Abstract
Production of bioethanol from rice straw has attracted attention from the point of effective use of agricultural residue. Starch content is an important determinant for bioethanol production from rice straw. The overexpression of CO2-responsive CCT protein (CRCT), which is the positive regulator of starch synthesis in vegetative organs, notably increased the starch content in rice straw. To produce the bioethanol from rice straw, the dilute acid pretreatment is a general pretreatment method. Importantly, the glucose yields in liquid hydrolyzate after dilute acid pretreatment was markedly increased in the CRCT overexpression lines compared with non-transgenic rice. In addition, the overexpression of CRCT enhanced the biomass production. In contrast, CRCT did not affect on the glucose yields from cellulose in acid-insoluble residue obtained after dilute acid pretreatment. With respect to byproduct in liquid hydrolyzate which inhibits the fermentation, the formic acid content was increased, whereas the furfural, 5-hydroxymethylfurfural and acetic acid contents were unchanged by the overexpression of CRCT. These results demonstrate that genetic engineering of CRCT is an effective method to increase the bioethanol production from rice straw.
Classification:
1. Introduction
Atmospheric CO2 concentration has been increased since industrial revolution and reached 400 ppm on 2015. In order to reduce an increase in the atmospheric CO2 concentration and the CO2 emission from fossil fuel consumption, shift from the petrochemical industry to the natural resource industry using plant biomass is considered as an effective strategy. Recently, the biofuels have received a great deal of attention in terms of carbon neutral (Kim & Dale, Citation2004). However, maize, sugar cane and cassava which have been used for bioethanol production are very important food crops, and then bioethanol production have developed a business competition with food supply. Given these facts, the development of biomass which does not compete with food supply is required for future bioethanol production.
Rice is one of the most important crops for staple food and its production is third following maize and wheat in the world. The amount of production of rice straw is more than 700 million tons in the world while most of it has been treated as agricultural waste (Kim & Dale, Citation2004). However, rice straw is mostly composed of polysaccharide such as cellulose and starch which can be useful for bioethanol production (Arai-Sanoh et al., Citation2011). Therefore, rice straw has attracted attention as a raw material for bioethanol production (Binod et al., Citation2010; Hattori & Morita, Citation2010; Sarkar et al., Citation2012). To produce the bioethanol using rice straws, polysaccharide must be hydrolyzed to fermentable sugars such as glucose. Starch is relatively susceptible to hydrolysis by acids and suitable substance for ethanol fermentation. On the contrary, cellulose cannot be easily hydrolyzed by acids and is needed the catalysis by cellulase. However, cellulose forms a highly crystalline structure and robust cell walls with hemicellulose and lignin so that cellulose in cell walls is highly resistant to enzymatic hydrolysis. Thus, pretreatment is essential to break the cell wall structure of lignocellulose biomass and various pretreatment methods have been developed to improve the efficiency of cellulose hydrolysis (Alvira et al., Citation2010).
Development of useful plants is an effective way to increase bioethanol production as well as processing step of plant materials. The most promising class of mutants are the brown midrib mutants which have brown vascular tissue in the leaves and stem as a result of changes in lignin composition in cell walls (Vermerris et al., Citation2007). Brown midrib mutants of maize and sorghum have been used as feedstock because they are easy to process. Recently, brown midrib mutants were applied for bioethanol production (Vermerris et al., Citation2007). Several studies have also reported the improvement of the bioethanol production by genetic engineering of plants. For example, Fu et al. (Citation2011) demonstrated that the knockdown of caffeic acid O-methyltransferase gene related to lignin biosynthesis decreased the lignin content and increased the bioethanol production in switchgrass. In contrast, the starch contents in plants have not been a target of the improvement of the bioethanol production by breeding and genetic engineering even though it is a determinant of glucose content in liquid hydrate after dilute acid pretreatment (Teramura et al., Citation2013).
In our previous study, we showed that a novel CONSTANS, CONSTANS-like and TOC1 (CCT) domain-containing protein, named CO2-responsive CCT Protein (CRCT), is a positive regulator of starch synthesis in vegetative organ of rice (Morita et al., Citation2015). Interestingly, the overexpression of CRCT greatly increased the starch content of straw in rice. Thus, it is likely that the overexpression of CRCT can be useful for bioethanol production from rice straws. However, the productivity of bioethanol using rice straw can be determined by many factors such as utilization efficiency of cellulose in cell wall, biomass production and grain yield as well as starch content in rice straw. Therefore, it is necessary to analyze the biomass characteristics of CRCT overexpression line in detail in order to clarify whether the overexpression of CRCT is an effective way to improve the productivity of bioethanol. In this study, using CRCT overexpression lines and knockdown line, we demonstrated that the overexpression of CRCT markedly enhanced the glucose yield from rice straws by increasing the starch content and biomass production.
2. Materials and methods
2.1. Plant materials and growth conditions
Non-transgenic rice (Oryza sativa L. cv. Nipponbare), CRCT overexpression lines and CRCT RNAi knockdown line were used in this study (Morita et al., Citation2015). In CRCT overexpression lines, the full length coding region of CRCT cDNA was constitutively overexpressed using rice actin promotor. In knockdown line, RNAi constract containing 3’UTR of CRCT were constitutively expressed using maize ubiquitin promoter. Rice plants were grown in soil under natural light and flooding conditions in a temperature-controlled greenhouse (30 °C day and 23 °C night). Rice seedlings at the 4.5 leaf stage were transplanted into 1/5000 a pots supplemented with a chemical fertilizer (N:P:K = 8:8:8) at .3 g N, a delayed release nitrogen fertilizer at 1.0 g, a micronutrient fertilizer at .15 g, and a silica at 4 g. After 60 days after transplanting, the chemical fertilizer was applied as top dressing at .16 g N per pot.
At 50 days after flowering, all panicles were removed from plants. Then, the straws were harvested just above ground levels. The straws and the panicles were dried at 80 and 50 °C, respectively, for 3 days. Then, dry weight and panicles number were determined. After hand-threshing panicles, the number of spikelets were counted. All spikelets were divided into filled and unfilled grains by soaking in sodium chloride solution (specific gravity = 1.06), and then grain filling ratio was determined. Thousand-grain weights were determined by measuring the brown rice weights. The rice straws were powdered using a Shake Master Auto grinding apparatus (BioMedical Science Co., Ltd., Tokyo, Japan) and used for subsequent analysis.
2.2. Dilute acid pretreatment
Dilute acid pretreatment was performed according to Teramura et al. (Citation2013). The powder straw (6 g) was suspended in 80 ml of 1% (w/v) sulfuric acid solution, and incubated at 180 °C for 45 min with agitation at 200 rpm in a laboratory scale thermostirrer (HME-19G-U; Koike Precision Instruments, Kawasaki, Japan). After pretreatment, the mixture was separated into liquid hydrolyzate and acid-insoluble residue by filtration using filter paper (No.1; ADVANTEC, Tokyo, Japan). The acid-insoluble residue was suspended with deionized water, neutralized to pH 7.0 by the addition of calcium hydroxide powder and washed with deionized water. Then, the insoluble residue was dried and weighted using an electric balance (XS105DU; METTLER TOLEDO, Greifensee, Switzerland). The liquid hydrolyzate was neutralized and adjusted to pH 5.0 by the addition of calcium hydroxide powder. The sugar content in the liquid hydrolyzate was determined by GC-MS as described below.
2.3. Determination of sugar content
Sugar content was determined as described Matsuda et al. (Citation2011). Equal amounts (1.5 μL) of liquid hydrolyzate and .1% (w/w) ribitol were added to tubes and dried using a vacuum concentrator (7,810,010; Labconco, Kansas City, MO). The resultant residue was dissolved in 100 μL of a mixture of 20 mg mL−1 of methoxyamine hydrochloride in pyridine and incubated at 30 °C for 90 min. After incubation, 50 μL of N-methyl-N-trimethylsilyltrifluoroacetamide (MSTFA) was added to the sample solution and incubated at 37 °C for 30 min. Aliquots of sample solutions (10 μL) were subjected to gas chromatography-mass spectrometry (GC-MS-2010 Plus; Shimadzu, Kyoto, Japan) under the following conditions: column, Agilent CP-Sil 8CB-MS (30 m × .25 mm); carrir gas, helium; injection temperature, 230 °C; oven temperature, 80 °C at t = 0 to 2 min, then to 330 °C at 15 °C min−1.
2.4. Compositional analysis of raw biomass and acid-insoluble residue
The compositions of biomass samples were determined according to the previous report based on National Renewable Energy Laboratory analytical method (Sluiter et al., Citation2011; Teramura et al., Citation2013). In the first step, 3 ml of 72% (w/v) sulfuric acid was added to 300 mg of dried raw biomass or acid-insoluble residue, and incubated at 30 °C for 2 h. In the second step, the mixtures were diluted to 4% (w/w) sulfuric acid with deionized water and autoclaved at 121 °C for 1 h. The contents in hydrolysis solution were determined by GC-MS under the conditions described above.
To release starch from raw biomass, 750 μL of .5 M NaOH was added to 75 mg of dried raw biomass and incubated at room temperature for 20 min. After removal of the residue, the sample solution was neutralized and adjusted to pH 5 by the addition of .5 M acetic acid, and diluted ten-times with deionized water. Hundred microliters of enzyme mixture containing 8 units α-amylase (Wako Pure Chemical Industries Ltd. Tokyo, Japan) and 13 units glucoamylase (Wako Pure Chemical Industries Ltd.) in .5 M sodium acetate was added to 100 μL sample solution and incubated at 50 °C for 1 h. Liberated sugars were determined by GC-MS under the condition described above.
2.5. Enzymatic saccharification
Enzymatic saccharification of acidinsoluble residue was conducted according to the method described by Teramura et al. (Citation2016). Ten percent (w/v) acid-insoluble residue was digested by 6.6 filter paper units (FPU) g−1 dry weight cellulase (Celic CTec2, Novozyme, Bagsvaerd, Denmark) in .3 M citrate buffer (pH 4.8). Tetracycline (40 μg mL−1) and cycloheximide (30 μg mL−1) were added to the cellulase reaction mixture to prevent microbial growth. The reaction mixture was incubated at 50 °C in an organic synthesizing apparatus (PPS-2000, Tokyo Rikakikai, Tokyo, Japan) with agitation at 120 rpm for 72 h. Cellulase reaction was stopped by rapid chilling on ice, followed by centrifugation at 20,000 × g for 10 min at 4 °C. The sugars in the supernatant were analyzed by GC-MS as described above.
2.6. Determination of byproduct contents
The contents of byproduct of dilute acid pretreatment were measured as described Matsuda et al. (Citation2011). Acetone (900 μL) was added to 100 μL of liquid hydrolyzate and mixed thoroughly. The sample mixture was then centrifuged at 20,000 × g for 10 min at room temperature. The supernatant (10 μL) was subjected to GC-MS analysis (GC-MS-2010plus; Shimadzu). 5-Hydroxymethylfurfural (5-HMF) and furfural were analyzed under the following condition: column, Agilent CP-Sil 8CB-MS (30 m × .25 mm); carrier gas, helium; injection temperature, 250 °C; oven temperature, 50 °C at t = 0–5 min, then increased to 280 °C at 20 °C min−1. Acetic acid and formic acid were analyzed under the following condition: column, Agilent DB-FFAP (60 m × .25 mm); carrier gas, helium; injection temperature, 250 °C; oven temperature, 100 °C at t = 0 to 5 min, then increased to 230 °C at 10 °C min−1.
3. Results and discussion
3.1. Effects of CRCT expression levels on glucose content in liquid hydrolyzate of rice straw
The straws of CRCT transgenic lines were pretreated with dilute acid, and then the glucose and xylose contents in liquid hydrolyzate and the weight of acid-insoluble residue were determined (Figure ). The glucose content of non-transgenic rice was approximately 7.5 g L−1. Notably, the glucose contents of CRCT overexpression lines were 17.8–21.8 g L−1, and hence were 2–3 times higher than that of non-transgenic rice. In contrast, the glucose content of CRCT knockdown line was significantly lower than that of non-transgenic line, which was 4.4 g L−1. The xylose contents of CRCT overexpression lines were significantly decreased, while that of CRCT knockdown line was comparable with that of non-transgenic rice. The weight of acid-insoluble residue of CRCT transgenic lines exhibited a similar tendency in the case of xylose content, i.e. the weight of acid-insoluble residue of CRCT overexpression lines were significantly decreased compared with that of non-transgenic rice. According to Teramura et al. (Citation2013), the glucose contents in liquid hydrolyzate were determined by the constituents of the rice straw, especially starch. The xylose contents were mostly determined by the contents of hemicelluloses. Therefore, the composition of rice straws of CRCT transgenic lines was analyzed (Table ). As expected from the results of Morita et al. (Citation2015), the starch contents of CRCT overexpression lines were greatly increased compared with that of non-transgenic rice. In addition, the cellulose contents of CRCT overexpression lines tended to be higher than that of non-transgenic rice. On the contrary, the hemicellulose contents and acid-insoluble lignin contents of CRCT overexpression lines were significantly decreased compared with those of non-transgenic line. Meanwhile, the changes in these compositions in knockdown line showed the opposite trend to those in CRCT overexpression lines.
Figure 1. Soluble sugar contents and pretreatment residue in hydrolyzate of rice straw.
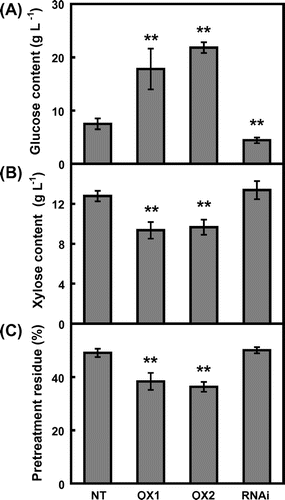
Table 1. Composition of constituents in rice straw of CRCT transgenic lines.
There have been some studies on the bioethanol production from rice straw by dilute acid pretreatment. Glucose content in liquid hydrolyzate of rice straw cultivated in Taiwan was reported to be 8.5 g per 100 g dry weight (DW) (Hsu et al., Citation2010). However, Teramura et al. (Citation2013) reported that glucose contents in liquid hydrolyzate of rice straw was varied depending on cultivars and ranged from 5.0 to 24.5 g per 100 g DW among 13 rice cultivars in Japan. Recently, Goda et al. (Citation2016) reported more thorough research of glucose contents in liquid hydrolyzate from straws using 208 rice cultivars that there were significant variations in glucose contents among rice cultivar and the glucose content of rice cultivar ‘Tachisuzuka’ was the highest among 208 rice cultivars showing 23 g per 100 g DW. Calculating from the result of Figure , CRCT overexpression lines showed markedly higher glucose contents in liquid hydrolyzate of straw that the glucose contents in liquid hydrolyzate of CRCT overexpression lines were amounted to 23.7–29.1 g per 100 g DW. Thus, the glucose yields of CRCT overexpression lines were higher than most of existing rice cultivars. Therefore, it is apparent that higher glucose yield observed in overexpression lines should be due to the high starch contents of rice straw.
3.2. Fermentation inhibitor contents in liquid hydrolyzate of rice straw
The dilute acid pretreatment of biomass generates some fermentation inhibitors as byproducts (Teramoto et al., Citation2008). Therefore, typical byproducts such as furfural, 5-HMF, acetic acid and formic acid were analyzed in liquid hydrolyzate after dilute pretreatment of rice straw (Figure ). The furfural contents and 5-HMF contents in CRCT transgenic lines were not significantly different from those of non-transgenic rice. However, the acetic acid content of CRCT knockdown line was lower than that of non-transgenic rice. The formic acid contents of CRCT overexpression lines were higher than that of non-transgenic rice. These results indicated that the expression levels of CRCT affected the byproduct contents such as acetic acid and formatic acid in liquid hydrolyzate.
Figure 2. Fermentation inhibitor contents in hydrolyzate of rice straw.
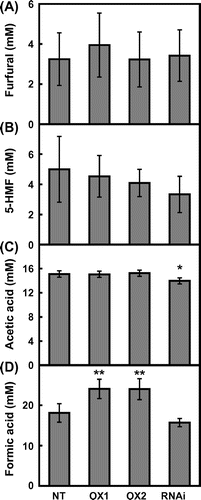
Teramura et al. (Citation2015) suggested that 5-HMF and formic acid in liquid hydrolyzate were likely derived from the hydrolytic reaction of starch to glucose during dilute acid pretreatment. In this study, the formic acid contents of CRCT overexpression lines which showed high starch content in stem were significantly increased compared with that of non-transgenic rice. Thus, it is considered that high glucose production in CRCT overexpression lines simultaneously increased the formic acid contents in liquid hydrolyzate. The generation of fermentation inhibitors is considered to be inevitable in dilute acid pretreatment. Thus, it is proposed that the development of yeast strain resistant to fermentation inhibitors (Hasunuma et al., Citation2011; Zaldivar et al., Citation2001), the establishment of effective method for the separation of fermentation inhibitors and the improvement of pretreatment methods to reduce the generation of fermentation inhibitors would be necessary to solve this problem.
3.3. Saccharification efficiency of acid-insoluble residue
To clarify the effects of CRCT expression levels on the glucose yield from acid-insoluble residue, the saccharification efficiency of cellulose by cellulase in acid-insoluble residue of CRCT transgenic lines were analyzed (Figure ). The saccharification efficiency was 49.4% in non-transgenic rice. The CRCT overexpression lines showed similar saccharification efficiency with non-transgenic rice which was approximately 50%. The saccharification efficiency of CRCT knockdown line was 42.7% which was significantly lower than that of non-transgenic rice. The carbohydrates and lignin composition of acid-insoluble residue of CRCT transgenic lines were almost unchanged compared with non-transgenic rice (Table ). Although the overexpression of CRCT markedly increased the glucose yield from liquid hydrolyzate, it did not largely affect on the enzymatic saccharification of cellulose in acid-insoluble residue.
Figure 3. Saccharification efficiency of acid-insoluble residue.
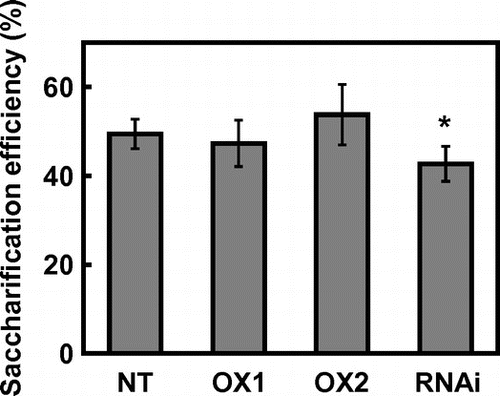
Teramura et al. (Citation2015) indicated that the compositional heterogeneity of rice straws among rice cultivars was substantially reduced by dilute acid pretreatment, which led to similar glucose yield from acid-insoluble residue. In this study, the compositional heterogeneities of acid-insoluble residue of CRCT transgenic lines were substantially lower than those of rice straws (Tables and ). Therefore, the glucose yields from acid-insoluble residue of CRCT transgenic lines were considered to be almost similar levels compared with that of non-transgenic rice.
Table 2. Composition of acid-insoluble residue after dilute acid pretreatment.
3.4. Effects of CRCT expression levels on the biomass production
Biomass production is one of the most important traits for raw material crop for bioethanol production. Therefore, the effects of CRCT expression levels on the biomass production and grain yield were examined (Table ). Notably, the dry weights of straws were increased in CRCT overexpression lines. In contrast, the dry weight of CRCT knockdown line was comparable with that of non-transgenic rice. However, the grain filling rate and 1000-grain weight of CRCT overexpression lines were significantly decreased compared with those of non-transgenic rice. These observations probably led to the reduction of grain yield in CRCT overexpression lines. Previous studies demonstrated that ‘Tachisuzuka’ and ‘Leafstar’ could accumulate high levels of carbohydrates in stems (Matsushita et al., Citation2011; Ookawa et al., Citation2010). The glucose contents in liquid hydrolyzate of rice straw after dilute acid pretreatment using these cultivars were also very high (Goda et al., Citation2016; Teramura et al., Citation2013). However, as observed in CRCT overexpression lines, the grain yield of these rice cultivars was significantly low because of their small panicles (Matsushita et al., Citation2011). Thus, it is considered that there should be trade-off relationship between the glucose content of rice straw and the grain yield.
Table 3. Yield and yield components of CRCT transgenic lines.
4. Conclusion
In this study, we showed that the overexpression of CRCT strikingly increased the glucose content of rice straw and biomass. These findings must be valuable for the efficient production of bioethanol from rice straw. However, as a disadvantage, the CRCT overexpression lines showed low grain yield. This defect in yield should be improved and can be overcome by the modification of genetic engineering. In our transgenic line, CRCT was overexpressed using the actin promoter which provides high levels of constitutive expression (Morita et al., Citation2015). The constitutive enhancement of starch synthesis could reduce the partitioning of photoassimilates to the reproductive organ when needed for its development. However, this can be avoided by using some promoters which direct specific expression at late ripening stage to overexpress CRCT. Another possibility is the improvement of source capacity, i.e. the enhancement of photosynthesis because the reduction in grain yield was caused by a deficiency of photoassimilates. Elevated CO2 is known to enhance the photosynthetic rate and grain yield in most C3 plants including rice (Ainsworth & Long, Citation2005). Thus, decreased yield observed in CRCT overexpression lines can be ameliorated by the elevated CO2 treatment. Interestingly, the CRCT overexpression lines showed higher photosynthetic rate than non-transgenic rice when they were grown under elevated CO2 condition (Morita et al., Citation2016). Thus, it is possible that our transgenic lines move toward goal of the development of rice showing high starch content in straw and high yield in the future high CO2 environment.
Disclosure statement
No potential conflict of interest was reported by the authors.
Funding
This work was supported by JSPS Research [grant number 16J03481] for R.M.; JSPS KAKENHI [grant number 22114511] for H.F.; the Network of Centers of Carbon Dioxide Resource Studies in Plants (NC-CARP) for A.K.
References
- Ainsworth, E. A., & Long, S. P. (2005). What have we learned from 15 years of free air CO2 enrichment (FACE)? A meta-analytic review of the responses of photosynthesis, canopy properties and plant production to rising CO2. New Phytologist, 165, 351–371.
- Alvira, P., Tomás-Pejó, E., Ballesteros, M., & Negro, M. J. (2010). Pretreatment technologies for an efficient bioethanol production process based on enzymatic hydrolysis: A review. Bioresource Technology, 101, 4851–4861.10.1016/j.biortech.2009.11.093
- Arai-Sanoh, Y., Ida, M., Zhao, R., Yoshinaga, S., Takai, T., Ishimaru, T., … Kondo, M. (2011). Genotypic variations in non-structural carbohydrate and cell-wall components of the stem in rice, sorghum, & sugar cane. Bioscience, Biotechnology, and Biochemistry, 75, 1104–1112.10.1271/bbb.110009
- Binod, P., Sindhu, R., Singhania, R. R., Vikram, S., Devi, L., Nagalakshmi, S., … Pandey, A. (2010). Bioethanol production from rice straw: An overview. Bioresource Technology, 101, 4767–4774.10.1016/j.biortech.2009.10.079
- Fu, C., Mielenz, J. R., Xiao, X., Ge, Y., Hamilton, C. Y., Rodriguez, M., Jr, … Wang, Z.Y. (2011). Genetic manipulation of lignin reduces recalcitrance and improves ethanol production from switchgrass. Proceedings of the National Academy of Sciences, 108, 3803–3808.10.1073/pnas.1100310108
- Goda, T., Teramura, H., Suehiro, M., Kanamaru, K., Kawaguchi, H., Ogino, C., … Yamasaki, M. (2016). Natural variation in the glucose content of dilute sulfuric acid-pretreated rice straw liquid hydrolysates: Implications for bioethanol production. Bioscience, Biotechnology, and Biochemistry, 80, 863–869.10.1080/09168451.2015.1136882
- Hasunuma, T., Sanda, T., Yamada, R., Yoshimura, K., Ishii, J., & Kondo, A. (2011). Metabolic pathway engineering based on metabolomics confers acetic and formic acid tolerance to a recombinant xylose-fermenting strain of Saccharomyces cerevisiae. Microbial Cell Factories, 10, 2.10.1186/1475-2859-10-2
- Hattori, T., & Morita, S. (2010). Energy crops for sustainable bioethanol production; which, where and how? Plant Production Science, 13, 221–234.10.1626/pps.13.221
- Hsu, T. C., Guo, G. L., Chen, W. H., & Hwang, W. S. (2010). Effect of dilute acid pretreatment of rice straw on structural properties and enzymatic hydrolysis. Bioresource Technology, 101, 4907–4913.10.1016/j.biortech.2009.10.009
- Kim, S., & Dale, B. E. (2004). Global potential bioethanol production from wasted crops and crop residues. Biomass & Bioenergy, 26, 361–375.10.1016/j.biombioe.2003.08.002
- Matsuda, F., Yamasaki, M., Hasunuma, T., Ogino, C., & Kondo, A. (2011). Variation in biomass properties among rice diverse cultivars. Bioscience, Biotechnology, and Biochemistry, 75, 1603–1605.10.1271/bbb.110082
- Matsushita, K., Iida, S., Ideta, O., Sunohara, Y., Maeda, H., Tamura, Y., … Takakuwa, M. (2011). ‘Tachisuzuka’, a new rice cultivar with high straw yield and high sugar content for whole-crop silage use. Breeding Science, 61, 86–92.10.1270/jsbbs.61.86
- Morita, R., Sugino, M., Hatanaka, T., Misoo, S., & Fukayama, H. (2015). CO2-responsive CONSTANS, CONSTANS-like, and time of chlorophyll a/b binding protein Expression1 protein is a positive regulator of starch synthesis in vegetative organs of rice. Plant Physiology, 167, 1321–1331.10.1104/pp.15.00021
- Morita, R., Inoue, K., Ikeda, K., Hatanaka, T., Misoo, S., & Fukayama, H. (2016). Starch content in leaf sheath controlled by CO2-responsive CCT protein is a potential determinant of photosynthetic capacity in rice. Plant and Cell Physiology, 57, 2334–2341.10.1093/pcp/pcw142
- Ookawa, T., Yasuda, K., Kato, H., Sakai, M., Seto, M., Sunaga, K., … Hirasawa, T. (2010). Biomass production and lodging resistance in ‘Leaf Star’, a new long-culm rice forage cultivar. Plant Production Science, 13, 58–66.10.1626/pps.13.58
- Sarkar, N., Ghosh, S. K., Bannerjee, S., & Aikat, K. (2012). Bioethanol production from agricultural wastes: An overview. Renewable Energy, 37, 19–27.10.1016/j.renene.2011.06.045
- Sluiter, A., Hames, B., R. Ruiz, Scarlata, C., Sluiter, J. & Templeton, D. (2011). Determination of structural carbohydrates and lignin in biomass. Golden: National Renewable Energy Laboratory, Midwest Research Institute, Contract No. DE-AC36-99-GO10337.
- Teramoto, Y., Lee, S. H., & Endo, T. (2008). Pretreatment of woody and herbaceous biomass for enzymatic saccharification using sulfuric acid-free ethanol cooking. Bioresource Technology, 99, 8856–8863.10.1016/j.biortech.2008.04.049
- Teramura, H., Oshima, T., Matsuda, F., Sasaki, K., Ogino, C., Yamasaki, M., & Kondo, A. (2013). Glucose content in the liquid hydrolysate after dilute acid pretreatment is affected by the starch content in rice straw. Bioresource Technology, 149, 520–524.10.1016/j.biortech.2013.09.109
- Teramura, H., Sasaki, K., Oshima, T., Aikawa, S., Matsuda, F., Okamoto, M., … Kondo, A. (2015). Changes in lignin and polysaccharide components in 13 cultivars of rice straw following dilute acid pretreatment as studied by solution-state 2D 1H-13C NMR. PLoS ONE, 10, e0128417.10.1371/journal.pone.0128417
- Teramura, H., Sasaki, K., Oshima, T., Matsuda, F., Okamoto, M., Shirai, T., … Kondo, A. (2016). Organosolv pretreatment of sorghum bagasse using a low concentration of hydrophobic solvents such as 1-butanol or 1-pentanol. Biotechnology for Biofuels, 9, 1403.10.1186/s13068-016-0427-z
- Vermerris, W., Saballos, A., Ejeta, G., Mosier, N. S., Ladisch, M. R., & Carpita, N. C. (2007). Molecular breeding to enhance ethanol production from corn and sorghum stover. Crop Science, 47, S142–S153.
- Zaldivar, J., Nielsen, J., & Olsson, L. (2001). Fuel ethanol production from lignocellulose: A challenge for metabolic engineering and process integration. Applied Microbiology and Biotechnology, 56, 17–34.10.1007/s002530100624