ABSTRACT
The sugar-accumulating potential of global and local sweet and grain sorghum varieties were tested under the local conditions. The basis for this study was the dependency of sugar accumulation on temperature and photoperiod. Thus, the efficacy of cultivars as a bioenergy source would need to be determined based on their performance under the local environmental conditions. A strong correlation of sucrose content with brix was observed, enabling large-scale screening of varieties for high sucrose content. The morphological characteristics inherent in sweet sorghum, such as tall stems, greater number of leaves and a longer vegetative period, were found to correlate with the total stem sugar content. Assessment of sugars along the stem revealed maximum sugar accumulation in the upper intermediate to upper internodes in most of the varieties tested. The maximum theoretical ethanol yield (MTEY), a function of brix and juice yield, was determined as a better indicator of testing the performance of a variety as a potential source of bioethanol, mainly due to a negative correlation of stem juiciness and sucrose content in the varieties tested. Further, the relative expression of vacuolar invertase genes, SbINV1 and SbINV2, was studied, and a strong negative correlation of SbINV2 to stem sucrose content was observed. This reveals a possibility of involvement of vacuolar invertase gene, SbINV2, in sugar accumulation in sweet sorghum stems, and as a key candidate for molecular breeding studies for higher stem sugar content.
Introduction
Sorghum (Sorghum bicolor (L.) Moench) is the fifth most important cereal crop in the world (Paterson, Citation2008) and serves as a staple food in many countries of Asia and Africa (Mace et al., Citation2013). They can be classified into four types based on morphological traits and usage, namely, grain sorghum, sweet sorghum, forage sorghum and energy sorghum (Mathur et al., Citation2017). While grain sorghums are cultivated for their grain, forage sorghums are mainly used as animal feed and silage (Shoemaker & Bransby, Citation2010). Sweet sorghums are found to accumulate sugars in their stalk, however, with lower grain yield when compared to the grain sorghum genotypes, while energy sorghums are genotypes that are bred mainly for bioenergy purposes due to their high lignocellulosic biomass. Various factors such as high resilience to harsher climates, ease of extraction, high energy use efficiency and high sugar-accumulating capacity have driven attention to sweet sorghum, as a competitive bioenergy crop.
Sweet sorghum is well adapted to the semi-arid regions and accumulates sugars in its stem during the rainy season but fails to do so at the same level during post-rainy season. Environmental factors such as light and water availability and temperature influence the sugar-accumulating potential of sorghum (Qazi et al., Citation2012; Rutto et al., Citation2013). Thus, selection of high sugar-yielding varieties suitable for bioethanol production is determined by its performance under the local environmental conditions.
Sugars are stored in the vacuoles of the storage parenchyma cells of sugar-accumulating species such as sugarcane (Etxeberria et al., Citation2012) and sugar beet (Leigh, Citation1984), where, in sugarcane the vacuoles constitute a major proportion of the storage parenchyma cells (Palaniswamy et al., Citation2016). Vacuolar invertases (VINs), a group of invertases (EC 3.2.1.26), catalyse the irreversible cleavage of sucrose to glucose and fructose in the vacuole. Its localization in the storage compartment of the cell has drawn attention towards its roles in sugar accumulation in grass species.
Studies in the past have demonstrated roles for VIN (Qazi et al., Citation2012; Yang et al., Citation2013) in sugar accumulation in sweet sorghum stems. This was in concert with another study that demonstrated a marked role for a VIN, SbINV2, in sugar accumulation in sweet sorghums, based on comparative transcriptome analysis of genes related to sugar metabolism between a grain sorghum and a sweet sorghum variety (T. Kurai, personal communication, 14 May 2014). With respect to expression of genes in the sugar metabolism pathway in grass species, roles for sucrose phosphate synthase (Botha and Black, Citation2000; Grof et al., Citation2007; Qazi et al., Citation2012; Yang et al., Citation2013; Zhu et al., Citation1997), VIN (Liu et al., Citation2014; McKinley et al. Citation2016; Qazi et al., Citation2012; Yang et al., Citation2013; Zhu et al., Citation1997), sucrose synthase (McKinley et al., Citation2016; Qazi et al., Citation2012), tonoplast-sugar transporter (Bihmidine et al., Citation2016) and sucrose transporter (Qazi et al., Citation2012) in stem sugar accumulation have been identified. Despite these findings, an inconsistency in the involvement of enzymes in sugar accumulation in sorghum is observed, mainly due to the difference in genotypes used, and the influence of abiotic external factors on gene expression.
The dependency of stem sugar content on environmental factors and the possible role of VIN on its estimation form the basis of the study conducted. We also evaluate the performance of varieties for stem sugar content and bioethanol-yielding capacity, with correlation studies between the morphological characters and stem sucrose content.
Materials and methods
Plant materials and growth conditions
Twenty sorghum varieties inclusive of 12 locally available commercial varieties including four grain sorghum varieties (GS-2, High grain, Meter sorghum and Mini sorghum), eight sweet sorghum varieties (Big sugar, BMR sweet, DH, Hachimitsu, Kanmi, Supersugar, TDN and Toumitsu) and eight global sweet sorghum varieties (Arjuna, Bromo, Cowley, Keller, M81-E, Rio, Roma and Wray) were grown in an experimental field at the Institute for Sustainable Agro-ecosystem Services (ISAS), The University of Tokyo (35.73ºN, 139.53ºE, 58 m above sea level), in Tokyo, Japan (). In 2015, nine of the 20 varieties (Cowley, Keller, Meter sorghum, Mini sorghum, Rio, Roma, Supersugar, TDN and Wray) were tested, while in 2016 all 20 varieties were assessed. Fertilizers were applied at 12 g N m−2, 12 g P2O5 m−2 and 12 g K2O m−2 as basal dressing. Cultivation was carried out between months of June and November, with sowing on 17 June 2015 and 1 June 2016 under rainfed conditions. The same field was used both years with a fallow period of ~6 months (December to June). The experiments were arranged as completely randomized block designs with three replications. A row spacing of 70 cm and plant-to-plant spacing of 20 cm was maintained resulting in an average planting density of 6.3 plants m−2.
Plant samplings
Sampling for RNA, non-structural carbohydrates (NSCs), juice weight estimation and determination of the morphological characteristics was performed at the late soft dough stage, a stage where kernels can be squeezed between fingers forming dough-like texture without any liquid. The lowermost internode was cryogenically sampled and placed in −80ºC until use. The choice of lowermost internodes for NSC and transcript level studies, despite maximum sugar accumulation in the intermediate to upper internodes, was solely for ease of sampling and maintaining consistency among differently heighted varieties.
Estimation of morphological characteristics
Plant height, number of leaves, number of tillers, stem girth, panicle length and days to flowering were determined for all the varieties. Plant height was measured from the base to the tip of the panicle, and panicle length was measured from the base of the peduncle to the tip of the panicle. Stem girth was determined by measuring the length of a thin rope that could snugly be wrapped around the base of the stem. Days to flowering were determined as the number of days from the day of sowing to the day of 30% flowering.
Determination of brix, stem juice content, stem juiciness and maximum theoretical ethanol yield (MTEY)
Brix (%) was measured for each internode, using ~1 ml juice obtained by squeezing ~1 cm of the mid-internode using a hand-held refractometer (PAL-1, ATAGO, Tokyo, Japan). Whole stem brix was estimated as an average of individual brix values obtained for corresponding internodes of the stem. Stem juice content (g cm−1) was determined as the difference in weights of a fixed length of the lower stem before and after drying at 80°C for a minimum of 10 days. This value of stem juice content was divided by the fresh weight of the sample to obtain stem juiciness (g gFW−1). The total juice weight per plant (g) was estimated as a product of the stem juice content (g cm−1) and the harvestable stalk length (cm).
Juice yield (L ha−1) was estimated by eq. (1) and MTEY by eq. (2) (Almodares & Hadi, Citation2009).
where total juice weight per plant was obtained in litres (L), considering the density of juice as 1.0 for ease of calculation.
Determination of starch and soluble sugar contents
The lowermost internodes that were sampled were cryogenically pulverized using a Multi-beads shocker (Yasui Kikai, Osaka, Japan) at 2,500 rpm for 15 s, twice. NSC (starch, sucrose, glucose and fructose) contents were determined as described previously (Okamura et al., Citation2013).
Quantitative real-time PCR
RNA was isolated using the CTAB:CI mixture (1:1 v/v), with incubation at 65ºC for 10 min. The aqueous layer was mixed with equal volume of isopropanol, followed by centrifugation at 11,000 g for 15 min at 4ºC. The obtained pellet was washed with 70% ethanol and resuspended with RNase-free water after drying. Purification of RNA was performed using the RNeasy Plant Mini kit (QIAGEN, Germany), followed by reverse transcription using a PrimeScript RT reagent kit with gDNA eraser (Perfect Real Time, Takara Bio). 1 µl of the cDNA synthesized was used for real-time PCR using SYBR Premix Ex Taq II (Tli RNase H Plus, Takara Bio) in a thermocycler (ABI7300, Thermo Fisher Scientific, Waltham, MA, USA) with an annealing temperature of 55°C. Primers specific to SbINV1, SbINV2 and EF1-a (Elongation factor 1-alpha) (Milne et al., Citation2013) are listed in . The GenBank database accession numbers for SbINV1 (Sb06g023760) and SbINV2 (Sb04g000620) used in this article are XM_002446812.2 and XM_021458735.1, respectively. mRNA transcript abundance was determined in terms of %EF1-a using eq. (3).
Table 1. Primers used for real-time PCR.
Statistical analyses
All statistical analyses were performed in Microsoft Excel 2016. The statistical differences between the years were determined using the Student’s T statistic. Pearson’s coefficient of correlation was used for correlation studies described in this study.
Results
Sugar accumulation pattern in sorghum stems
Total NSC content constituting total soluble sugar content and starch content was determined for the varieties (), where Cowley and Roma consistently fared as the high sucrose-yielding cultivars in both 2015 (Figure S1) and 2016 ()). While Supersugar accumulated more sucrose under early planting conditions in 2016 than in 2015 (Figure S1), TDN failed to accumulate sucrose in a comparable level under these conditions ()). The grain sorghum varieties Meter, Mini, GS2 and High grain, along with two short-heighted, early flowering sweet sorghum varieties BMR Sweet and TDN failed to accumulate sugars in their stems ()). Starch content in all the varieties were considerably lower and did not show any comparative trend to the sugar-accumulating potential of varieties assessed ()).
Figure 2. (a) NSC content in the lowermost internodes of sorghum stems and (b) pattern of sugar accumulation expressed in terms of brix for each internode along the whole length of the stem, for varieties grown in 2016. Data represent the mean ± SE (n=3–4). Varieties sorted on the basis of increasing sucrose content. The colour legend is not numerically universally applicable, but indicative of the high-to-low pattern of brix for each variety.
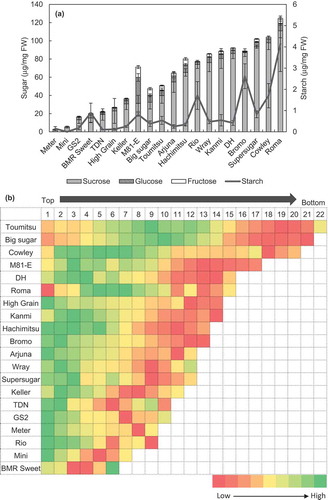
The varieties tested in 2015 showed an upward trend of brix from flowering to 30 DAF, a stage that marked the soft dough stage of grain development (Figure S2). Thus, this stage was used for further assessment of varieties for sugar accumulation. Brix for each internode was measured to determine the pattern of sugar accumulation along the length of the stem where maximum sugar accumulation was observed in the upper intermediate or uppermost internodes, with the exception of BMR sweet, an early flowering variety that showed maximum sugar accumulation at the lowermost internode ()).
Relationship between morphological characteristics and sugars in the stem
Plant height, number of leaves, number of tillers, stem girth, panicle length and days to flowering were recorded (Tables S1 and S2) and scored for correlation with sucrose and total sugar content in varieties assessed in 2015 and 2016 (). Consistent significance of correlation was obtained for plant height, number of leaves and days to flowering with total sugar content in both years, the correlating morphological traits being inherent with sweet sorghum. The varieties tested in 2015, which were sown later than those in 2016, tended to be taller and accumulate higher amount of total sugars in their stems mainly owing to the longer vegetative period in 2015 (Tables S1 and S2).
Table 2. Correlation between morphological characteristics and sucrose and total sugar content for varieties cultivated in 2015 and 2016. Pearson’s correlation coefficient is represented as r. Asterisks indicate statistical significance of correlation with *p < 0.05 and **p < 0.01 (n.s. – not significant).
Effect of climatic conditions on sugar accumulation
Difference in total sugars in the stems between the two years was observed, where, in 2015, i.e. the cultivation regime with a later sowing date, the total sugar content was markedly higher for all the varieties tested (). The sucrose content, however, showed inconsistency in its variability between years, where some varieties showed higher sucrose levels in 2015 (Meter, Mini, Keller and TDN), and some in 2016 (Supersugar and Wray), while a few others (Cowley, Rio and Roma) showed no differences in their stem sucrose content between the years (). Although this reconfirms the dependency of sugar accumulation potential on external environmental conditions, it also highlights a variation in the level of this dependency among varieties. Further, climate data including temperature, daily solar radiation, day–night temperature, and rainfall were analysed, and no significant differences in temperature and daily solar radiation between the two years (Figure S3A) were observed. However, rainfall in 2015, in the months of August and September, i.e. the period of flowering and the stages after, was lower than in 2016 (Figure S3C), while the day/night temperature difference towards the end of August was higher in 2016 when compared to 2015 (Figure S3B). Although minimal differences in climatic conditions between the years was observed, earlier sowing in 2016 highlights a difference in stage-specific exposure to certain climatic conditions between the years.
Figure 3. Differences in total soluble sugar content (shown as lines) and total sucrose content (shown as bars) in the lowermost internodes of sorghum stems for nine varieties between 2015 (black bars and dotted line) and 2016 (grey bars and solid line). Data represent the mean ± SE (n=3–4). Asterisks indicate statistical significance of difference between the years using Student’s T statistic with *p<0.05, **p<0.01 and ***p<0.001.
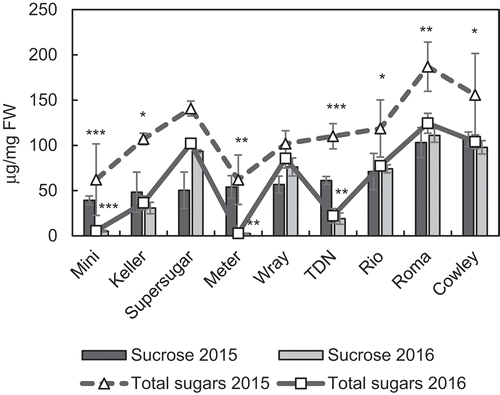
Relationship of brix and stem juiciness with sucrose content and estimation of the maximum theoretical ethanol yield (MTEY)
A strong positive correlation was found between brix and sucrose content in stems of the varieties cultivated ()), while a strong negative correlation was observed between stem juiciness and brix ()), where the grain sorghum varieties, GS2, Meter, Mini and High grain were found to possess the juiciest stems ()). MTEY was estimated and found to be highest for Toumitsu, with Cowley and Big sugar showing next higher comparable values ()). Despite higher brix, Roma and Cowley were unable to outperform Toumitsu in MTEY mainly due to their lower stem juiciness and relatively smaller plant height, contributing to a lower juice yield.
Figure 4. (a) Correlation of brix with sucrose content, (b) varieties sorted in terms of juiciness of the stems, (c) correlation of juiciness with brix and (d) the maximum theoretical ethanol yield. Data represent the mean ± SE (n=4–6) for varieties grown in 2016. r represents the Pearson’s coefficient of correlation and asterisks indicate the statistical significance of this correlation with **p<0.01.
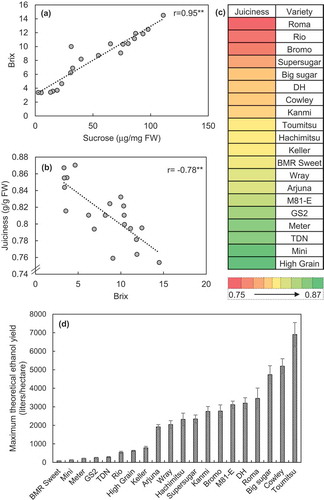
Relationship of SbInv1 and SbInv2 transcript levels and sucrose content in stems
mRNA abundance of SbINV1 ()) and SbINV2 ()) in the stems of the varieties tested was determined and plotted against their corresponding sucrose values (). Correlation between sucrose content and SbINV1 could not be established ()), while SbINV2 significantly negatively correlated with sucrose content in the stems of high sugar-yielding varieties ()), outlining a possible involvement of SbINV2 in determination of sucrose-accumulating capacity of sweet sorghums.
Figure 5. mRNA abundance of (a) SbINV1 and (b) SbINV2, expressed as %EF1a, and the corresponding sucrose content in their lowermost internodes. Expression levels are shown by bars and sucrose content by lines. Correlation of sucrose content with (c) SbINV1 and (d) SbINV2 for high sugar-yielding varieties is shown. Data represent the mean ± SE (n=3–4). r indicates the Pearson’s coefficient of correlation. Asterisks indicate statistical significance with ***p<0.001. Varieties sorted on the basis of increasing sucrose content. Data shown for 2016 with similar correlation data obtained in 2015.
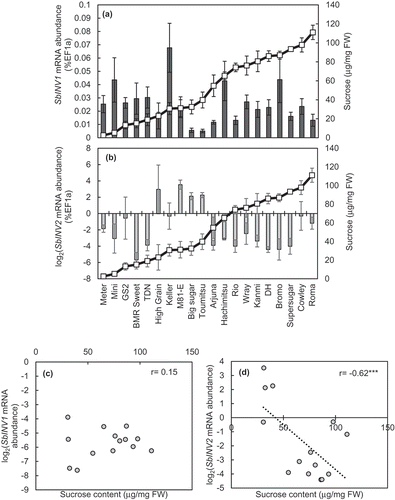
Discussion
Growth of sweet sorghum in temperate climates
Sweet sorghums are not adapted to temperate conditions; however, lately due to the development of photoperiod-insensitive lines, sorghums have been cultivated in temperate regions as well (Thurber et al., Citation2013). Sweet sorghums have been previously grown in the colder Northern regions of Japan, for stem biomass, with sowing in early to mid-June as ideal for maximum stem yield (Fujii et al., Citation2016; Nakamura et al., Citation2011). The period of growth from sowing to flowering in tropical regions is around 55–70 days, with an extension by 20–30 days in temperate conditions (Mocoeur et al., Citation2015; Rao et al., Citation2013), which was observed in the varieties grown in our study as well (Tables S1 and S2). Maximum stem sucrose accumulation is observed during the soft dough stage, which was observed around 30 DAF in the varieties used in this study (Figure S2). The physiological maturity was reached at 40–45 DAF in most of the varieties analysed in both years, with the late flowering varieties, Toumitsu and Big sugar, being unable to achieve grain maturity. The growth characteristics and sugar-accumulating stages observed in the varieties tested in the study were consistent with previous studies conducted in temperate climates, thus providing favourable cultivable climatic conditions for growth of sorghum in Tokyo.
Sucrose accumulation potential is dependent on multiple factors
Various morphological factors, such as the plant height and number of leaves, were found to positively correlate with the total stem sugar content (). Taller stems were deemed favourable for sugar accumulation, and thus, found to possess higher number of leaves. This suggested a possible increase in photosynthetic ability of taller sweet sorghum varieties compared to shorter grain sorghum varieties, a finding consistent with a previous study (Bihmidine et al., Citation2015). The panicle length negatively correlated, while the flowering date positively correlated with the total stem sugar content. A longer vegetative stage pointed towards taller plants with a higher ability of stems to accumulate sugar. Taller stems, higher number of leaves, shorter panicles and delayed flowering are traits that are typical to sweet sorghum and were found to correlate consistently with the total stem sugar content among all varieties.
Another key result obtained from this study was the differential expression of SbINV2 among varieties, with a significant negative correlation with sucrose content ()). It has been long believed that sweet sorghums store most of their carbohydrates as sugars in their stem, while grain sorghums store most of their carbohydrates in grain (McBee & Miller, Citation1982). Thus, it is expected that the grain quality in sweet sorghums is lower than that of grain sorghum, mainly due to a limited availability of carbohydrates for grain (Shukla et al., Citation2017). However, sweet sorghum genotypes with a higher or comparable grain yield to grain sorghums have been reported (Channappagoudar et al., Citation2007; Ganesh et al., Citation2010). Breeding studies to produce sweet sorghum varieties with higher grain yield and higher stem sucrose content in the stems have been a focus in recent times (Mathur et al., Citation2017; Shukla et al., Citation2017). Studies in the past have revealed the independent existence of stem internodes and the panicles as storage sinks in sweet sorghum genotypes (Murray et al., Citation2008; Rao et al., Citation2013). Absence of changes in the stem sugar content under elimination of one of the sinks, achieved through pruning or cytoplasmic male-sterile lines, supports the prevalence of independent physiological pathways in the stems and panicles (Gutjahr et al., Citation2013). Thus, the elimination of trade-off between grain yield and stem sugar yield in sweet sorghums can be achieved. The presence of independent physiological pathway in sweet sorghum stems paves way to the utilization of SbINV2 as a molecular marker for breeding varieties with high sucrose content in their stems. Further, full-length DNA sequencing of SbINV2 in the varieties analysed to isolate any polymorphisms could isolate the specific diversity in terms of divergence observed between the sweet sorghum and grain sorghum varieties, and among the sweet sorghum varieties.
Furthermore, a study focused on Quantitative trait locus (QTL) analysis for sugar-related agronomic traits between a grain and a sweet sorghum variety identified one of the QTLs for plant height, flowering date, panicle weight and brix to be located on chromosome 4 (Shiringani et al., Citation2010), the chromosome bearing SbINV2, a VIN isoform found to significantly negatively correlate among the sweet sorghum varieties tested in this study ()). Based on this QTL data, RNA sequencing study of a sweet sorghum variety SIL-05 was performed where almost negligible amounts of SbINV2 in its stems were observed (Mizuno et al., Citation2016). Although this points towards the coexistence of morphological traits native to sweet sorghums and lower expression levels of SbINV2, further studies are needed to determine the possibility of its independent or codependent existence for the sugar-accumulating trait.
The differences in total sugar content and the sucrose levels between two cultivation years were also observed (), which could be attributed to differed sowing dates causing a variation in climatic conditions during similar stages of growth between the years (Figure S3). Effects of planting date on sugar yield have been previously reported (Tovignan et al., Citation2016; Teetor et al., Citation2011), demonstrating a location-wise variability further strengthening the need for optimization of sowing dates for sugar yield. Although the interaction effects of different genotypes with sowing dates in terms of sugar yield have been studied in tropical climates (Reddy et al., Citation2014) showing different optimum sowing dates for different genotypes, cultivation of the varieties tested in this study under varied sowing regimes could prove valuable for sorghum cultivation in the Tokyo region.
Testing efficacy of varieties based on ethanol yield potential
MTEY, a function of brix and juice yield, was found to be the closest determinant for testing efficacy of varieties for bioethanol production. Brix was found to strongly positively correlate with the sucrose content ()), thus enabling the possibility of high throughput screening of varieties for high stem sugar content. A strong negative correlation was found between the stem juiciness and brix ()), implying that the varieties with lower brix content had juicier stems. Juice yield is a function of stem juiciness and stalk length; thus the taller varieties with moderate stem juiciness were found to have a higher juice yield. This suggested that while the varieties with higher brix values did fairly well in terms of MTEY, the varieties that maintained a balance with juice yield and brix, i.e. possessing moderate juice yield and moderate-to-high brix values, performed extremely well in terms of MTEY, as observed in this study (). Thus, in terms of evaluating varieties for their performance in sugar-accumulating potential in terms of bioethanol production, MTEY rather than brix values serves as a key determinant.
Conclusion
The current study assessed the performance of the local and global sorghum varieties under local environmental conditions and isolated the significance of both sucrose content and the stem juice yield in evaluating the efficacy of a variety for bioethanol production. Among the global varieties, Roma and Cowley fared well in terms of MTEY mainly due to a higher sucrose content in their stems, while the taller local varieties, Toumitsu and Big sugar, performed better than the global elite varieties, Roma and Cowley, mainly due to a higher juice yield and moderate levels of stem sucrose content. The morphological traits specific to sweet sorghum, such as plant height, number of leaves and days to flowering, were found to correlate strongly with the total sugar content in the stems, and a strong negative correlation of a vacuolar invertase, SbINV2, with stem sucrose content was also determined.
PPS2018_073RP-File009.pdf
Download PDF (145.3 KB)Acknowledgments
The authors would like to thank the technical support staff of the Institute for Sustainable Agro-ecosystem Services, The University of Tokyo for their cultivation management of sorghum. We also thank Dr. Satoshi Nakamura and Dr. Takashi Takamizo for their kind contribution of sorghum seeds, and Rei Tanimoto, Takashi Ohtaguro, Shota Tsujimoto, Haibo Zhang, Aoi Kamoshita, Makoto Umeda, Ryugo Yagi, Akihiro Matsumoto, Kosuke Sonoda and Toru Yamada for their continued assistance with the field samplings.
Disclosure statement
No potential conflict of interest was reported by the authors.
Supplemental material
Supplementary data for this article can be accessed here
References
- Almodares, A., & Hadi, M. R. (2009). Production of bioethanol from sweet sorghum: A review. African Journal of Agricultural Research, 4, 772–780.
- Bihmidine, S., Baker, R. F., Hoffner, C., & Braun, D. M. (2015). Sucrose accumulation in sweet sorghum stems occurs by apoplasmic phloem unloading and does not involve differential sucrose transporter expression. BMC Plant Biology, 15, 186–186.
- Bihmidine, S., Julius, B. T., Dweikat, I., & Braun, D. M. (2016). Tonoplast sugar transporters (SbTSTs) putatively control sucrose accumulation in sweet sorghum stems. Plant Signaling and Behavior, 11, 1–7.
- Botha, F. C., & Black, K. G. (2000). Sucrose phosphate synthase and sucrose synthase activity during maturation of internodal tissue in sugarcane. Australian Journal of Plant Physiology, 27, 81–85.
- Channappagoudar, B. B., Biradar, N. R., Patil, J. B., & Hiremath, S. M. (2007). Study on morpho-physiological, biophysical characters and alcohol production in sweet sorghum genotypes. Karnataka Journal of Agricultural Sciences, 20, 234–237.
- Etxeberria, E., Pozueta-Romero, J., & Gonzalez, P. (2012). In and out of the plant storage vacuole. Plant Science, 190, 52–61.
- Fujii, A., Nakamura, S., Nabeya, K., Nakajima, T., & Goto, Y. (2016). Relation between seeding times and stem yield of sorghum in cold region in Japan. Plant Production Science, 19, 73–80.
- Ganesh, K. C., Fatima, A., Srinivasa, R. P., Reddy, B. V. S., Rathore, A., Nageswar, R. R., … Kamal, A. (2010). Characterization of improved sweet sorghum genotypes for biochemical parameters, sugar yield and its attributes at different phenological stages. Sugar Tech, 12, 322–328.
- Grof, C. P. L., Albertson, P. L., Bursle, J., Perroux, J. M., Bonnett, G. D., & Manners, J. M. (2007). Sucrose-phosphate synthase, a biochemical marker of high sucrose accumulation in sugarcane. Crop Science, 47, 1530–1539.
- Gutjahr, S., Clément-Vidal, A., Soutiras, A., Sonderegger, N., Braconnier, S., Dingkuhn, M., & Luquet, D. (2013). Grain, sugar and biomass accumulation in photoperiod-sensitive sorghums. II. Biochemical processes at internode level and interaction with phenology. Functional Plant Biology, 40, 355–368.
- Leigh, R. A. (1984). The role of the vacuole in the accumulation and mobilization of sucrose. Plant Growth Regulation, 2, 339–346.
- Liu, Y., Nie, Y. D., Han, F. X., Zhao, X. N., Dun, B. Q., Lu, M., & Li, G. Y. (2014). Allelic variation of a soluble acid invertase gene (SAI-1) and development of a functional marker in sweet sorghum [Sorghum bicolor (L.) Moench]. Molecular Breeding, 33, 721–730.
- Mace, E. S., Tai, S., Gilding, E. K., Li, Y., Prentis, P. J., Bian, L., …., & Wang, J. (2013). Whole-genome sequencing reveals untapped genetic potential in Africa’s indigenous cereal crop sorghum. Nature Communications, 4, 2320.
- Mathur, S., Umakanth, A. V., Tonapi, V. A., Sharma, R., & Sharma, M. K. (2017). Sweet sorghum as biofuel feedstock: Recent advances and available resources. Biotechnology for Biofuels, 10, 146–146.
- McBee, G. G., & Miller, F. R. (1982). Carbohydrates in sorghum culms as influenced by cultivars, spacing, and maturity over a diurnal period. Crop Science, 22, 381–385.
- McKinley, B., Rooney, W., Wilkerson, C., & Mullet, J. (2016). Dynamics of biomass partitioning, stem gene expression, cell wall biosynthesis, and sucrose accumulation during development of Sorghum bicolor. The Plant Journal, 88, 662–680.
- Milne, R. J., Byrt, C. S., Patrick, J. W., & Grof, C. P. L. (2013). Are sucrose transporter expression profiles linked with patterns of biomass partitioning in Sorghum phenotypes? Frontiers in Plant Science, 4, 223.
- Mizuno, H., Kasuga, S., & Kawahigashi, H. (2016). The sorghum SWEET gene family: Stem sucrose accumulation as revealed through transcriptome profiling. Biotechnology for Biofuels, 9, 127–127.
- Mocoeur, A., Zhang, Y. M., Liu, Z. Q., Shen, X., Zhang, L. M., Rasmussen, S. K., & Jing, H. C. (2015). Stability and genetic control of morphological, biomass and biofuel traits under temperate maritime and continental conditions in sweet sorghum (Sorghum bicolor). Theoretical and Applied Genetics, 128, 1685–1701.
- Murray, S. C., Sharma, A., Rooney, W. L., Klein, P. E., Mullet, J. E., Mitchell, S. E., & Kresovich, S. (2008). Genetic improvement of sorghum as a biofuel feedstock: I. QTL for stem sugar and grain non-structural carbohydrates. Crop Science, 48, 2165–2179.
- Nakamura, S., Nakajima, N., Nitta, Y., & Goto, Y. (2011). Analysis of successive internode growth in sweet sorghum using leaf number as a plant age indicator. Plant Production Science, 14, 299–306.
- Okamura, M., Hirose, T., Hashida, Y., Yamagishi, T., Starch Ohsugi, R., & Aoki, N. (2013). Reduction in rice stems due to a lack of OsAGPL1 or OsAPL3 decreases grain yield under low irradiance during ripening and modifies plant architecture. Functional Plant Biology, 40, 1137–1146.
- Palaniswamy, H., Syamaladevi, D. P., Mohan, C., Philip, A., Petchiyappan, A., & Narayanan, S. (2016). Vacuolar targeting of r-proteins in sugarcane leads to higher levels of purifiable commercially equivalent recombinant proteins in cane juice. Plant Biotechnology Journal, 14, 791–807.
- Paterson, A. H. (2008). Genomics of sorghum. International Journal of Plant Genomics, 2008, 362451.
- Qazi, H. A., Paranjpe, S., & Bhargava, S. (2012). Stem sugar accumulation in sweet sorghum - Activity and expression of sucrose metabolizing enzymes and sucrose transporters. Journal of Plant Physiology, 169, 605–613.
- Rao, P. S., Kumar, C. G., & Reddy, B. V. S. (2013). Characterization of improved sweet Sorghum cultivars (pp. 1–15). Berlin: Springer.
- Reddy, P. S., Reddy, B. V. S., & Rao, P. S. (2014). Genotype by sowing date interaction effects on sugar yield components in sweet sorghum (Sorghum bicolor L. Moench). SABRAO Journal of Breeding and Genetics, 46(2), 241–255.
- Rutto, L. K., Xu, Y., Brandt, M., Ren, S., & Kering, M. K. (2013). Juice, ethanol, and grain yield potential of five sweet sorghum (Sorghum bicolor [L.] Moench) cultivars. Journal of Sustainable Bioenergy Systems, 3, 113–118.
- Shiringani, A. L., Frisch, M., & Friedt, W. (2010). Genetic mapping of QTLs for sugar-related traits in a RIL population of Sorghum bicolor L. Moench. Theoretical and Applied Genetics, 121, 323–336.
- Shoemaker, C. E., & Bransby, D. I. (2010). The Role of Sorghum as a Bioenergy Feedstock. In R. Braun, D. Karlen & D. Johnson (Eds.), Sustainable Alternative Fuel Feedstock Opportunities, Challenges and Roadmaps for Six U.S. Regions (pp. 149–159). Proceedings of the Sustainable Feedstocks for Advance Biofuels Workshop, Atlanta, GA.
- Shukla, S., Felderhoff, T. J., Saballos, A., & Vermerris, W. (2017). The relationship between plant height and sugar accumulation in the stems of sweet sorghum (Sorghum bicolor (L.) Moench). Field Crops Research, 203, 181–191.
- Teetor, V. H., Duclos, D. V., Wittenberg, E. T., Young, K. M., Chawhuaymak, J., Riley, M. R., & Ray, D. T. (2011). Effects of planting date on sugar and ethanol yield of sweet sorghum grown in Arizona. Industrial Crops and Products, 34, 1293–1300.
- Thurber, C. S., Ma, J. M., Higgins, R. H., & Brown, P. J. (2013). Retrospective genomic analysis of sorghum adaptation to temperate-zone grain production. Genome Biology, 14, 68–68.
- Tovignan, K., Fonceka, D., Ndoye, I., Cisse, N., & Luquet, D. (2016). The sowing date and post-flowering water status affect the sugar and grain production of photoperiodic, sweet sorghum through the regulation of sink size and leaf area dynamics. Field Crops Research, 192, 67–77.
- Yang, L., Bao-Qing, D., Xiang-Na, Z., Mei-Qi, Y., Ming, L., & Gui-Ying, L. (2013). Correlation analysis between the key enzymes activities and sugar content in sweet sorghum (Sorghum bicolor L. Moench) stems at physiological maturity stage. Australian Journal of Crop Science, 7, 84–92.
- Zhu, Y. J., Komor, E., & Moore, P. H. (1997). Sucrose accumulation in the sugarcane stem is regulated by the difference between the activities of soluble acid invertase and sucrose phosphate synthase. Plant Physiology, 115, 609–616.