Abstract
C4 plants show higher photosynthetic capacity and resource use efficiency than C3 plants. However, the genetic variations of these traits and their regulatory factors in C4 plants still remain to be resolved. We investigated physiological, biochemical, and structural traits involved in photosynthesis and photosynthetic water and nitrogen use efficiencies (PWUE and PNUE) in 22 maize lines and four teosinte lines from various regions of the world. Net photosynthetic rate (PN) ranged from 32.1 to 46.5 μmol m−2 s−1. PN was positively correlated with stomatal conductance, transpiration rate, and chlorophyll, nitrogen and soluble protein contents of leaves, but not with specific leaf weight. PN was positively correlated with the activities of ribulose-1,5-bisphosphate carboxylase/oxygenase and the C4-acid decarboxylases, NADP-malic enzyme and phosphoenolpyruvate carboxykinase, but not with the activity of phosphoenolpyruvate carboxylase. Leaf structural traits (stomatal parameters, leaf thickness, and interveinal distance) were not correlated with PN. These data suggest that physiological and biochemical traits are involved in the genetic variation of PN, but structural traits are not directly involved. PWUE is in the lower class of values reported for C4 plants, whereas PNUE is in the highest class of values reported for C4 plants. PNUE was negatively correlated with leaf nitrogen content but not significantly correlated with PN. PWUE was not correlated with δ13C values of leaves, indicating difficulty in using δ13C values as an indicator of PWUE of maize. In general, teosinte lines showed lower PN but higher PWUE than maize lines.
Classification:
Introduction
Photosynthetic traits of leaves are one of the most important physiological factors responsible for plant productivity. The improvement of photosynthetic traits promises further increases in plant productivity (Evans, Citation2013; Zhu et al., Citation2010). The genetic variation in photosynthetic traits found in both crop and wild species includes a potential to improve crop photosynthesis and ultimately productivity but is largely unexplored (McCouch, Citation2004; Flood et al., Citation2011). Photosynthesis is complicatedly regulated by biophysical, biochemical, physiological, and structural traits of leaves. However, our understanding of the regulatory processes is still insufficient (Evans, Citation2013). The factors that cause the genetic variation in photosynthesis also remain largely unknown (Flood et al., Citation2011).
It is well known that C4 plants have higher photosynthetic rate and productivity than C3 plants (Brown, Citation1999; Osmond et al., Citation1982). This is attained by a CO2 concentrating mechanism (CCM) operating in C4 plants. In C4 leaves, mesophyll and bundle-sheath (BS) cells are differentiated and surround vascular bundles. In C4 photosynthesis, atmospheric CO2 entering through stomata is fixed by phosphoenolpyruvate carboxylase (PEPC) in mesophyll cells. The C4 acids produced are transported to BS cells, where they are decarboxylated by C4 acid decarboxylase. Released CO2 is fixed by Rubisco. This biochemical process raises the CO2 concentration around Rubisco in BS cells and thus reduces photorespiration (Hatch, Citation1987). The quantitative balance between mesophyll and BS cells is also required to attain the intimate cooperation between C4 and C3 cycles (Dengler et al., Citation1994). In general, C4 leaves have a denser vascular system than C3 leaves. It is thought that this structural trait is associated with rapid translocation of photosynthates in C4 plants (Leonardos & Grodzinski, Citation2000; Ueno et al., Citation2006). Because of the complex mechanism of C4 photosynthesis, the factors determining photosynthetic rate in C4 plants still remain to be resolved (von Caemmerer & Furbank, Citation2016). It is suggested that CO2 delivery process in mesophyll cells, activities and properties of C4 and C3 photosynthetic enzymes, substrate regeneration in C4 and C3 cycles, transport and diffusion of metabolites, electron transport and light capture in chloroplasts, and CO2 leakiness from BS cells are involved in the regulation of C4 photosynthesis (reviewed by von Caemmerer & Furbank, Citation2016). It also appears that biochemical, physiological, and structural traits of leaves are intricately involved in the genetic variation in photosynthetic rate of C4 plants (Tsutsumi et al., Citation2017).
Depending on the C4 acid decarboxylation system in BS cells, the C4 photosynthetic pathway is classified into three types: NADP-malic enzyme (NADP-ME), NAD-malic enzyme (NAD-ME), and phosphoenolpyruvate carboxykinase (PCK) (Hatch, Citation1987). The NADP-ME-type C4 grasses include crops with high biomass productivity, such as maize, sorghum, and Napier grass (Brown, Citation1999; Carpita & McCann, Citation2008). In general, each C4 species is thought to use only one of the 3 decarboxylation systems (Hatch, Citation1987). However, some C4 species may use more than one. Maize (Zea mays ssp. mays), a model NADP-ME-type C4 grass, uses both NADP-ME and PCK (Walker et al., Citation1997; Wingler et al., Citation1999). However, the physiological significance of the dual C4 acid decarboxylation system in C4 plants is unclear (Furbank, Citation2011; Koteyeva et al., Citation2015).
Because C4 plants have a CCM, their photosynthetic water and nitrogen use efficiencies (PWUE and PNUE) are higher than those of C3 plants (Brown, Citation1977; Osmond et al., Citation1982). These traits of C4 plants provide advantages for survival in natural habitats and are also useful for sustainable agriculture. PNUE values of NADP-ME-type C4 grasses are higher than those of NAD-ME-type C4 grasses, whereas PWUE values are not significantly different (Ghannoum et al., Citation2001, 2005, 2011). Further extensive studies would be required to clarify the genetic variation in resource use efficiency among C4 plants.
Maize is important as a grain, forage, and bioenergy crop (Carpita & McCann, Citation2008). Previous studies have addressed the genetic variation in the photosynthetic rate of maize. Some studies reported a large genetic difference in photosynthetic rate (Crosbie et al., Citation1977; Heichel & Musgrave, Citation1969), whereas others found a small difference (Baer & Schrader, Citation1985; Duncan & Hesketh, Citation1968). Previous studies suggested that Rubisco and pyruvate, Pi dikinase (PPDK) are rate-limiting enzymes in C4 photosynthesis in maize (Baer & Schrader, Citation1985; Usuda, Citation1984; Usuda et al., Citation1985; von Caemmerer & Furbank, Citation2016). However, it is still uncertain whether other physiological, biochemical, and structural factors are involved in the variation in photosynthetic rate of maize.
The aim of this study is to investigate the genetic variations in photosynthetic rate and resource use efficiency in maize lines from various regions of the world. Another aim is to determine which factors in physiological, biochemical, and structural traits of leaves regulate these genetic variations in maize lines. We examined here various traits of leaves such as gas exchange traits, chlorophyll (Chl) and nitrogen (N) contents, carbon isotope ratio, activities of C3 and C4 enzymes, stomatal parameters, and interveinal distance (IVD) for these maize lines. On the other hand, because wild lines are valuable as genetic resources to improve physiological traits of crops (Flood et al., Citation2011), four lines of teosintes (Z. mays ssp. mexicana, Zea diploperennis, Zea perennis, Zea nicaraguensis) were added for comparison.
Materials and methods
Plant materials and growth conditions
Twenty-two maize lines (Z. mays ssp. mays) and four teosinte lines (one line per species: Z. mays ssp. mexicana, Z. diploperennis, Z. perennis, and Z. nicaraguensis) were used in this study (Table ). These lines were selected from a wide range of countries. Seeds were provided by the Plant Introduction Station, Agricultural Research Service, USDA, and the NARO Genebank, Tsukuba, Japan. They were germinated in nursery boxes filled with loam granules and were grown for 10 days in a greenhouse in an experimental field of Kyushu University (33°35′N, 130°23′E) during summer 2014. Five seedlings per line were transplanted into 5-L pots (one plant per pot) filled with a sandy loam mixed with chemical fertilizer containing 1.0 g each of nitrogen, phosphorus, and potassium. Plants were then grown outdoors at a mean air temperature of 26 °C and midday PPFD of full sunlight exceeding 2000 μmol m−2 s−1. Plants were watered daily to avoid drying of soil. At 2 weeks after transplanting, the chemical fertilizer containing .6 g each of elements was supplied. At 3–4 weeks after transplanting, physiological, biochemical, and structural traits of photosynthesis were examined in fully expanded upper leaves of 3–5 plants per line. At this time, plant height was 80–120 cm in maize lines and 50–80 cm in teosinte lines, and all plants except for CM109 and CB44 were in vegetative stage. However, these two maize lines initiated to develop tassels (Supplemental data 1).
Table 1. Maize and teosinte lines examined in this study.
Gas exchange and PWUE
Gas exchange in leaves was measured using a portable photosynthesis system (Li-6400XT; Li-COR, Lincoln, NE, USA). Gas exchange parameters – net photosynthetic rate (PN), stomatal conductance (gs), transpiration rate (Tr), and intercellular CO2 concentration (Ci) – were measured at a PPFD of 2000 μmol m−2 s−1, leaf temperature of 30.0 ± .5 °C, relative humidity of 60% ± .5%, and ambient CO2 concentration (Ca) of 380 μmol mol−1. PWUE was calculated by dividing PN by Tr.
Chl and N contents, specific leaf weight, and PNUE
Chl content and specific leaf weight (SLW) were measured in the same leaves used for gas exchange measurements. Chl was extracted from the leaves (3.4 cm2) in 80% acetone, and Chl content was measured spectrophotometrically according to Arnon (Citation1949). Leaves (5.7 cm2) were air-dried for 2 days at 80 °C and weighed, and SLW was calculated by dividing dry mass by leaf area. Leaf N content was determined in lower leaves next to the ones used for gas exchange measurements. Leaves were air-dried as described above and ground to powder. The N content in .3 g of leaf powder was determined using a micro-Kjeldahl procedure (Tsutsumi et al., Citation2017). PNUE was calculated by dividing PN by N content.
Enzyme assays and leaf soluble protein content
Parts of the same leaves used for gas exchange measurements were sampled between 10:00 and 12:00 on a clear day, immediately frozen in liquid nitrogen, and stored at –80 °C. For enzyme assay, leaves (.2 g fresh mass) were ground on ice with a pestle in a mortar containing 1 mL of grinding medium [50 mM HEPES-KOH (pH 7.5), 1 mM EDTA-2Na, 5 mM dithiothreitol, 10 mM MgCl2, and .02% (v/v) Triton X-100] containing .5% (w/v) bovine serum albumin, 5 mg of polyvinylpolypyrrolidone, and .1 g of quartz sand. The homogenates were filtered through two layers of gauze, the filtrates were centrifuged for 5 min at 10,000 × g at 4 °C, and the supernatants were used for the enzyme assay. An aliquot of the filtrate was taken for determination of Chl content.
Activities of photosynthetic enzymes were assayed spectrophotometrically in 1-mL reaction mixtures at 30 °C. Activities of PEPC and NADP-ME were assayed as described by Ueno and Sentoku (Citation2006). The activity of PCK was assayed in the carboxylase direction following NADH oxidation according to Sharwood et al. (Citation2014). The total activity of Rubisco was measured as described by Ueno and Sentoku (Citation2006) except that 5 U phosphoglycerate kinase, 5 U glyceraldehyde 3-phosphate dehydrogenase, and 5 U phosphocreatine kinase were used. In the assay of Rubisco activity, the supernatant was preincubated in the presence of 10 mM NaHCO3 and 10 mM MgCl2 at 25 °C for 10 min.
For measurements of leaf soluble protein (LSP) content, leaves (.1 g fresh mass) were ground on ice, and supernatants were obtained as for enzyme assays except that bovine serum albumin was omitted and 1 mM phenylmethylsulfonyl fluoride and .002% (w/v) leupeptin were added to the grinding medium. The LSP content was measured according to Bradford (Citation1976).
Carbon isotope ratio
A part of each leaf used for gas exchange measurement was air-dried at 80 °C and separately ground in a mortar with a pestle. The same amounts of powder from each leaf were thoroughly mixed, and 2 mg of the mixture was used for measurement of 12C and 13C contents as described by Sato and Suzuki (Citation2010). The isotope ratio was expressed in δ notation in parts per million (‰) with respect to the Pee Dee belemnite standard.
Structural traits
The middle portions of leaves used for gas exchange measurements were fixed in formalin–acetic acid–alcohol solution for 1 day and cleared according to Ueno et al. (Citation2006). Stomatal density (SD), guard cell length (GL), and IVD were measured under a light microscope. Stomata were counted on each leaf surface in four .38-mm2 fields per leaf at 300× magnification. SD was calculated as the sum of the number of stomata on both sides per unit leaf area. GL of 20 cells on each side (40 cells in total) was measured with a micrometer at 600 × magnification. IVD was the mean of 10 measurements of the distance between centers of adjacent small longitudinal veins.
The middle portions of leaves were also fixed in 3% (v/v) glutaraldehyde in 50 mM sodium phosphate buffer (pH 6.8) at room temperature for 1.5 h. After washing with phosphate buffer, they were post-fixed in 2% (w/v) OsO4 in sodium phosphate buffer (pH 6.8) for 2 h, dehydrated through an acetone series, and embedded in Quetol resin (Nisshin-EM Co. Ltd., Shinjuku, Tokyo, Japan) at 70 °C. The samples were transversely sectioned at 1 μm thickness with a glass knife on an ultramicrotome and stained with 1% toluidine blue O. Leaf thickness was measured in ImageJ software (Schneider et al., Citation2012) as the mean of 10 points per section.
Statistical analysis
Data were analyzed using BellCurve for Excel (Social Survey Research Information Co., Ltd., Shinjuku, Tokyo, Japan). One-way analysis of variance (ANOVA) was used for all parameters. Pearson’s correlation coefficients between the parameters were calculated.
Results
Gas exchange and PWUE
Gas exchange traits differed significantly among the maize and teosinte lines examined (p < .01; Supplemental data 2). PN ranged from 32.1 μmol m−2 s−1 (teosinte line Ames 21875) to 46.5 μmol m−2 s−1 (maize line PI 195114) with a mean of 38.9 μmol m−2 s−1 (Figure (A); Supplemental data 2). The three teosinte lines of species other than Z. mays (PI 441930, Ames 21875, PI 615697) had lower PN than most Z. mays lines had. However, teosinte line Ames 8083 (Z. mays ssp. mexicana) had an intermediate PN value (Figure (A)). PN was positively correlated with gs (Figure (A)) and Tr (Figure (B)) but not with Ci/Ca (Table ). PWUE ranged from 5.25 mmol mol−1 (maize line IL14H) to 7.02 mmol mol−1 (Ames 21875), with a mean of 6.07 mmol mol−1 (Figure (B); Supplemental data 2). PWUE was high in all teosinte lines except PI 615697 (Figure (B); Supplemental data 2). PWUE was negatively correlated with PN, gs, Tr, and Ci/Ca (Table ).
Figure 1. Variations in (A) net photosynthetic rate (PN), (B) photosynthetic water use efficiency (PWUE), and (C) photosynthetic nitrogen use efficiency (PNUE) in leaves of maize and teosinte lines.
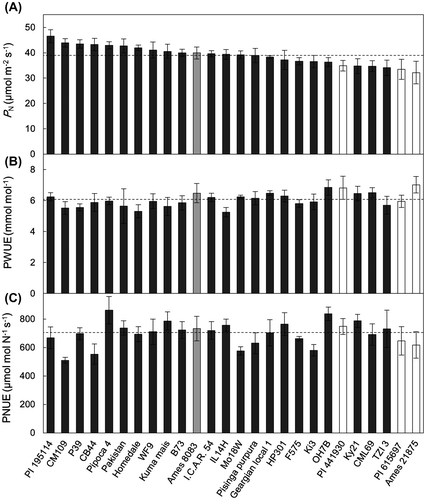
Figure 2. Relationships between PN and (A) stomatal conductance (gs), (B) transpiration rate (Tr), (C) chlorophyll (Chl) content, (D) leaf soluble protein (LSP) content, (E) leaf N content, and (F) specific leaf weight (SLW) in leaves of maize and teosinte lines.
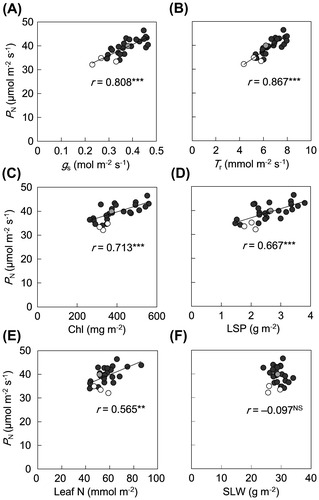
Table 2. Correlation coefficients (r) from linear regression analysis and statistical significance of the relationships between physiological and biochemical traits in maize and teosinte lines. PN, net photosynthetic rate; gs, stomatal conductance; Tr, transpiration rate; Ci/Ca, intercellular CO2 to ambient CO2 concentration; PWUE, photosynthetic water use efficiency; LSP, leaf soluble protein; Chl, chlorophyll; SLW, specific leaf weight; PNUE, photosynthetic nitrogen use efficiency; δ13C, carbon isotope ratio; PEPC, phosphoenolpyruvate carboxylase; ME, malic enzyme; PCK, phosphoenolpyruvate carboxykinase; DC, capacity for C4 acid decarboxylation (=NADP−ME activity + PCK activity); PCK ratio, [=PCK activity/(NADP−ME activity + PCK activity)].
Other physiological traits
All these traits except δ13C values showed significant differences among lines (p < .001; Supplemental data 3). Chl and LSP contents showed large variations among lines, with maximum-to-minimum ratios of 2.1 and 2.6, respectively (Supplemental data 3). The contents of Chl (Figure (C)), LSP (Figure (D)), and N (Figure (E)) were positively correlated with PN, whereas that of SLW (Figure (F)) was not. PNUE ranged from 510 μmol mol N−1 s−1 (CM109) to 862 μmol mol N−1 s−1 (Pipoca 4), with a mean of 700 μmol mol N−1 s−1 (Figure (C); Supplemental data 3). Among the four teosinte lines, PNUE was high in PI 441903 and Ames 8083 and low in PI 615697 and Ames 21875. PNUE was not correlated with any gas-exchange or physiological traits except leaf N content (Table ). The δ13C values were not correlated with any gas exchange or physiological traits (Figure ; Table ).
Activities of photosynthetic enzymes
PEPC activity was not significantly correlated with PN (Figure (A)), whereas NADP-ME and Rubisco activities were positively correlated with PN (Figure (B) and (D)). PCK activity varied considerably among the lines (Supplemental data 4) and was high in two lines with high PN (PI 195114 and CM 109; Supplemental data 4). The sum of NADP-ME and PCK activities (capacity for C4 acid decarboxylation) varied among lines, with a maximum-to-minimum ratio of 3.0 (Supplemental data 4). PCK activity and the capacity for C4 acid decarboxylation were positively correlated with PN (Figure (C); Table ). However, the contribution ratio of PCK activity to the total C4 acid decarboxylation capacity (PCK ratio) was not correlated with PN (Table ). Chl content and LSP were positively correlated with the activities of Rubisco, NADP-ME, and PCK but not with that of PEPC (Table ).
Figure 4. Relationships between PN and activities of (A) PEP carboxylase (PEPC), (B) NADP-malic enzyme (NADP-ME), (C) PEP carboxykinase (PCK), and (D) Rubisco in leaves of maize and teosinte lines.
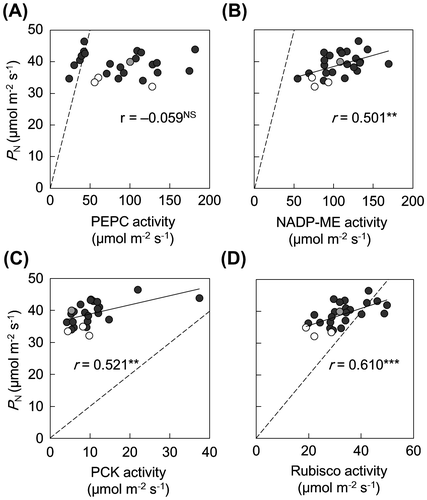
Structural traits
Variations in leaf thickness, IVD, and GL were small, with maximum-to-minimum ratios of 1.52 for leaf thickness, 1.40 for IVD, and 1.35 for GL (Supplemental data 5). Variations in SD and SD × GL were larger, with maximum-to-minimum ratios of 2.43 for SD and 2.26 for SD × GL (Supplemental data 5). The ratio of adaxial to abaxial GL was 1.04 ± .02 and the ratio of adaxial to abaxial SD was .71 ± .06 (data not shown). There were no correlations between these structural traits and gas-exchange or physiological traits (Supplemental data 6), except that PWUE was weakly negatively correlated with SD (r = –.412; p < .05) and SD × GL (r = –.415; p < .05). On the other hand, leaf thickness was positively correlated with IVD, whereas SD was negatively correlated with GL (Table ).
Table 3. Correlation coefficients (r) from linear regression analysis and statistical significance of the relationships between structural traits of maize and teosinte lines. IVD, interveinal distance; SD, stomatal density; GL, guard cell length.
Discussion
Physiological traits
Our study showed that PN in maize and teosinte lines ranged from 32.1–46.5 μmol m−2 s−1, a factor of 1.45 times. Although some studies have reported a variation of >2 times in PN of maize genotypes (Crosbie et al., Citation1977; Heichel & Musgrave, Citation1969), we found no such large difference. On the other hand, Duncan and Hesketh (Citation1968) reported a variation in PN among maize cultivars similar to that in our study. It seems likely that the differences in growth condition, plant age, and measurement method of PN bring about such different results.
We found a positive correlation between PN and gs (Figure (A)). This relationship has been reported in various C3 and C4 species (Fernandez et al., Citation2015; Tsutsumi et al., Citation2017; Wong et al., Citation1985). Because maize has a CCM, CO2 diffusion in stomata may not be the primary PN regulator. The PN of C4 plants is saturated at the Ci observed at the atmospheric CO2 concentration, whereas the PN of C3 plants is not saturated until a much higher CO2 concentration (Pearcy & Ehleringer, Citation1984; Wong et al., Citation1985). In preliminary measurements of some maize lines, we also confirmed that PN is saturated at Ci ≈ 100 μmol mol−1, which is observed at Ca = 380 μmol mol−1 (data not shown). This suggests that PN would not greatly increase even though gs alone increased and thereby higher Ci was attained within the leaf. Therefore, the effect of non-stomatal factors on the genetic variation in PN of maize and teosinte lines cannot be ruled out. On the other hand, there was a close relationship between PN and gs in maize lines. The reason is unknown. However, the coordinated mechanism between mesophyll photosynthesis and stomata (Lawson et al., Citation2014) may be involved in this relationship. According to this hypothesis, the concentration of CO2 inside the leaf would help maintain the coordination of the mesophyll photosynthesis with stomatal aperture (Lawson et al., Citation2014).
The Chl, LSP, and leaf N contents were positively correlated with PN (Figure (C)–(E)), as reported in many plant species, including maize (Wong et al., Citation1985; Sage & Pearcy, Citation1987; Tsutsumi et al., Citation2017), because all these parameters are closely associated with the contents of photosynthetic pigments and enzymes. No significant correlation was detected between PN and SLW (Figure (F)), as in Saccharum (Nose et al., Citation1994) and Oryza species (Kiran et al., Citation2013), although positive correlations were found in leguminous (Pearce et al., Citation1969), cruciferous (Suresh et al., Citation1997), and Amaranthus species (Tsutsumi et al., Citation2017). Thus, the relationship between PN and SLW varies with species.
Biochemical traits
PN was positively correlated with activities of NADP-ME, PCK, and Rubisco, but not with that of PEPC (Figure ). A positive correlation between PN and Rubisco activity was found in C4 and C3 species (Ghannoum et al., Citation2011; Tsutsumi et al., Citation2017; von Caemmerer et al., Citation1997). In our study, a considerable number of maize and teosinte lines showed lower Rubisco activities than PN (Figure (D)). Baer and Schrader (Citation1985) reported that, in Rubisco of maize lines, total activities are lower than initial activities. Therefore, this enzymatic trait may be involved in the lower Rubisco activities relative to PN, because we measured total activities. However, the possibility of deactivation and/or degradation of Rubisco during extraction cannot be ruled out. In NADP-ME-type C4 grasses, a positive correlation between PN and NADP-ME activity was also reported (Nose et al., Citation1994; Usuda, Citation1984). Using antisense RNA, Pengelly et al. (Citation2012) suggested that NADP-ME is unlikely to be a rate-limiting enzyme in C4 photosynthesis of Flaveria bidentis, an NADP-ME-type C4 dicot, because PN of transgenic plants was not significantly reduced until 40% reduction of NADP-ME activity in the wild-type plants. At present, it is unknown whether this relationship between NADP-ME activity and PN found in the C4 dicot is applicable to NADP-ME-type C4 grasses as well. However, these data suggest that the relationship between NADP-ME activity and PN may more or less differ among species.
Maize has two C4 acid decarboxylation enzymes, NADP-ME and PCK (Walker et al., Citation1997; Wingler et al., Citation1999). Our study has revealed, for the first time, a considerable genetic variation in PCK activity among maize and teosinte lines. The PCK system in maize has been estimated to contribute 10–14% of the carbon in BS (Arrivault et al., Citation2017). This ratio was within the range found in our study (3.6–19.8%; Supplemental data 4).
The physiological significance of C4 acid decarboxylation depending on PCK in maize remains to be understood (Furbank, Citation2011). Sharwood et al. (Citation2014) reported that the ratio of PCK activity to NADP-ME activity changes in maize exposed to shade and salinity. The parallel operation of the two C4 acid decarboxylation systems may compensate each other under certain environmental conditions (Bellasio & Griffiths, Citation2014) and may be energetically profitable. The C4 acid decarboxylation depending on PCK generates phosphoenolpyruvate (PEP). If PEP is transported to mesophyll cells, ATP needed for the conversion of pyruvate to PEP by PPDK may be saved. We detected no positive correlation between PN and PCK ratio in maize and teosinte lines (Table ). Therefore, the difference in PN among maize and teosite lines would not result from the difference in the contribution ratio of PCK activity to the total C4 acid decarboxylaton capacity. Further investigation would be required to understand the physiological significance of decarboxylation by PCK in maize.
Our study suggests that biochemical processes from C4 acid decarboxylation to re-fixation of CO2 by Rubisco are involved in the genetic variation in PN. However, we did not examine the activity of PPDK, which is well known to be the rate-limiting factor in C4 photosynthesis (Baer & Schrader, Citation1985; Usuda, Citation1984; von Caemmerer & Furbank, Citation2016), because a preliminary study on several lines of maize showed that their activities of PPDK were unstable and unreliable. The relationships between PN and PPDK activity and between PPDK and PCK activities remain to be explored.
Structural traits
PN was not correlated with leaf thickness or IVD (Supplemental data 6). In C3 plants, photosynthesis is performed within single mesophyll cells. Therefore, C3 leaves can change their structure in response to environmental change. The genetic variation in leaf structure of C4 plants is restricted to a narrower range than that of C3 plants, because C4 photosynthesis requires a strict quantitative balance between two types of photosynthetic cells (Dengler et al., Citation1994; Ghannoum et al., Citation2011; Tsutsumi et al., Citation2017). The maximum-to-minimum ratios of leaf thickness and IVD in maize and teosinte lines were generally smaller than those of physiological and biochemical traits. This may conceal the possible relationships between PN and these structural traits.
We found a negative correlation between SD and GL (Table ), as reported in other species (Büssis et al., Citation2006; Lawson & Blatt, Citation2014; Tsutsumi et al., Citation2017). The genetic variation in SD (2.43 times) was greater than that in GL (1.35 times; Supplemental data 5), suggesting physical and genetic limitations on the range of alterations in GL, whereas SD is much more flexible (Tsutsumi et al., Citation2017).
The stomatal parameters were not correlated with PN, gs, or Tr (Table ). It seems that an increase in SD would increase PN by increasing gs. Several studies reported positive correlations between SD and PN in various species, but other studies reported no such correlations (reviewed by Lawson & Blatt, Citation2014). Importantly, stomatal anatomical features such as SD and GL define the maximum theoretical conductance, whereas PN is the actual physiological outcome (Dow et al., Citation2014; Lawson & Blatt, Citation2014). The relationships between stomatal anatomical features and gas exchange parameters would also be affected by environmental factors such as vapor pressure deficit (Kawamitsu et al., Citation2002). Therefore, more detailed analyses under different conditions would be needed.
Resource use efficiency
C4 plants can maintain high CO2 concentration within BS cells owing to the CCM. This allows C4 plants to have higher PNUE than that of C3 plants (Brown, Citation1977; Ghannoum et al., Citation2011). A comparative study of C4 subtypes in grasses showed higher PNUE in the NADP-ME type than in the NAD-ME type, because Rubisco turnover rate is faster in the former than in the latter (Ghannoum et al., Citation2005). In our study, the maximum-to-minimum PNUE ratio of maize and teosinte lines was 1.69, and the mean was 700 μmol mol N−1 s−1 (Supplemental data 3), which was far higher than PNUE in C3 plants and was in the highest class of PNUE values previously reported in C4 plants (Ghannoum et al., Citation2005; Taylor et al., Citation2010; Togawa & Ueno, Citation2015; Tsutsumi et al., Citation2017; Vogan & Sage, Citation2011). Our study suggests that the genetic variation in PNUE in maize and teosinte lines depends on leaf N content but not on PN (Table ), because lines with lower leaf N content showed higher PNUE. Wild relatives of cultivated crops often inhabit more severe environment than cultivated conditions and possess useful traits that have been lost in cultivated crops during domestication (Hamaoka et al., Citation2013; Scafaro et al., Citation2010). Therefore, we expected that some teosinte lines would have higher PNUE than maize lines, but found no such trend (Figure. (C)).
The maximum-to-minimum PWUE ratio of maize and teosinte lines was 1.34 times (Supplemental data 2), which was lower than that of PNUE. The mean PWUE was 6.07 μmol mol−1 (Supplemental data 2) and was in the lower class of PWUE values previously reported in C4 plants (Osmond et al., Citation1982; Togawa & Ueno, Citation2015; Tsutsumi et al., Citation2017).
In C3 plants, there is a positive correlation between δ13C values and PWUE, and δ13C is useful for screening cultivars for high PWUE (Farquhar & Richards, Citation1984). In this study, the δ13C values did not vary greatly among maize and teosinte lines (Supplemental data 3) and were not significantly correlated with PWUE (Figure ). In sorghum (C4), O’Leary (Citation1988) also found no significant correlation between δ13C values and PWUE in 120 lines, whereas Henderson et al. (Citation1998) reported a weak but significant correlation in 30 lines. The carbon isotope ratio in plant dry matter reflects carbon isotope discrimination (Δ) during photosynthesis (Cernusak et al., Citation2013). In C3 plants, Δ is well correlated with Ci/Ca and hence can be used as an index of PWUE. In C4 plants, the variation in Ci/Ca is smaller than in C3 plants and Δ is related to both CO2 leakiness from BS cells and to Ci/Ca (Cernusak et al., Citation2013; Ghannoum et al., Citation2011; Henderson et al., Citation1998). For these reasons, it would not be easy to use δ13C values as an indicator of PWUE of C4 plants.
We found weak negative correlations between PWUE and SD or SD × GL (Table ). A decrease in SD would reduce water loss from leaves. However, it may also decrease PN while increasing PWUE, because PN is negatively correlated with PWUE (Table ). It is worth noting that all teosinte lines except Z. nicaraguensis (PI 615697) showed higher PWUE values than those of cultivated maize lines. Z. nicaraguensis is known to grow in wet habitats such as coastal, estuarine, and river environments (Bird, Citation2000; Iltis & Benz, Citation2000). The lower PWUE in Z. nicaraguensis may reflect these ecological traits.
Conclusion
This study investigated the genetic variations in photosynthetic rate and resource use efficiency in maize and teosinte lines and the regulatory factors involving in these variations. PN was positively correlated with physiological traits of leaves such as gs, Tr, and Chl and N contents. However, gs may not be the primary PN regulator, because maize has a CCM. PN was also positively correlated with activities of NADP-ME, PCK, and Rubisco, but not with that of PEPC. These data suggest that biochemical processes from C4 acid decarboxylation to re-fixation of CO2 by Rubisco are involved in the genetic variation in PN. On the other hand, structural traits of leaves such as leaf thickness, IVD, and stomatal parameters were not correlated with PN. It is suggested that physiological and biochemical traits are involved in the genetic variation of PN in maize and teosinte lines but structural traits are not directly involved. In maize and teosinte lines, PNUE was in the highest class and PWUE was in lower class of values previously reported in other C4 plants. It is worth noting that PNUE was negatively correlated with leaf N content. Some teosinte lines showed higher PWUE and have a value as genetic resources. The photosynthetic traits of maize may be also regulated by other factors not examined in this study, such as electron transport and CO2 leakiness from BS cells. Further studies will be required for our better understanding of the genetic variation in photosynthetic traits in maize.
Disclosure statement
No potential conflict of interest was reported by the authors.
Funding
This work was supported by the Japan Society for the Promotion of Science KAKENHI [grant number JP 16H04868], [grant number JP24380010] to O.U.
Supplemental data
Supplementary material for this article can be accessed at https://doi.org/10.1080/1343943X.2017.1398050
TPPS_1398050_Supplementary_data_2-6.docx
Download MS Word (46.7 KB)TPPS_1398050_Supplementary_data_1.TIF
Download TIFF Image (5.5 MB)TPPS_1398050_Supplementary_data_1.TIF
Download TIFF Image (6 MB)TPPS_1398050_Supplementary_data_1.TIF
Download TIFF Image (6.1 MB)Acknowledgements
We thank the Plant Introduction Station (Agricultural Research Service, USDA) and the NARO Genebank, Tsukuba, Japan, for their kind gifts of maize and teosinte seeds.
References
- Arnon, D. I. (1949). Copper enzymes in isolated chloroplast: Polyphenoloxidase in Beta vulgaris. Plant Physiology, 24, 1–15. doi:10.1104/pp.24.1.1
- Arrivault, S., Obata, T., Szecówka, M., Mengin, V., Guenther, M., Hoehne, M., … Stitt, M. (2017). Metabolite pools and carbon flow during C4 photosynthesis in maize: 13CO2 labeling kinetics and cell type fractionation. Journal of Experimental Botany, 68, 283–298. doi:10.1093/jxb/erw414
- Baer, G. R., & Schrader, L. E. (1985). Relationships between CO2 exchange rates and activities of pyruvate, Pi dikinase and ribulose bisphosphate carboxylase, chlorophyll concentration, and cell volume in maize leaves. Plant Physiology, 77, 612–616. doi:10.1104/pp.77.3.612
- Bellasio, C., & Griffiths, H. (2014). The operation of two decarboxylases, transamination, and partitioning of C4 metabolic processes between mesophyll and bundle sheath cells allows light capture to be balanced for the maize C4 pathway. Plant Physiology, 164, 466–480. doi:10.1104/pp.113.228221
- Bird, R. M. C. K. (2000). A remarkable new teosinte from Nicaragua: Growth and treatment of progeny. Maize Genetic Cooperation Newsletter, 74, 58–59 . Retrieved from https://www.cabdirect.org/cabdirect/abstract/20001613170
- Bradford, M. M. (1976). A rapid and sensitive method for the quantitation of microgram quantities of protein utilizing the principle of protein-dye binding. Analytical Biochemistry, 72, 248–254. doi:10.1016/0003-2697(76)90527-3
- Brown, R. H. (1977). A difference in N use efficiency in C3 and C4 plants and its implications in adaptation and evolution. Crop Science, 18, 93–98. doi:10.2135/cropsci1978.0011183X001800010025x
- Brown, R. H. (1999). Agronomic implications of C4 photosynthesis. In R. F. Sage & R. K. Monson (Eds.), C4 Plant Biology (pp. 473–507). San Diego, CA: Academic Press.10.1016/B978-012614440-6/50015-X
- Büssis, D., von Groll, U., Fisahn, J., & Altmann, T. (2006). Stomatal aperture can compensate altered stomatal density in Arabidopsis thaliana at growth light conditions. Functional Plant Biology, 33, 1037–1043. doi:10.1071/FP06078
- Carpita, N. C., & McCann, M. C. (2008). Maize and sorghum: Genetic resources for bioenergy grasses. Trends in Plant Science, 13, 415–420. doi:10.1016/j.tplants.2008.06.002
- Cernusak, L. A., Ubierna, N., Winter, K., Holtum, J. A. M., Marshall, J. D., & Farquhar, G. D. (2013). Environmental and physiological determinants of carbon isotope discrimination in terrestrial plants. New Phytologist, 200, 950–965. doi:10.1111/nph.12423
- Crosbie, T. M., Mock, J. J., & Pearce, R. B. (1977). Variability and selection advance for photosynthesis in Iowa stiff stalk synthetic maize population. Crop Science, 17, 511–514. doi:10.2135/cropsci1977.0011183X001700040007x
- Dengler, N. G., Dengler, R. E., Donnelly, P. M., & Hattersley, P. W. (1994). Quantitative leaf anatomy of C3 and C4 grasses (Poaceae): Bundle sheath and mesophyll surface area relationships. Annals of Botany, 73, 241–255. doi:10.1006/anbo.1994.1029
- Dow, G. L., Bergmann, D. C., & Berry, J. A. (2014). An integrated model of stomatal development and leaf physiology. New Phytologist, 201, 1218–1226. doi:10.1111/nph.12608
- Duncan, W. G., & Hesketh, J. D. (1968). Net photosynthetic rates, relative leaf growth rates, and leaf numbers of 22 races of maize grown at eight temperatures. Crop Science, 8, 670–674. doi:10.2135/cropsci1968.0011183X000800060009x
- Evans, J. R. (2013). Improving photosynthesis. Plant Physiology, 162, 1780–1793. doi:10.1104/pp.113.219006
- Farquhar, G. D., & Richards, R. A. (1984). Isotopic composition of plant carbon correlates with water-use efficiency of wheat genotypes. Australian Journal of Plant Physiology, 11, 539–552. doi:10.1071/PP9840539
- Fernandez, M. G. S., Strand, K., Hamblin, M. T., Westgate, M., Heaton, E., & Kresovich, S. (2015). Genetic analysis and phenotypic characterization of leaf photosynthetic capacity in a sorghum (Sorghum spp.) diversity panel. Genetic Resources and Crop Evolution, 62, 939–950. doi:10.1007/s10722-014-0202-6
- Flood, P. J., Harbinson, J., & Aarts, M. G. M. (2011). Natural genetic variation in plant photosynthesis. Trends in Plant Science, 16, 327–335. doi:10.1016/j.tplants.2011.02.005
- Furbank, R. T. (2011). Evolution of the C4 photosynthetic mechanism: Are there really three C4 acid decarboxylation types? Journal of Experimental Botany, 62, 3103–3108. doi:10.1093/jxb/err080
- Ghannoum, O., von Caemmerer, S., & Conroy, J. P. (2001). Carbon and water economy of Australian NAD-ME and NADP-ME C4 grasses. Australian Journal of Plant Physiology, 28, 213–223. doi:10.1071/PP00078
- Ghannoum, O., Evans, J. R., Chow, W. S., Andrews, T. J., Conroy, J. P., & von Caemmerer, S. (2005). Faster rubisco is the key to superior nitrogen-use efficiency in NADP-malic enzyme relatives to NAD-malic enzyme C4 grasses. Plant Physiology, 137, 638–650. doi:10.1104/pp.104.054759
- Ghannoum, O., Evans, J. R., & von Caemmerer, S. (2011). Nitrogen and water use efficiency of C4 plants. In A. S. Raghavendra & R. F. Sage (Eds.), C4 photosynthesis and related CO2 concentrating mechanisms (pp. 129–146). Dordrecht: Springer.
- Hamaoka, N., Uchida, Y., Tomita, M., Kumagai, E., Araki, T., & Ueno, O. (2013). Genetic variations in dry matter production, nitrogen uptake, and nitrogen use efficiency in the AA genome oryza species grown under different nitrogen conditions. Plant Production Science, 16, 107–116. doi:10.1626/pps.16.107
- Hatch, M. D. (1987). C4 photosynthesis: A unique blend of modified biochemistry, anatomy and ultrastructure. Biochimica et Biophysica Acta (BBA) – Reviews on Bioenergetics, 895, 81–106. doi:10.1016/S0304-4173(87)80009-5
- Heichel, G. H., & Musgrave, R. B. (1969). Varietal difference in net photosynthesis of Zea mays L. Crop Science, 9, 483–486. doi:10.2135/cropsci1969.0011183X000900040029x
- Henderson, S., von Caemmerer, S., Farquhar, G. D., Wade, L., & Hammer, G. (1998). Correlation between carbon isotope discrimination and transpiration efficiency in lines of the C4 species Sorghum bicolor in the glasshouse and the field. Australian Journal of Plant Physiology, 25, 111–123. doi:10.1071/PP95033
- Iltis, H. H., & Benz, B. F. (2000). Zea nicaraguensis (Poaceae), a new teosinte from pacific coastal Nicaragua. Novon, 10, 382–390. doi:10.2307/3392992
- Kawamitsu, Y., Hiyane, S., Tamashiro, Y., & Hakoyama, S. (2002). Regulation of photosynthesis and water use efficiency in relation to stomatal frequency and interveinal distance in C3- and C4-Grass Species. Environmental Control in Biology, 40, 365–374. doi:10.2525/ecb1963.40.365
- Kiran, T. V., Rao, Y. V., Subrahmanyam, D., Rani, N. S., Bhadana, V. P., Rao, P. R., & Voleti, S. R. (2013). Variation in leaf photosynthetic characteristics in wild rice species. Photosynthetica, 51, 350–358. doi:10.1007/s11099-013-0032-3
- Koteyeva, N. K., Voznesenskaya, E. V., & Edwards, G. E. (2015). An assessment of the capacity for phosphoenolpyruvate carboxykinase to contribute to C4 photosynthesis. Plant Science, 235, 70–80. doi:10.1016/j.plantsci.2015.03.004
- Lawson, T., & Blatt, M. R. (2014). Stomatal size, speed, and responsiveness impact on photosynthesis and water use efficiency. Plant Physiology, 164, 1556–1570. doi:10.1104/pp.114.237107
- Lawson, T., Simkin, A. J., Kelly, G., & Granot, D. (2014). Mesophyll photosynthesis and guard cell metabolism impacts on stomatal behavior. New Phytologist, 203, 1064–1081. doi:10.1111/nph.12945
- Leonardos, E. D., & Grodzinski, B. (2000). Photosynthesis, immediate export and carbon partitioning in source leaves of C3, C3–C4 intermediate and C4 Panicum and Flaveria species at ambient and elevated CO2 levels. Plant, Cell and Environment, 23, 839–851. doi:10.1046/j.1365-3040.2000.00604.x
- McCouch, S. (2004). Diversifying selection in plant breeding. PLoS Biology, 2, e347. doi:10.1371/journal.pbio.0020347
- Nose, A., Uehara, M., & Kawamitsu, Y. (1994). Variations in leaf gas exchange traits of saccharum including feral sugarcane, Saccharum spontaneum L. Japanese Journal of Crop Science, 63, 489–495. doi:10.1626/jcs.63.489
- O’Leary, M. H. (1988). Carbon isotopes in photosynthesis. BioScience, 38, 328–336. doi: 10.2307/1310735
- Osmond, C. B., Winter, K., & Ziegler, H. (1982). Functional significance of different pathways of CO2 fixation in photosynthesis. In O. L. Lange, P. S. Nobel, & C. B. Osmond (Eds.), Encyclopedia of plant physiology, New series, Vol. 12B (pp. 479–547). Berlin: Springer Verlag.
- Pearce, R. B., Carlson, D. K., Barnes, D. K., Hart, R. H., & Hanson, C. H. (1969). Specific leaf weight and photosynthesis in alfalfa. Crop Science, 9, 423–426. doi:10.2135/cropsci1969.0011183X000900040010
- Pearcy, R. W., & Ehleringer, J. (1984). Comparative ecophysiology of C3 and C4 plants. Plant, Cell and Environment, 7, 1–13. doi:10.1111/j.1365-3040.1984.tb01194.x
- Pengelly, J. J. L., Tan, J., Furbank, R. T., & von Caemmerer, S. (2012). Antisense reduction of NADP-malic enzyme in Flaveria bidentis reduces flow of CO2 through the C4 cycle. Plant Physiology, 160, 1070–1080. doi:10.1104/pp.112.203240
- Sage, R. F. & Pearcy, R. W. (1987). The nitrogen use efficiency of C3 and C4 plants. II. Leaf nitrogen effects on the gas exchange characteristics of Chenopodium album (L.) and Amaranthus retroflexus (L.). Plant Physiology, 84, 959–963. doi:10.1104/pp.84.3.959
- Sato, R., & Suzuki, Y. (2010). Carbon and nitrogen stable isotope analysis by EA/IRMS. Researches in Organic Geochemistry, 26, 21–29. doi:10.20612/rog.26.0_21
- Scafaro, A. P., Haynes, P. A., & Atwell, B. J. (2010). Physiological and molecular changes in Oryza meridionalis Ng., a heat-tolerant species of wild rice. Journal of Experimental Botany, 61, 191–202. doi:10.1093/jxb/erp294
- Schneider, C. A., Rasband, W. S., & Eliceiri, K. W. (2012). NIH Image to ImageJ: 25 Years of image analysis. Nature Methods, 9, 671–675. doi:10.1038/nmeth.2089
- Sharwood, R. E., Sonawane, B. V., & Ghannoum, O. (2014). Photosynthetic flexibility in maize exposed to salinity and shade. Journal of Experimental Botany, 65, 3715–3724. doi:10.1093/jxb/eru130
- Suresh, K., Rao, K. L. N., & Nair, T. V. R. (1997). Genetic variability in photosynthetic rate and leaf characters in Brassicaceae coenospecies. Photosynthetica, 33, 173–178. doi:10.1023/A:1022147910426
- Taylor, S. H., Hulme, S. P., Rees, M., Ripley, B. S., Woodward, F. I., & Osborne, C. P. (2010). Ecophysiological traits in C3 and C4 grasses: A phylogenetically controlled screening experiment. New Phytologist, 185, 780–791. doi:10.1111/j.1469-8137.2009.03102.x
- Togawa, Y., & Ueno, O. (2015). Comparison of resource use efficiencies involved in photosynthesis among biochemical subtypes of C4 grasses. Abstracts of 239th Meeting of the Crop Science Society of Japan, Tokyo. (p. 209).
- Tsutsumi, N., Tohya, M., Nakashima, T., & Ueno, O. (2017). Variations in structural, biochemical, and physiological traits of photosynthesis and resource use efficiency in Amaranthus species (NAD-ME-type C4). Plant Production Science, 20, 300–312. doi:10.1080/1343943X.2017.1320948
- Ueno, O., & Sentoku, N. (2006). Comparison of leaf structure and photosynthetic characteristics of C3 and C4 Alloteropsis semialata subspecies. Plant, Cell and Environment, 29, 257–268. doi:10.1111/j.1365-3040.2005.01418.x
- Ueno, O., Kawano, Y., Wakayama, M., & Takeda, T. (2006). Leaf vascular systems in C3 and C4 grasses: A two-dimensional analysis. Annals of Botany, 97, 611–621. doi:10.1093/aob/mcl010
- Usuda, H. (1984). Variations in the photosynthetic rate and activity of photosynthetic enzymes in maize leaf tissue of different ages. Plant and Cell Physiology, 25, 1297–1301. doi:10.1093/oxfordjournals.pcp.a076838
- Usuda, H., Ku, M. S. B., & Edwards, G. E. (1985). Influence of light intensity during growth on photosynthesis and activity of several key photosynthetic enzymes in a C4 plant (Zea mays). Physiologia Plantarum, 63, 65–70. doi:10.1111/j.1399-3054.1985.tb02819.x
- Vogan, P. J., & Sage, R. F. (2011). Water-use efficiency and nitrogen-use efficiency of C3–C4 intermediate species of Flaveria Juss (Asteraceae). Plant, Cell and Environment, 34, 1415–1430. doi:10.1111/j.1365-3040.2011.02340.x
- von Caemmerer, S., & Furbank, R. T. (2016). Strategies for improving C4 photosynthesis. Current Opinion in Plant Biology, 31, 125–134. doi:10.1016/j.pbi.2016.04.003
- von Caemmerer, S., Millgate, A., Farquhar, G. D., & Furbank, R. T. (1997). Reduction of ribulose-1,5-bisphosphate carboxylase/oxygenase by antisense RNA in the C4 plant Flaveria bidentis leads to reduced assimilation rates and increased carbon isotope discrimination. Plant Physiology, 113, 469–477. doi:10.1104/pp.113.2.469
- Walker, R. P., Acheson, R. M., Técsi, L. I., & Leegood, R. C. (1997). phosphoenolpyruvate carboxykinase in C4 plants: Its role and regulation. Australian Journal of Plant Physiology, 24, 459–468. doi:10.1071/PP97007
- Wingler, A., Walker, R. P., Chen, Z. H., & Leegood, R. C. (1999). phosphoenolpyruvate carboxykinase is involved in the decarboxylation of aspartate in the bundle sheath of maize. Plant Physiology, 120, 539–545. doi:10.1104/pp.120.2.539
- Wong, S. C., Cowan, I. R., & Farquhar, G. D. (1985). Leaf conductance in relation to rate of CO2 assimilation. 1. Influence of nitrogen nutrition, phosphorous nutrition, photon flux density, and ambient partial pressure of CO2 during ontogeny. Plant Physiology, 78, 821–825. doi:10.1104/pp.78.4.830
- Zhu, X. G., Long, S. P., & Ort, D. R. (2010). Improving photosynthetic efficiency for greater yield. Annual Review of Plant Biology, 61, 235–261. doi:10.1146/annurev-arplant-042809-112206