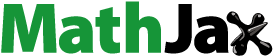
ABSTRACT
A K-shaped eccentrically braced high-strength steel frame is a novel structure. For such structures to exhibit good plastic deformation under severe earthquakes, the links are made of ordinary steel (fy≤345 MPa), whereas high-strength steel (fy≥460 MPa) is used in the frame beams and columns to reduce the cross section while ensuring the elasticity of the nonenergy consuming components. The response modification factor R is crucial to the performance-based seismic design. For an appropriate and economical seismic design, the R value and displacement amplification factor Cd should be reasonably selected. In China’s code for Seismic Design of Buildings R is uniform without considering high-strength steel, which is unreasonable. It is important to study R and Cd of K-shaped eccentrically braced high-strength steel frame. Therefore, structures with different stories (5, 10, 15, and 20 stories) and link lengths (900, 1000, 1100, and 1200 mm) were designed via the performance-based seismic design method. A static nonlinear pushover analysis and an increment dynamic analysis (IDA) were conducted. The R and Cd of each prototype were calculated using the capacity spectrum method. The effects of various factors in terms of the number of stories and link length were evaluated.
1. Introduction
K-shaped eccentrically braced frames (EBFs) made of high-strength steel are a new type of structure, in which an ordinary steel (fy ≤ 345 MPa) is utilized for the links and braces, whereas a high-strength steel (fy ≥ 460 MPa) is used for the frame beams and columns, as shown in . This type of structure not only reduces the cross-sectional size of the beam and column members, but also promotes the application of high-strength steel in seismic fortification areas. This significantly improves the performance of eccentrically braced steel frames and reduces the cost. The structure relies on the plastic shear deformation of the links to consume the seismic energy under severe earthquake loading, while the beams and columns remain in the elastic state (Li, Su, and Sui Citation2019).
Conventional design methods lead to structural over-strength, the large section size limits the engineering application of the structure, and the calculation accuracy cannot be ensured (Li, Su, and Lian et al. Citation2015). Therefore, the design concept of structural energy dissipation proposed by Japanese experts has been widely accepted (Tong and Zhao Citation2008). Based on the ductility and over-strength of structures, the energy consumption capacity of the structure in the ductile state is fully utilized to allow the structure to enter the elastoplastic range under severe earthquake loading. The response spectrum and displacement are adjusted on the basis of the response modification factor R and displacement amplification factor Cd; thus, the seismic performance of the structure under earthquake loading can be improved (Yang, Gu, and He Citation2010). Based on the analytically predicted dynamic responses of four actual building frames subjected to a set of eight historical earthquake records, some conclusions were obtained regarding the ratio of the seismic deflection amplification factor to the force reduction factor (Uang and Maarouf Citation1994). Twelve EBFs of different heights (3, 9, and 18 stories) and strengths were designed, and two types of analyses were performed: a static elastic analysis under the design base shear and a nonlinear dynamic analysis under ten design-level earthquakes. The correlation between the two types of analyses was found to be poor. Calibrated amplification factors were developed to better estimate the link inelastic rotation. Amplifying only the shear component of the elastic story deformations can help obtain more reasonable estimates (Richards and Thompson Citation2009). Based on the results of a numerical study, a new set of displacement amplification factors was established. With the proposed modifications, the EBFs were redesigned and analyzed using an inelastic time-history analysis. The proposed modifications helped improve the calculation procedures for the displacement amplification factor and link rotation angle (Kuşyılmaz and Topkaya Citation2014). The response modification factor and displacement amplification factor are vital to the design of steel and concrete structures. In seismic design, the response modification factor is typically used to reduce the structural strength under seismic fortification and to further calculate its seismic response (Yang et al., Citation2012). The displacement amplification factor is used to amplify the displacement in the elastic range so that the plastic displacement and deformation capacity of the structure can be estimated. Therefore, the response modification factor and displacement amplification factor are key considerations in the seismic design. The values of the two factors are directly related to the safety and performance of the structure during an earthquake. In recent years, some countries have proposed specifications for the response modification factor and displacement amplification factor; however, the values under these specifications are different. Because of empirical differences, the R and Cd values are not uniform. Any modification to the Cd value makes the link sections to experience a lower degree of link rotation angle during seismic events and exhibit stiffening, which increase the cumulative link rotation angle capacity, whereas any modification to the R factor can result in inadequate stiffening of the link beams (Kuşyılmaz and Topkaya Citation2016). R takes a uniform value of 2.8125 as specified in the Code for Seismic Design of Buildings (GB50011-Citation2010 Citation2010). This setting does not distinguish between specific structural forms and yields different R factors. This leads to poor reliability of specific structures and makes some structures unsafe.
The response modification factor is the ratio of the base shear in the fully elastic state under moderate earthquake to the design base shear in the inelastic state under the same seismic level. In the current seismic design code of China, the concept of response modification factor refers to reducing the elastic response spectrum of a single-degree-of-freedom (SDOF) system under moderate earthquakes (Li and Su Citation2014). Later, scholars have studied and investigated the deflection amplification of buckling-restrained braced frames (BRBFs). The results revealed that the deflection amplification is nonuniform along the building height. Inelastic drifts were found to accumulate in the lower stories, and the deflection amplification factor (Cd) exceeded the recommended value of 5.0 in the ASCE 7 standard. A Cd profile that varies along the building height was proposed, enabling cost-optimized solutions when compared with the use of a single-valued deflection amplification factor (Özkılıç, Bozkurt, and Opkaya Citation2018). The displacement amplification factor Cd is the ratio of the maximum elastoplastic displacement to the design displacement of the structure at a certain seismic level. In the seismic design of structures, the displacement amplification factor is used to estimate the maximum plastic displacement and to predict their maximum deformation capacity (Su and Li Citation2014). Researchers have conducted a significant amount of work on the response modification factor and displacement amplification factor of structures such as moment-resisting frames and concentrically braced frames (Lu and Gu Citation2008). A series of three-story, nine-story, and 20-story special concentrically braced frames (SCBFs) were designed and evaluated. The results showed significant variation between the FEMAP695 method and an alternate procedure for scaling the multiple acceleration records to the seismic design hazard. Among the buildings studied, the three-story building exhibited the highest vulnerability. To achieve a consistent margin of safety against collapse, a significantly lower R factor is required for low-rise SCBFs (three-story), whereas the current value of 6 can be continued for mid-rise and high-rise SCBFs (9-story and 20-story), as provided in ASCE-07 (Hsiao, Lehman, and Roeder Citation2013). Scholars have focused on the overall dynamic behavior of moment resisting frames (MRFs), BRBFs, and self-centering buckling-restrained braced frames under pulse-like near-fault earthquakes. To facilitate performance-based design, residual inter-story drift (RID) prediction models have been proposed, enabling a quick evaluation of the relationship between the maximum inter-story drift and the RID (Fang et al. Citation2018). In accordance with ASCE 7–05, 2–12 story buildings were designed to study the comparative residual drift response of special moment-resisting frames (SMRFs) and BRBFs. BRBFs experience greater residual drifts than SMRFs; however, the scatter in the residual drift results is significant (Erochko et al. Citation2010). A novel type of self-centering brace called variable friction self-centering energy-dissipation brace (VF-SCEDB) has been proposed, and an experimental study on a proof-of-concept VF-SCEDB specimen was conducted. The proposed VF-SCEDB exhibited both excellent energy-dissipation and self-centering capabilities. Self-centering frames have almost negligible residual inter-story drift under the maximum earthquake considered. Compared with normal SCBs and SCEDBs, the proposed VF-SCEDBs can further reduce the peak deformation and mitigate inter-story drift concentration (Chen et al. Citation2020). The overstrength, ductility, and response modification factors of low-to-mid-rise BRBFs designed as per the National Building Code of Canada 2010 have been evaluated. Four-, six-, and eight-story buildings with span lengths of 6 m and 8 m were designed and analyzed. The code prescribed value of 4.8 is somewhat a lower bound for the response modification factor of BRBFs. Additionally, the response modification factor decreases with the increase in the story height and span length. The response modification factor may be slightly affected by the bracing configuration (Moni, Moradi, and Alam Citation2016). The chapter “Seismic design requirements for building structures” in ASCE 7–16 presents the design coefficients and factors for various seismic force-resisting systems. For a particular structure, there is only one set of R and Cd values, e.g., R = 8.0 and Cd = 4.0 for steel EBFs; appropriate values of the response modification coefficient R, overstrength factor Ω0, and deflection amplification factor Cd are used to determine the base shear, element design forces, and design story drift.
The response modification factor and displacement amplification factor are not specified and are difficult to quantify. There is a lack of understanding of these factors.
The response modification factor R and displacement amplification factor Cd can be determined on the basis of the structural response curve shown in (Uang Citation1991). The horizontal coordinates in are the roof displacements of the structure, and the vertical coordinates are the base shear. OA is the performance curve of the structure in the perfectly elastic state, ODEF is the actual elastic response of the structure under earthquake loading, and ODCF is the performance curve of the structure in the ideal state. The actual nonlinear response of the structure is idealized by a bilinear elastoplastic relationship (lines ODEF are transferred to ODCF in ). The bilinear line ODCF coincides with the initial linear response of the structure, and the area enclosed by ODCF is equal to the area under the actual curve.
The response modification factor R is defined as follows:
Here, the ductility reduction factor Rμ is a parameter that measures the strength reduction due to the inelasticity effect, Rμ = Ve/Vy, where Ve is the maximum seismic demand for an elastic response, and Vy is the base shear corresponding to the maximum inelastic displacement and is also the yield force of the structure; RΩ is the over-strength factor defined as the ratio of Vy to the design base shear Vd (RΩ = Vy/Vd). The calculation formula for the displacement amplification factor Cd is:
where Δmax is the maximum displacement of the structure under moderate or severe seismic ground motion, and Δd is the design roof displacement of the structure.
In this study, the response modification factor and displacement amplification factor of a K-shaped eccentrically braced high-strength steel frame were analyzed. Our results will not only help optimize the seismic performance of this new structure and improve its safety and applicability from an economic perspective, but also help develop the design theory of existing seismic specifications. Thus, a reference is provided for the seismic design of this type of structural system.
2. Structural design and finite element modeling
2.1. Performance-based seismic design (PBSD)
The target drift and expected failure mode are primarily determined when designing a structure through the PBSD method. For EBFs, the expected failure mode is when only the links dissipate energy while the other components remain elastic when the structure is being loaded in the elastoplastic state. The story drifts along the height of the structure are well-distributed.
For EBFs, the steps involved in the PBSD method are as follows:
1) First, the fundamental period of the EBF structures is estimated, and the yield drift θy and target drift θu of the EBFs are calculated using approximate formulae. The ductility factor μs = θu/θy and ductility reduction factor Rμ are acquired using μs.
2) Second, the base shear-to-structure weight ratio V/G in the elastoplastic state is calculated using the ductility factor and other parameters, and the base shear force in the elastoplastic state is obtained.
3) Third, the story shear force of each story is obtained via the shear force distribution in the elastoplastic state. βi represents the shear distribution factor at level i, which is equal to Vi/Vn.
4) The link design is a key procedure in the PBSD method, because plastic deformations are isolated in the links and column bases. When the lateral force distribution is known, yield shear links are proportioned to create a uniform story drift along the height. By using the principle of virtual work and equating the external work to the internal work, the section of shear links can be determined.
5) Finally, the other members, such as braces, beams, and columns, are confirmed by the column free bodies using the elastic structural design program.
High-strength steel EBFs designed using the PBSD method have similar failure modes and performance objectives; hence, the seismic performance of different types of high-strength steel EBFs tend to be compared. shows the specific design process (Li, Tian, and Liu Citation2017). The different structures have similar performance objectives, bearing capacity, failure mode, and story drift distribution.
2.2. Structural design
Prototype structures were designed on the basis of the performance-based seismic design (PBSD) method. The effects of the story number (5, 10, 15, and 20 stories) and link length (900, 1000, 1100, and 1200 mm) were considered, and 16 structures were designed in total. show the plane layout and calculation unit of the model. The model number K5-0.9 indicates that the story number is 5, and the link length is 900 mm.
As shown in , the bay width is constant in both directions and is equal to 7.2 m. The story height is 3.6 m, there are five bays in the X-direction, three bays in the Y-direction, a braced bay is incorporated along the perimeter of each direction to provide the required lateral resistance, and the shadow part of a single bay frame is taken for analysis and calculation. In the model of EBFs with a high-strength steel, Q345 steel (the nominal yield strength is 345 MPa) is used for the links and braces, and Q460 steel (the nominal yield strength is 460 MPa) is utilized for the frame beams and columns. The elastic modulus is 2.06 × 105 MPa. The buildings are assumed to be located in Xi’an, China, where a peak ground acceleration of 0.3 g with a 10% probability of exceedance over a 50-year period is observed. In addition, a damping of 4% is considered appropriate for a steel building with a structural height not exceeding 50 m, and 3% for structural heights between 50 and 200 m based the requirements of (GB50011-Citation2010 Citation2010).
2.3. Finite element modeling
In this study, the SAP2000 software was used to establish a finite element analysis model. The K-5-0.9 model is taken as an example to specify the performance-based design process, as it is similar to the other models.
K-5-0.9 has a 5-story structure with a total height of 18 m and a total span of 21.6 m. shows the layout of the prototype structure, where the shadow part represents the typical computing units. The links and braces are made of ordinary steel with fy = 345 MPa, the other components are made of high strength steel with fy = 460 MPa, and the elastic modulus E is 2.06 × 105 MPa. The link length is 900 mm. Shear yielding links are adopted that use the line element simulation. The two ends of the frame column are defined as P-M hinges, and both ends of the frame beam are defined as bending hinges (M3 hinge). The brace is axially loaded, the middle part is defined as the axial force hinge (P hinge), and the two ends and central part of the links are defined as plastic shear hinges (V2 hinge).
The section of the frame column is a square steel tube, and the section of the other members is a welded H-type steel, as shown in , where “H” refers to the welded H-shaped section, with a section depth of h, flange width of bf, web thickness of tw, and flange thickness of tf (in mm). The floor dead load is 5.0 kN/m2 (including the deadweight of the floor), the live load is 2.0 kN/m2, the roof dead load is 6.0 kN/m2, the roof live load is 2.0 kN/m2, the snow load is 0.25 kN/m2, and the fundamental wind pressure is 0.35 kN/m2. The P-delta effect due to the gravity loads of buildings is considered in the SAP2000. The design member sections are listed in of the Appendix. In the tables, the length ratio of the links is ρ = eVp/Mp, and the links are shear yielding (or short link) when ρ is <1.6.
3. Experimental verification
A 1:2 scaled one-story one-bay K-shaped eccentrically braced high-strength steel frame specimen with a shear link was designed and employed to experimentally study its hysteretic and monotonic performance. The story height and span of the specimen were 1.8 and 3.6 m, respectively. The length of the shear link was 600 mm (ρ = eVp/Mp = 1.45; where e, Vp, and Mp are the link length, plastic shear capacity, and plastic moment capacity, respectively), ρ = 1.45 < 1.6, and the link was short (or shear yielding) in design.
Beams, columns, and braces were made of steel Q460 with a nominal yield strength of 460 MPa, whereas the link was made of steel Q345 with a nominal yield strength of 345 MPa. Welded joints were used to connect the links to the beams and the other members in the test specimen. presents the parameters of the member sections. The member sections are built-up sections, and H-sections are used for the members. lists the mechanical properties of the steel. The beam-column joint is a rigid connection, and the link and frame beam are butt welded. Full-depth web stiffeners are provided on both sides of the link web, and the link is provided with intermediate web stiffeners of the transverse type with a spacing of 150 mm and thickness of 10 mm.
Table 1. Member sizes of the specimen.
Table 2. Mechanical properties of steel.
A vertical load of 800 kN is applied to the top of the column by a hydraulic jack to simulate the axial force transferred to the column by the superstructure. The actuator is connected to the reaction wall at one end, and the specimen is connected at the other end to exert a horizontal load. The lateral load is transferred to the frame column on the other side through the loading beam with the hinged connection, causing a synchronous lateral displacement of the frame on the left and right columns, thereby avoiding the influence of the transmission force of the link on the horizontal load transfer (). The loading test was monotonically conducted using an actuator at a loading speed of 0.05 mm/s until structural failure. The horizontal reciprocating loading was controlled by a combination of the force and displacement in the pseudo-static test. The specimen was controlled by force before yielding and then by displacements ±Δy, ±2Δy, ±3Δy, ±4Δy, ±4.5Δy, and ±5.5Δy, where Δy is the yielding displacement. The specimens were subjected to one cycle per stage before yielding, followed by three displacement cycles until the specimen was destroyed. shows the loading protocol.
The SAP2000 finite element (FE) analysis software was used in this study to analyze the performance of the high-strength steel EBFs. An FE model was developed, and the average values of the flange and web plate properties were used as the material properties of each component. The FE models were established using the SAP2000 software (version 15.2.1). In the FE models, the plastic hinges were defined at the column-ends and beam-ends using plastic hinges for the steel columns and beams in the SAP2000 software based on the model presented in of FEMA-356 for steel columns and beams. For the shear link, the model presented in of FEMA-356 was considered for the nonlinear behavior. The ultimate shear force of the shear link Vu = 1.4Vp based on the experimental results of the shear links. Moreover, the immediate occupancy deformation ΔIO, life safety plastic deformation ΔLS, and collapse prevention deformation ΔCP of the shear link were applied using the parameters suggested by FEMA-356, as shown in
Table 3. First three periods of each prototype.
Table 4. Maximum values of the seismic influence coefficient αmax.
Table 5. Design base shear force Vd and displacement Δd for each model.
Table 6. Yield displacement Δy and yield base shear Vy for each model.
The pushover curve obtained from the pushover analyses of the FE models was compared with the test monotonic loading curves shown in . shows the hysteresis curves obtained by cyclic loading. The monotonic curve shows that the K-shaped eccentric brace with a high-strength steel frame exhibits excellent ductility and plastic deformation. The bearing capacity and stiffness of the specimens decrease with increasing plastic deformation of the link. The ultimate bearing capacity of the specimen is approximately 825 kN, and the corresponding ultimate story drift is 3.33%, which exceeds the elastoplastic story drift limit, i.e., 2%. The structure has excellent deformation capacity and ductility, the hysteresis loop is complete and stable, and the EBFs have an excellent energy dissipation capacity. For the test specimen in the elastic state at the beginning of the loading, the hysteretic loop is long and narrow, the specimen only marginally dissipates energy, and the hysteretic circuit gradually opens and tends to be complete when the link in the elastoplastic state.
show the failure modes of the specimens from the monotonic and hysteretic tests. shows the failure mode of the FE model from the pushover analysis. The failure mode of the FE model is similar to that obtained from the monotonic test. When the frame drift is more significant than H/50 (H is the height of the structure), the deformation of the link reaches the plastic limit state (GB50011-Citation2010 Citation2010), the shear hinge unloads, and the pushover curves exhibit a downward trend. The components in SAP2000 are truss elements, the average values of the material properties of the flanges and web plates were taken as the material model, and the plastic deformation of the structure was concentrated in the plastic hinges by defining the plastic behavior of the plastic hinge reaction structure. When the plastic hinge of the structure reaches the limit state in the FE model, unloading of the hinge occurs, causing the bearing capacity to rapidly decrease. The line element is used for the beams and columns in the FE model. The plastic zone is just a point, and it is different from the actual structure, which is the primary cause of errors.
4. Pushover analysis
4.1. Fundamental period of the structures
The first three periods of the structure are obtained by modal analysis, as listed in . Specifically, there is only one period for the SDOF system, while for the multi-degree-freedom system, there is the same number of mode vibrations as the number of degrees of freedom in the model.
The performance curve of the structure is obtained by a pushover analysis using SAP2000 software. The performance curves are transformed into the spectral acceleration–displacement curves (Sai–Sdi curves) of the equivalent SDOF system. Based on the quality participation coefficient αi corresponding to Ti in each vibration of the structure, the number of vibration modes participating in the combination is determined. The combination of the mass participation ratio should be >0.9, and the capacity spectrum curve corresponding to each period is obtained. This study mainly determines the capacity spectrum curve of the first three periods. The conversion formulae for the capacity spectrum method under period Ti are as follows:
Structural spectral acceleration:
Structural spectral displacement:
Mass participation ratio:
Mode participation ratio:
The representation value of the gravity load
where Xi,roof is the horizontal relative top displacement of the structure under the i-th vibration mode; Gj is the gravity load representation value of the j-story of the structures; ji is the horizontal relative displacement of the j-story under the i-th vibration mode.
4.2. Elastic-to-plastic demand spectrum
The seismic influence coefficient α is specified in the Code for Seismic Design of Buildings (GB50011-Citation2010 Citation2010) of China with the fundamental period T shown in .
The relationship between the seismic influence coefficient α and the fundamental period T can be expressed as follows:
where αmax is the maximum value of the seismic influence coefficient, η2 is the damping adjustment factor, determined by EquationEquation (12)(12)
(12) , when η2 < 0.55 and η2 = 0.55. Tg is the characteristic period, and γ is the attenuation coefficient of the descending curve calculated using EquationEquation (13)
(13)
(13) . η1 is the adjustment factor for the descent slope of the straight descent part, calculated as follows when η1 < 0 and η1 = 0.
where is the damping ratio of the structure.
presents the maximum value of the seismic influence coefficient.
In the elastic SDOF system, the parameters of the structural demand spectrum curve have the following relationship.
where Sae and Sde are the spectral acceleration and spectral displacement, respectively, k is the peak ground acceleration, β is the dynamic factor, M* is the structural equivalent mass, and K is the stiffness of the structure.
In the elastic-to-plastic SDOF system, the parameters of the structural demand spectrum curve have the following relationship.
where Sa and Sd are the spectral acceleration and spectral displacement in the elastic-to-plastic demand spectrum, respectively, μ is the ductility of the structure; Rf is the strength reduction factor, calculated as follows.
According to EquationEquations (17)(17)
(17) –(Equation20
(20)
(20) ), if the ductility factor of the structure is determined, the elastic-to-plastic demand spectrum curves will be as shown in
4.3. Response modification factor R and displacement amplification coefficient Cd
A static pushover analysis was performed using the inverted triangle lateral load distribution specified in the Chinese code (Sun and Ou Citation2008).
The R and Cd values of the studied frame are determined using the capacity spectrum method. To consider the influence of high-order vibration modes, the performance curve of the structure is transferred to the spectral acceleration–displacement curve (Sai–Sdi curve) of the equivalent SDOF system, and an inelastic demand spectrum of the structure is established. The design base shear Vd and design roof displacement Δd of the structure under frequent earthquakes are calculated, as listed in .
The elastic base shear Ve of the structure is determined on the basis of the seismic performance of the structure under moderate earthquakes. The R and Cd values of the structure can be calculated using EquationEquations (1)(1)
(1) and (Equation2
(2)
(2) ).
The capability spectrum method (CSM) is used to calculate the performance coefficient. The solutions to R and Cd based on the CSM are obtained through an iterative process, as shown in .
Taking the K5-0.9 structure as a calculation example, a pushover analysis of K5-0.9 is conducted to obtain the performance curve, and the R and Cd values of the structure are calculated using the CSM.
(1) Determining the yield point of the structure
The performance curve of the structure is transformed into a bilinear line by taking the area enclosed by the curve as unity. The inflection point of the curve is the yield point. shows the sample curve.
The area enclosed by the pushover curve is equal to that enclosed by the bilinear curve. The significant yield displacement Δy and significant yield shear Vy can be obtained from the following formulae:
where A0 is the area of the pushover curve, Δm is the maximum displacement, Vm is the maximum shear, and Ke is the initial stiffness.
The roof displacement and base shear of each model at the yield point are calculated and shown in .
From , the significant yield displacement tends to increase as the number of story increases. This is because the lateral stiffness of the structure decreases gradually with an increase in the height of the story, thereby significantly increasing the yield displacement under the same conditions.
The first-order response curve of the model K5-0.9 is plotted by connecting the values of the spectral acceleration and displacement. shows the response curve under each period.
According to the Chinese CSI (Citation2010), the standard value of structures under horizontal seismic action is calculated by the formula:
where Fek is the standard value under horizontal seismic action, α1 is the horizontal seismic influence coefficient, and Geq is the standard value of the structural gravity load.
The horizontal seismic influence coefficient α1 and related parameters can be calculated as specified in the Chinese CSI (Citation2010):
where η2 is the damping adjustment coefficient, ξ is the damping ratio, and αmax is the maximum seismic influence coefficient.
Since Δd has a linear relationship with Vd, the ratio value is the initial stiffness Ke, and Δd can be obtained as follows:
The design base shear force and design roof displacement of each model are calculated. lists the results.
(2) Reduction in the seismic demand spectrum
Based on the conditions of site category and earthquake grouping in the design of prototype structures and China’s CSI (Citation2010 version), the numerical relationship between the statistical earthquake period and the seismic influence coefficient can be determined. shows the relationship between the seismic period and the seismic influence coefficient.
4.4. Displacement demand of the structure
The calculated response spectrum curve is intersected with the reduced seismic demand spectrum under moderate and severe earthquake loadings, respectively, and the displacement demand of the structure under different ground motions is obtained by iterating the ductility demand coefficient µ1.
(1) Response curves intersect the demand spectrum curves under moderate ground motions
Taking the K5-0.9 model as an example, first, the corresponding acceleration–displacement spectrum curve is obtained under the condition that the ductility demand µ0 = 2. Second, the demand spectrum curves are intersected with the response spectrum curve of different periods, as shown in . Subsequently, the spectral displacements of these intersections are transformed into the roof displacement by applying to EquationEquation (4)(4)
(4) . The results are: Δ1 = 248.69 mm, Δ2 = −29.19 mm, and Δ3 = 10.65 mm.
A new ductility demand coefficient µ1 is obtained by taking the square root of the three roof displacements and dividing it by the significant yield displacement Δy, as follows:
The ductility coefficient (µ1) is used to calculate another demand spectrum. The new roof displacement and ductility demand coefficient (µ2) are obtained by intersecting the demand spectrum with the response spectrum. Finally, this step is repeated until the two obtained ductility demand coefficients are similar. After repeated calculations, the final iteration results are µ = 2.352 and Δ = 165.221 mm.
(2) Response spectrum curves intersect the demand spectrum curves under severe ground motions
Taking the K5-0.9 model as an example, the corresponding acceleration–displacement spectrum curve is obtained under the condition that the ductility demand µ0 = 5. The demand spectrum curve is intersected with the response spectrum curve of the first three periods, as shown in . The spectral displacements of these intersections are transformed into the roof displacement by applying to EquationEquation (4)(4)
(4) . The results are: Δ1 = 356.35 mm, Δ2 = −39.28 mm, and Δ3 = 16.45 mm.
The ductility coefficient (µ1) is used to calculate another demand spectrum. Another new roof displacement and ductility demand coefficient (µ2) are computed by intersecting the demand spectrum with the response spectrum. This step is repeated until the two obtained ductility demand coefficients are similar. Through repeated calculations, the final iteration results are µ = 5.347 and Δ = 338.867 mm.
The displacements under moderate earthquake Δe and severe earthquake Δmax of each model are listed in .
Table 7. Target displacement of each model.
The response curve shows that Ve and Δe have a linear relationship, and the proportion coefficient is the initial stiffness Ke. Therefore, the shear force Ve in elasticity can be calculated.
presents the base shear force of each model.
Table 8. Base shear force Ve of each model.
Finally, the response modification factor R and displacement amplification factor Cd of K5-0.9 are calculated.
The response modification factor R and displacement amplification factor Cd of the other models are shown in .
Table 9. Response modification factor R and displacement amplification factor Cd of each model.
From , the minimum and maximum values of the response modification factor R calculated using the pushover method are 6.392 and 11.567, respectively. The minimum and maximum values of the displacement amplification factor Cd calculated using the pushover method are 11.620 and 16.258, respectively. The two factors are significantly higher than 0.25 and 2.8125 specified in China’s CSI (Citation2010 Edition).
4.5. Effect of number of stories and length of link
In the simulation, the number of stories and link length are used as the parameters. The influence of the two parameters on the two factors is explained below.
(1) Response modification factor R
shows the influence of the change in the total number of stories on the R value. In addition to the 20-story, the value of the response modification factor R increases as the number of stories increases.
illustrates the influence of the link length on the R value. The response modification factor R of the 5-story and 10-story models decreases as the length of the link increases. The changing orderliness of R on the 15-and 20-story models is not evident.
(2) Displacement amplification factor Cd
shows the influence of the total number of stories on the Cd value. The number of stories has little influence on the value of the displacement amplification factor Cd.
illustrates the influence of the link length on the Cd value. With the exception of the 10-story, the displacement amplification factor Cd of the other stories decreases as the length of link increases, but is not distinct.
5. Calculation of response modification factor and displacement amplification factor based on increment dynamic analysis
5.1. Selection of ground motions
Nine natural ground motions with an average fluctuation of no more than 20% and ground motions in the ranges of 0.1− Tg and 0.2T1 − 1.5T1 are selected. The artificial ground motion is simulated using Seismosignal. These 10 ground motions are used for the analysis ( details each ground motion). The average ground motion curve of the 10 groups is obtained and placed in the same coordinate axis, as shown in . It is checked whether the selected ground motions meet the aforementioned requirements.
Table 10. Seismic ground motion records.
5.2. Steps of IDA
In this study, the increment dynamic analysis (IDA) of several ground motion records was adopted for each structure. The basic process is illustrated as follows:
Use SAP2000 to establish the FE model of the structures
Select appropriate ground motions according to relevant specifications
Amplify each ground motion to prepare a group of ground motions, including mild, moderate, and severe earthquakes
Implement the inelastic dynamic time-history analysis using each group of amplified ground motions
Extract the values of the base shear and roof displacement and plot the envelope curve (V–Δ) obtained from each group of ground motions in the same coordinate system. The response curve of the structure is then obtained by curve fitting.
5.3. Calculation of Factors by IDA
The specific steps in obtaining the factors via the CSM are illustrated as follows:
A nonlinear dynamic analysis is conducted under a group of different ground motions. The envelope curve of the roof displacement and bottom shear force is plotted. All the curves can be obtained via the other ground motion groups.
Fit all the obtained Δ–V envelope curves to compute the response curve of the IDA. This curve is similar to the pushover curve.
The yield shear force Vy and yield displacement Δy are determined by making the fitted IDA response curve bilinear.
Transform the fitted IDA response curve into spectrum acceleration–displacement curve (Sai–Sdi)
Plot the response curve obtained in step 4 and the elastic-to-plastic demand spectrum of the different ground motions in the same coordinate system, and determine the capacity point of the different ground motions through iteration.
Use EquationEquations (1)
(1)
(1) and (Equation2
(2)
(2) ) to calculate the response modification factor R and displacement amplification factor Cd of the structure.
The steps are shown in the .
5.4. Response curve of IDA
The base shear–displacement curve of K5-0.9 is obtained by fitting all the bottom shear and displacement under different ground motions, as shown in . The base shear–displacement curve obtained by the IDA is similar to that obtained by the pushover analysis. The curve is transformed into a bilinear curve to obtain a significant yield point. The fitted curves of the other structural models are calculated by analogy ().
Table 11. Significant yield displacement Δy and significant yield shear force Vy for each model.
5.5. Calculation of response modification factor and displacement amplification factor
First, each ground motion is dispersed into a series of ground motion data with different intensities using the amplified coefficient. The base shear–displacement curve can be obtained using the dynamic time-history analysis with the ground motions. The curve is then transformed into the response spectrum curve of the IDA by applying to the CSM and intersected with the demand spectrum under moderate and severe earthquakes. The maximum displacement Δmax under severe earthquake and moderate earthquake Δe are calculated considering a high-order vibration mode. The results are summarized in .
Table 12. Target displacement of each prototype.
Finally, the R and Cd values of each model are calculated using the method described in the previous section. lists the ultimate results.
Table 13. Response modification factor R and displacement amplification factor Cd of each model.
The minimum and maximum values of R calculated using the IDA are 3.435 and 10.319, respectively. The minimum and maximum values of the displacement amplification factor Cd are 8.506 and 13.981, respectively.
5.6. Effects of story height and link length
The story height and link length are used as the changed parameters to demonstrate their influence on the factors.
(1) Response modification factor R
shows the influence of the total number of stories on the R value. In addition to the 20-story model, the structural response modification factor R increases as the number of stories increase.
illustrates the influence of the link length on the R value. R decreases gradually as the link length increases.
(2) Displacement amplification factor Cd
shows the influence of the total number of stories on the Cd value. The number of stories has little effect on the value of the displacement amplification factor Cd.
illustrates the influence of the link length on the Cd value. The displacement amplification factor Cd for the 5-story and 20-story models decreases as the link length increases. However, the changing orderliness of Cd for the 10-story and 15-story models is not evident.
6. Recommended values of R and Cd
A pushover analysis is a static elastoplastic analysis method. A certain lateral force distribution model is used to simulate horizontal earthquake action. It is an approximate calculation method that can help simulate the response of structures under earthquake motion. However, the IDA is used to input seismic ground motions to the structure. The response of a structure is real under earthquakes, and the IDA approach has its drawbacks in that the results are relatively discrete. The R and Cd values are influenced by the IDA curves and fitting data. Therefore, this study adopts two methods to analyze the response modification factor.
The results obtained by the pushover analysis and IDA are compared in terms of the story height and link length. The response modification factor and displacement amplification factor are summarized in , respectively.
Table 14. Summary of response modification factor R of each model.
Table 15. Summary of displacement amplification factor Cd of each model.
Clearly, the values of the response modification factor R and displacement amplification factor Cd calculated by the pushover method are higher than those computed using the IDA, and the maximum difference in the response modification factor is 25.9%. However, the statistical regularity of the displacement amplification factor is unclear. This is because in the calculation and simulation, the dispersion in the displacement amplification factor calculated by the IDA is significant. The maximum difference in the displacement amplification factor Cd is 38.5%.
The values of the response modification factor and displacement amplification factor are averaged and then associated with the structural ductility coefficient µs and the structural period T, respectively. show the R–µs, R–T, and Cd–T curves by fitting.
shows the fitted curve between R and ductility coefficient µs. From the curve, the relationship can be defined as R = −3.286 µs+18.568.
shows the fitted curves between R and period T for the 5-, 10-, and 15-story structures. From the curves, the relationship R = 4.275T1 + 4.127 can be obtained. However, there is no evident relationship between R and period T for the 20-story structure. The recommended value is R = 7.194.
shows the fitted curves of Cd for the 10-, 15-, and 20-story structures. The relationship can be defined as Cd = −2.525T1 + 18.645. However, there is no evident relationship between Cd and period T for the 5-story structure. The recommended value is Cd = 12.33.
The calculation formula for the response modification factor R associated with the structural period T is applicable to 5–15 story structures, not to the 20-story one. This is because the deformation in the 20-story structure is different from that in the other structures. The deformation response of high-rise buildings is dominated by bending deformation underground motions, whereas mid- and low-rise buildings are dominated by shear deformation. In the simulation, it is assumed that all the models exhibit shear deformation.
On the other hand, the calculation formula for Cd associated with the structural period T is suitable for 10–20 story structures, not for the 20-story structure. This is because the data of the 5-story model are discrete in the simulation calculation; therefore, the relationship is not evident. However, the statistical regularity between the average values of the response modification factor R and ductility demand µ is evident, which follows a linear distribution, whereas the statistical regularity between the displacement amplification factor Cd and ductility demand is unclear. This is because in the simulation, the dispersion in the displacement amplification factor calculated by the IDA is strong, and the maximum difference in the displacement amplification factor Cd is 38.5%.
The response modification factor R and displacement amplification factor Cd are summarized. show the regularity between the total number of stories and structural factors.
compares all the R values calculated by the pushover analysis. The values of the 5-, 10-, 15-, and 20-story models range from 6.392 to 7.054, 8.033 to 10.285, 11.015 to 11.567, and 8.511 to 10.457, respectively. Furthermore, when the number of stories is the same, the value of R has little difference, and the overall fluctuation is not evident.
shows all the Cd values calculated by the IDA. It can be concluded that the values of the 5-, 10-, 15-, and 20-story models range from 9.219 to 12.364, 11.340 to 13.981, 10.962 to 13.753, and 8.506 to 12.578, respectively. The values of Cd in the same story do not fluctuate much. Moreover, the displacement amplification coefficient Cd tends to decrease as the length of the link increases, and there is no significant relationship between Cd and the number of stories. The overall fluctuation is not remarkable.
7. Conclusions
This study compared the calculation results of the pushover analysis and IDA and demonstrated the changing regularity between the factors and the number of stories and link length. Relevant R and Cd formulae in terms of the ductility demand and structural period were obtained. Finally, appropriate values of R and Cd were recommended. The results of the study can be summarized as follows:
(1) The response modification factor R and displacement amplification factor Cd of K-shaped eccentrically braced high-strength steel frame are closely related to the story height and link length. Based on the pushover analysis and IDA, the response modification factor R increases with an increase in the total number of stories, except for the 20-story structure.
(2) The response modification factor R is 2.8125 greater than the value specified in the seismic code of China. This demonstrates that the code does not make full use of the structural ductility, which is not economical and reasonable for seismic design.
(3) Through the analysis, the average R value obtained using the two analysis methods is taken as 7.194 for the 20-story structure. The relationship between the response modification factor R and the first period T1 of structures with less than 20 stories is linear, R = 4.275T1 + 4.1274. The relationship between the response modification factor R and ductility coefficient µs can be defined as R = −3.286 µs+18.568.
(4) There is a linear relationship between the displacement amplification factor Cd and the first period T1 of structures with 5 stories or more, and it can be defined as Cd = −2.525T1 + 18.645. While there is no evident relationship between the displacement amplification factor Cd and the period T1 for the 5-story structure. The recommended value can be taken as Cd = 12.331.
Disclosure statement
No potential conflict of interest was reported by the authors.
Additional information
Funding
References
- ASCE7–16 American National Standards. 2016. Minimum Design Loads and Associated Criteria for Buildings and Other Structures. Reston, Virginia: American Society of Civil Engineers.
- Chen, J. B., C. Fang, W. Wang, and Y. Q. Liu. 2020. “Variable-friction Self-centering Energy-dissipation Braces (Vf-scedbs) with NiTi SMA Cables for Seismic Resilience.” Journal of Constructional Steel Research 175 (12): 1–15. doi:10.1016/j.jcsr.2020.106318.
- CSI. 2010. SAP2000: Integrated Software for Structural Analysis & Design, Version 14.2.4-Analysis Reference Manual. Berkeley, USA: Computers and Structures.
- Erochko, J., C. Christopoulos, R. Tremblay, and H. Choi. 2010. “Residual Drift Response of SMRFs and BRB Frames in Steel Buildings Designed according to ASCE 7-05.” Journal of Structural Engineering 137 (5): 589–599. doi:10.1061/(ASCE)ST.1943-541X.0000296.
- Fang, C., Q. M. Zhong, W. Wang, S. L. Hu, and C. X. Qiu. 2018. “Peak and Residual Responses of Steel Moment-resisting and Braced Frames under Pulse-like Near-fault Earthquakes.” Engineering Structures 177 (12): 579–597. doi:10.1016/j.engstruct.2018.10.013.
- GB50011-2010. 2010. Code for Seismic Design of Buildings. in Chinese. Beijing, China: China Architecture Industry Press.
- Hsiao, P. C., D. E. Lehman, and C. W. Roeder. 2013. “Evaluation of the Response Modification Coefficient and Collapseotential of Special Concentrically Braced Frames.” Earthquake Engineering & Structural Dynamics 42 (10): 1547–1564. doi:10.1002/eqe.2286.
- Kuşyılmaz, A., and C. Topkaya. 2014. “Displacement Amplification Factors for Steel Eccentrically Braced Frames Earthquake.” Engineering and Structural Dynamics 44 (2): 167–184. doi:10.1002/eqe.2463.
- Kuşyılmaz, A., and C. Topkaya. 2016. “Evaluation of Seismic Response Factors for Eccentrically Braced Frames Using FEMA P695 Methodology.” Earthquake Spectra 32: 303–321. doi:10.1193/071014EQS097M.
- Li, S., and M. Z. Su. 2014. “Research on Seismic Design Method of Eccentrically Braced Steel Frame Based on Performance.” Engineering Mechanics 31 (10): 195–204. doi:10.3901/JME.2014.20.195.
- Li, S., M. Z. Su, M. Lian, X. W. Zheng and L.Shi 2015. “Seismic Behavior of K-shaped Eccentrically Braced Steel Frames with Multi-story High Strength Steel Composite.” Journal of Civil Engineering 2015 (10): 38–47. (in Chinese).
- Li, S., J. B. Tian, and Y. H. Liu. 2017. “Performance-based Seismic Design of Eccentrically Braced Steel Frames Using Target Drift and Failure Mode.” Earthquakes and Structures 13 (5): 443–454.
- Li, T. F., M. Z. Su, and Y. Sui. 2019. “Hybrid Test on the Seismic Behavior of High Strength Steel Composite K-eccentrically Braced Steel Frames.” Engineering Mechanics 36 (4): 101–108. doi:10.3901/JME.2000.12.101.
- Lu, Y., and Q. Gu. 2008. “The Structural Influencingfactor in Multi-story Moment Resisting Steel Frames.” Journal of University of Science and Technology of Suzhou (Engineering Technology Edition) 21 (4): 1–4+13. in Chinese.
- Moni, M., S. Moradi, and M. S. Alam. 2016. “Response Modification Factors for Steel Buckling Restrained Braced Frames Designed as per the 2010 National Building Code of Canada.” Canadian Journal of Civil Engineering 43 (8): 702–715. doi:10.1139/cjce-2014-0014.
- Özkılıç, Y. O., M. B. Bozkurt, and C. Opkaya. 2018. “Evaluation of Seismic Response Factors for BRBFs Using FEMA P695 Methodology.” Journal of Constructional Steel Research 151: 41–57. doi:10.1016/j.jcsr.2018.09.015.
- Richards, P. W., and B. Thompson. 2009. “Estimating Inelastic Drifts and Link Rotation Demands in EBFs.” AISC Engineering Journal 46 123–135. Third Quarter.
- Su, M. Z., and Y. L. Li. 2014. “Structural Influence Factor of Y-type Eccentrically Braced Frame with High Strength Steel Composite Based on IDA Method.” Journal of Xi’an University of Architectural Science and Technology (Natural Science Edition) 46 (5): 635–642. (in Chinese).
- Sun, A., and J. P. Ou. 2008. “Lateral Action Patterns and Their Effects on Pushover Seismic Analysis of Steel Tall Buildings.” Journal of Earthquake Engineering and Engineering Vibration 28 (4): 88–93. (in Chinese).
- Tong, G. S., and Y. F. Zhao. 2008. “Composit Ion of Structural Behavior Factor in Codes of China, Japan, Europe and USA for Earthquake-Resistance and Their Corresponding Ductility Demands.” Progress In Steel Building Structures 10 (5): 53–62. (in Chinese).
- Uang, C. M. 1991. “Establishing R (Or Rw) and Cd Factors for Building Seismic Provisions.” Journal of Structural Engineering (ASCE) 117 (1): 19–28. doi:10.1061/(ASCE)0733-9445(1991)117:1(19).
- Uang, C. M., and A. Maarouf. 1994. “Deflection Amplification Factor for Seismic Design Provisions.” Journal of Structural Engineering 120 (8): 2423–2436. doi:10.1061/(ASCE)0733-9445(1994)120:8(2423).
- Yang, J. F., Q. Gu, and T. He. 2010. “Structural Influence Factor and Displacement Amplification Factor (I) - Method for Determining Herringbone Center Braced Steel Frames by Incremental Dynamic Analysis Method.” Seismic Engineering and Engineering Vibration 30 (2): 64–71. (in Chinese).
- Yang, W. X., Q. Gu, Z. S. Song, D. Li, and Y. Z. Fang. 2012. “Response Modification Factor R and Overstrength Factor Ω of Y-eccentric Braced Steel Frame.” Engineering Mechanics 29 (10): 129–136. (in Chinese).
Appendix
Table A1. K5-0.9 sections.
Table A2. K5-1.0 sections.
Table A3. K5-1.1 sections.
Table A4. K5-1.2 sections.
Table A5. K10-0.9 sections.
Table A6. K10-1.0 sections.
Table A7. K10-1.1 sections.
Table A8. K10-1.2 sections.
Table A9. K15-0.9 sections.
Table A10. K15-1.0 sections.
Table A11. K15-1.1 sections.
Table A12. K15-1.2 sections.
Table A13. K20-0.9 sections.
Table A14. K20-1.0 sections.
Table A15. K20-1.1 sections.
Table A16. K20-1.2 sections.