatory bowel disease and ulcerative colitis due to its capability to stimulate the production of SCFAs [Citation59–61]. The mechanisms by which SCFAs perform their effects are associated with stimulation of secretion of mucin and protection of the intestinal epithelial barrier [Citation61, Citation62]. In the study of Scheppach at al. (1992) butyrate was used in the treatment of inflammation caused by ulcerative colitis and in those patients the concentrations of SCFAs were lower [Citation63]. Butyrate is involved in the regulation of gene expression as a consequence of chromatin remodeling, specifically inhibition of histone deacetylase [Citation64] and increased expression of the transcriptional coactivator PGC-1α (peroxisome proliferator-activated receptor-gamma coactivator), which is involved in the regulation of energy metabolism of the cell, carbohydrate and lipid metabolism, mitochondrial biosynthesis etc. [Citation65, Citation66]. Oxidation of butyrate occurs in the mitochondria of epithelial cells of the large intestine, which results in ATP production [Citation67]. Another mechanism for the impact of SCFAs on energy balance includes binding to G-protein coupled receptors (GPR) such as GPR41 and GPR43 expressed on the surface of intestinal enteroendocrine cells [Citation68]. As a result of this interaction, specific hormones like peptide YY (PYY) are secreted into the systemic circulation, providing a connection between the gut environment and the host [Citation56].
Conclusions
Data from our experiments provide much more realistic information about the interaction of probiotic strain Lb. delbrueckii subsp. bulgaricus L14 and prebiotic oligosaccharides including lactulose, GalOS and FOS, and polysaccharides inulin and β-glucan in in vitro simulation system of GIT, including their utilization, secretion of enzymes for hydrolysis of studied prebiotics and production of lactic acid, acetic acid, and SCFAs. We found that metabolites derived from prebiotic oligosaccharides and polysaccharides support the growth and development of probiotic strains of LAB stimulating the activity of different carbohydrolases. Furthermore, the specificity of the metabolism of the studied oligosaccharides and polysaccharides has been demonstrated. On the one hand, the ratio of D-lactic/L-lactic acid and, on the other hand, the ratio of lactic acid/acetic acid/butyric acid showed diverse values by utilization of different studied oligosaccharides and polysaccharides. Depending on the type of prebiotics and strain specificity, there is a change from the homofermentative pathway to a mixed metabolic pathway of absorption and utilization of monosaccharide components of prebiotics.
Author contributions
Conceptualization: Ivica Dimov and Ilia Iliev ; methodology: Ilia Iliev, Tonka Vasilieva, Veseiln Bivolarski and Mariana Nikolova; data curation : Ivica Dimov, Daniela Mollova, Mariana Nikolova ; formal analysis: Ivica Dimov, Daniela Mollova ; funding acquisition: Ilia Iliev; investigation: Ivica Dimov, Mariana Nikolova, Veselin Bivolarski and Tonka Vasileva; supervision: Anelia Bivolarska and Ilia Iliev; visualization: Ivica Dimov; writing: original draft: Ivica Dimov; writing - review & editing: Veselin Bivolarski and Ilia Iliev .
Data availability statement
All data that support the findings reported in this study are available from the corresponding author upon reasonable request.
Disclosure statement
The authors have no conflict of interest to disclose.
Additional information
Funding
References
- Kellow NJ, Coughlan MT, Savige GS, et al. Effect of dietary prebiotic supplementation on advanced glycation, insulin resistance and inflammatory biomarkers in adults with pre-diabetes: a study protocol for a double-blind placebo-controlled randomised crossover clinical trial. Of science ®] [google scholar]. BMC Endocr Disord. 2014;14:55.
- Gao J, Azad MAK, Han H, et al. Impact of prebiotics on enteric diseases and oxidative stress. Curr Pharm Des. 2020;26(22):2630–2641.
- Nguyen TTU, Kim HW, Kim W. Effects of probiotics, prebiotics, and synbiotics on uremic toxins, inflammation, and oxidative stress in hemodialysis patients: a systematic review and meta-analysis of randomized controlled trials. JCM. 2021;10(19):4456.
- Gibson GR, Roberfroid MB. Dietary modulation of the human colonic microbiota: introducing the concept of prebiotics. J Nutr. 1995;125(6):1401–1412.
- Hashmi A, Naeem N, Farooq Z, et al. Effect of prebiotic galacto-oligosaccharides on serum lipid profile of hypercholesterolemics. Probiotics Antimicro Prot. 2016;8(1):19–30.
- Gibson GR, Hutkins R, Sanders ME, et al. Expert consensus document: the international scientific association for probiotics and prebiotics (ISAPP) consensus statement on the definition and scope of prebiotics. Nat Rev Gastroenterol Hepatol. 2017;14(8):491–502.
- Barreteau H, Delattre C, Michaud P. Production of oligosaccharides as promising new food additive generation. Food Technol Biotechnol. 2006;44(3).
- Petuely F. Der bifidusfaktor. Dtsch med Wochenschr. 1957;82(46):1957–1960. (In German).
- Karakan T, Tuohy KM, Janssen-van Solingen G. Low-dose lactulose as a prebiotic for improved gut health and enhanced mineral absorption. Front Nutr. 2021;8:672925.
- TayebiKhosroshahi H, Habibzadeh A, Khoshbaten M, et al. Lactulose for reduction of nitrogen products in patients with chronic kidney disease. Iran J Kidney Dis. 2014;8(5):377–381.
- Pranami D, Sharma R, Pathak H. Lactulose: a prebiotic, laxative and detoxifying agent. Drugs Ther Perspect. 2017;33(5):228–233.
- Ruszkowski J, Witkowski JM. Lactulose: patient- and dose-dependent prebiotic properties in humans. Anaerobe. 2019;59:100–106.
- Mao B, Li D, Zhao J, et al. In vitro fermentation of lactulose by human gut bacteria. J. Agric. Food Chem. 2014;62(45):10970–10977.
- Enam F, Mansell TJ. Prebiotics: tools to manipulate the gut microbiome and metabolome. J Ind Microbiol Biotechnol. 2019;46(9–10):1445–1459.
- Yang J, Tu J, Liu H, et al. Identification of an immunostimulatory polysaccharide in banana. Food Chem. 2019;277:46–53.
- Gunness P, Michiels J, Vanhaecke L, et al. Reduction in circulating bile acid and restricted diffusion across the intestinal epithelium are associated with a decrease in blood cholesterol in the presence of oat β-glucan. FASEB J. 2016;30(12):4227–4238.
- Murphy EJ, Rezoagli E, Major I, et al. β-Glucan metabolic and immunomodulatory properties and potential for clinical application. JoF. 2020;6(4):356.
- Hill C, Guarner F, Reid G, et al. Expert consensus document. The international scientific association for probiotics and prebiotics consensus statement on the scope and appropriate use of the term probiotic. Nat Rev Gastroenterol Hepatol. 2014;11(8):506–514.
- Cuevas-González PF, Liceaga AM, Aguilar-Toalá JE. Postbiotics and paraprobiotics: from concepts to applications. Food Res Int. 2020;136:109502.
- Carding S, Verbeke K, Vipond DT, et al. Dysbiosis of the gut microbiota in disease. Microb Ecol Health Dis. 2015;26:26191.
- Farooqui T. Link between gut microbiome and cardiometabolic diseases. In: Gut microbiota in neurologic and visceral diseases. Cambridge: Academic Press. 2021. 185–205.
- De Man JC, Rogosa D, Sharpe ME. A medium for the cultivation of lactobacilli. J Appl Bacteriol. 1960;23(1):130–135.
- Brodkorb A, Egger L, Alminger M, et al. INFOGEST static in vitro simulation of gastrointestinal food digestion. Nat Protoc. 2019;14(4):991–1014.
- Dimov I, Mollova D, Vasileva T, et al. Effect of Polysaccharides Obtained from Plantago major L. leaves on Lactobacillus bulgaricus L14 in an In Vitro Model System of the Gastrointestinal Tract. Proceedings of the 5th Balkan Scientific Conference on Biology. p. 2021,25–34.
- Petek F, Villarroya E, Courtois JE. Purification et propriétés de l’alpha-galactosidase des graines germées de vicia sativa [purification and properties of the alpha-galactosidase of the germinating seeds of vicia sativa]. Eur J Biochem. 1969;8(3):395–402.
- Lim K, Chae CB. A simple assay for DNA transfection by incubation of the cells in culture dishes with substrates for beta-galactosidase. Biotechniques. 1989;7(6):576–579.
- Dewi RT, Iskandar YM, Hanafi M, et al. Inhibitory effect of koji Aspergillus terreus on alpha-glucosidase activity and postprandial hyperglycemia. Pak J Biol Sci. 2007;10(18):3131–3135.
- Martin SA, Akin DE. Effect of phenolic monomers on the growth and beta-glucosidase activity of Bacteroides ruminicola and on the carboxymethylcellulase, beta-glucosidase, and xylanase activities of Bacteroides succinogenes. Appl Environ Microbiol. 1988;54(12):3019–3022.
- Miller GL. Use of dinitrosalicylic acid reagent for determination of reducing sugar. Anal Chem. 1959;31(3):426–428.
- Bradford MM. A rapid and sensitive method for the quantitation of microgram quantities of protein utilizing the principle of protein-dye binding. Anal Biochem. 1976;72:248–254.
- Matusek A, Merész P, Le TKD, et al. Effect of temperature and pH on the degradation of fructo-oligosaccharides. Eur Food Res Technol. 2009;228(3):355–365.
- Teferra TF. Possible actions of inulin as prebiotic polysaccharide: a review. Food Frontiers. 2021;2(4):407–416.
- Vandeputte D, Falony G, Vieira-Silva S, et al. Prebiotic inulin-type fructans induce specific changes in the human gut microbiota. Gut. 2017;66(11):1968–1974.
- Cárdenas-Reyna T, Angulo C, Guluarte C, et al. In vitro immunostimulatory potential of fungal β-glucans in pacific red snapper (lutjanus Peru) cells. Dev Comp Immunol. 2017;77:350–358.
- Choromanska A, Kulbacka J, Harasym J, et al. High- and low-molecular weight oat beta-glucan reveals antitumor activity in human epithelial lung cancer. Pathol Oncol Res. 2018;24(3):583–592.
- Neri D, Balcão V, Costa R, et al. Galacto-oligosaccharides production during lactose hydrolysis by free Aspergillus oryzae β-galactosidase and immobilized on magnetic polysiloxane-polyvinyl alcohol. Food Chem. 2009;115(1):92–99.
- Vera C, Guerrero C, Illanes A, et al. Fed-batch synthesis of galacto-oligosaccharides with Aspergillus oryzae β-galactosidase using optimal control strategy. Biotechnol Progress. 2014;30(1):59–67.
- Vera C, Córdova A, Aburto C, et al. Synthesis and purification of galacto-oligosaccharides: state of the art. World J Microbiol Biotechnol. 2016;32(12):197.
- Fischer C, Kleinschmidt T. Synthesis of galactooligosaccharides in milk and whey: a review. Compr Rev Food Sci Food Saf. 2018;17(3):678–697.
- Davani-Davari D, Negahdaripour M, Karimzadeh I, et al. Prebiotics: definition, types, sources, mechanisms, and clinical applications. Foods. 2019;8(3):92.
- Fara A, Sabater C, Palacios J, et al. Prebiotic galactooligosaccharides production from lactose and lactulose by Lactobacillus delbrueckii subsp. bulgaricus CRL450. Food Funct. 2020;11(7):5875–5886.
- Tzortzis G, Goulas AK, Baillon ML, et al. In vitro evaluation of the fermentation properties of galactooligosaccharides synthesised by alpha-galactosidase from Lactobacillus reuteri. Appl Microbiol Biotechnol. 2004;64(1):106–111.
- Holt SM, Teresi JM, Côté GL. Influence of alternansucrase-derived oligosaccharides and other carbohydrates on alpha-galactosidase and alpha-glucosidase activity in Bifidobacterium adolescentis. Lett Appl Microbiol. 2008;46(1):73–79.
- Singh SP, Jadaun JS, Narnoliya LK, et al. Prebiotic oligosaccharides: special focus on fructooligosaccharides, its biosynthesis and bioactivity. Appl Biochem Biotechnol. 2017;183(2):613–635.
- Ahmed W, Rashid S. Functional and therapeutic potential of inulin: a comprehensive review. Crit Rev Food Sci Nutr. 2019;59(1):1–13.
- Tannock GW, Munro K, Bibiloni R, et al. Impact of consumption of oligosaccharide-containing biscuits on the fecal microbiota of humans. Appl Environ Microbiol. 2004;70(4):2129–2136.
- Garrigues C, Loubiere P, Lindley ND, et al. Control of the shift from homolactic acid to mixed-acid fermentation in Lactococcus lactis: predominant role of the NADH/NAD + ratio. J Bacteriol. 1997;179(17):5282–5287.
- Borch E, Berg H, Holst O. Heterolactic fermentation by a homofermentative lactobacillus sp. during glucose limitation in anaerobic continuous culture with complete cell recycle. J Appl Bacteriol. 1991;71(3):265–269.
- Ignatova T, Iliev I, Kirilov N, et al. Effect of oligosaccharides on the growth of Lactobacillus delbrueckii subsp. bulgaricus strains isolated from dairy products. J Agric Food Chem. 2009;57(20):9496–9502.
- Smith PM, Howitt MR, Panikov N, et al. The microbial metabolites, short-chain fatty acids, regulate colonic treg cell homeostasis. Science. 2013;341(6145):569–573.
- Van Laere KM, Beldman G, Voragen AG. A new arabinofuranohydrolase from Bifidobacterium adolescentis able to remove arabinosyl residues from double-substituted xylose units in arabinoxylan. Appl Microbiol Biotechnol. 1997;47(3):231–235.
- Kaplan H, Hutkins RW. Metabolism of fructooligosaccharides by Lactobacillus paracasei 1195. Appl Environ Microbiol. 2003;69(4):2217–2222.
- Molin G. Probiotics in foods not containing milk or milk constituents, with special reference to Lactobacillus plantarum 299v. Am J Clin Nutr. 2001;73(2):380S–385s.
- Abouloifa H, Khodaei N, Rokni Y, et al. The prebiotics (fructo-oligosaccharides and xylo-oligosaccharides) modulate the probiotic properties of lactiplantibacillus and levilactobacillus strains isolated from traditional fermented olive. World J Microbiol Biotechnol. 2020;36(12):185.
- Iliev I, Vasileva T, Bivolarski V, et al. Metabolic profiling of xylooligosaccharides by lactobacilli. Polymers (Basel). 2020;12(10):2387.. Crossref]
- Hartstra AV, Bouter KE, Bäckhed F, et al. Insights into the role of the microbiome in obesity and type 2 diabetes. Diabetes Care. 2015;38(1):159–165.
- Schwiertz A, Taras D, Schäfer K, et al. Microbiota and SCFA in lean and overweight healthy subjects. Obesity (Silver Spring). 2010;18(1):190–195. [). Crossref]
- Scheppach W. Effects of short chain fatty acids on gut morphology and function. Gut. 1994;35(1 Suppl):S35–S38.
- Hallert C, Björck I, Nyman M, et al. Increasing fecal butyrate in ulcerative colitis patients by diet: controlled pilot study. Inflamm Bowel Dis. 2003;9(2):116–121.
- Parada Venegas D, De la Fuente MK, Landskron G, et al. Short chain fatty acids (SCFAs)-mediated gut epithelial and immune regulation and its relevance for inflammatory bowel diseases. Front Immunol. 2019;10:277.
- Wu Y, Jha R, Li A, et al. Probiotics (Lactobacillus plantarum HNU082) supplementation relieves ulcerative colitis by affecting intestinal barrier functions, immunity-related gene expression, gut microbiota, and metabolic pathways in mice. Microbiol Spectr. 2022;10(6):e0165122.
- Hosseinkhani F, Heinken A, Thiele I, et al. The contribution of gut bacterial metabolites in the human immune signaling pathway of non-communicable diseases. Gut Microbes. 2021;13(1):1–22.
- Scheppach W, Sommer H, Kirchner T, et al. Effect of butyrate enemas on the colonic mucosa in distal ulcerative colitis. Gastroenterology. 1992;103(1):51–56.
- Davie JR. Inhibition of histone deacetylase activity by butyrate. J Nutr. 2003;133(7 Suppl):2485S–2493S.
- Gao Z, Yin J, Zhang J, et al. Butyrate improves insulin sensitivity and increases energy expenditure in mice. Diabetes. 2009;58(7):1509–1517.
- Liang H, Ward WF. PGC-1alpha: a key regulator of energy metabolism. Adv Physiol Educ. 2006;30(4):145–151.
- Donohoe DR, Garge N, Zhang X, et al. The microbiome and butyrate regulate energy metabolism and autophagy in the mammalian colon. Cell Metab. 2011;13(5):517–526.
- Tilg H, Kaser A. Gut microbiome, obesity, and metabolic dysfunction. J Clin Invest. 2011;121(6):2126–2132.