Abstract
In most teleost fishes, the optic nerves decussate completely as they project to the mesencephalic region. Examination of the decussation pattern of 25 species from 11 different orders in Pisces revealed that each species shows a specific chiasmic type. In 11 species out of the 25, laterality of the chiasmic pattern was not determined; in half of the individuals examined, the left optic nerve ran dorsally to the right optic nerve, while in the other half, the right optic nerve was dorsal. In eight other species the optic nerves from both eyes branched into several bundles at the chiasmic point, and intercalated to form a complicated decussation pattern. In the present study we report our findings that Spratelloides gracilis, of the order Clupeiformes, family Clupeidae, shows a particular laterality of decussation: the left optic nerve ran dorsally to the right (n=200/202). In contrast, Etrumeus teres, of the same order and family, had a strong preference of the opposite (complementary) chiasmic pattern to that of S. gracilis (n=59/59), revealing that these two species display opposite left–right optic chiasm patterning. As far as we investigated, other species of Clupeiformes have not shown left–right preference in the decussation pattern. We conclude that the opposite laterality of the optic chiasms of these two closely related species, S. gracilis and E. teres, enables investigation of species-specific laterality in fishes of symmetric shapes.
In 1682, Isaac Newton predicted that the optic nerves might cross each other at the chiasmic point (Jeffery, Citation2001; Sweeney, Citation1984). Then an anatomical chart of a human being, describing the existence of the optic chiasm, was published for the first time by John Taylor (Taylor, Citation1750). In Taylor's anatomical chart some optic nerve fibres crossed at the chiasmic point and projected further to the contralateral side, while the remainder projected ipsilaterally without crossing. Today we know that this observation by Taylor was highly accurate. At present it is known that half of the optic nerves from each side cross at the chiasmic point and project to the contralateral side, while the remainder project ipsilaterally, in mammals including humans (Fisher, Citation1986; Gray, Citation1918), monkeys (Fukuda, Sawai, Watanabe, Wakakuwa, & Morigiwa, Citation1989), cats (Cooper & Pettigrew, Citation1979), rabbits (Provis & Watson, Citation1981), and rodents (Baker & Jeffery, Citation1989; Colello & Guillery, Citation1990). In conditional knockout mice lacking Exostoses 1, which is indispensable for the synthesis of heparan sulphate, it is reported that after the optic nerves pass through the optic chiasm, most fibres are misrouted into the opposite eye; that is, projection towards the brain is inhibited (Inatani, Irie, Plump, Tessier-Lavigne, & Yamaguchi, Citation2003).
While avian adult optic nerves show total decussation (Drenhaus & Rager, Citation1992), a subset of fibres project ipsilaterally in the chick embryo during development (O'Leary, Gerfen, & Cowan, Citation1983). After an intraocular injection of monensin, which specifically inhibits membrane glycoprotein transport within the axon, axons from the injected eye are misrouted towards the opposite eye in chickens (Nakayama & Furuya, Citation1989). In Xenopus laevis tadpoles show total decussation of optic nerves, while adults show semi-decussation after metamorphosis (Hoskins, Citation1986; Hoskins & Grobstein, Citation1984; Nakagawa et al., Citation2000). Similarly, lamprey larvae show total decussation of the optic nerves, while adult lampreys show semi-decussation (Kennedy & Rubinson, Citation1977; Kosareva, Citation1980).
In fish, the decussation pattern at the optic chiasm has been studied in Fundulus embryos (Oppenheimer, Citation1950), goldfish (Fernald, Citation1982; Roth, Citation1979), and electric gymnotid fish (Apteronotus leptorhynchus; Sas & Maler, Citation1986). In these species the optic nerves cross at the chiasmic point and show complete decussation. After formation of the optic chiasm, the optic nerves project to the mesencephalic region. Left–right asymmetry of the decussation pattern can occur in the optic chiasm of fishes, in that the optic nerve from one side can pass dorsally or ventrally to the nerve from the other side. While most lower vertebrates show total decussation of their optic nerves, many higher vertebrates show semi-decussation, an important difference when we consider the development and evolution of the brain and nervous system.
It has been reported that flatfish and flounder, which have remarkably asymmetric bodies, show left–right asymmetry of the optic chiasm (Parker, Citation1903; Policansky, Citation1982). The frontal region of flatfish develops to be sinistral or dextral during metamorphosis, and shows remarkable asymmetry. The eyes also migrate to one side of the body concomitantly with the turning of the frontal region. This morphological asymmetry affects several functional asymmetries of the head and jaws during prey capture (Gibb, Citation1996). When flatfish larvae were treated with triiodothyronine prior to metamorphosis, the frontal bones were formed symmetrically, eye migration was inhibited, and the treated flatfishes developed symmetrically (Schreiber, Citation2006). This report proposes that the difference of the sensitivity for thyroid hormone between the left and right sides may determine the left–right asymmetry of the rotation of the frontal bone during metamorphosis. The mutant Japanese flounder (Paralichthys olivaceus) “reversed (rev)” displays facial left–right reversal due to randomised embryonic pitx2 expression, which is normally left-handed (Hashimoto et al., Citation2002). The causative gene responsible for the rev locus has not yet been identified. It remains controversial whether flounder metamorphic asymmetry is determined by an unidentified genetic cascade in a manner independent of visceral asymmetry control.
The above molecular mechanism identified in the flatfishes may affect their chiasmic pattern. However, comparative studies of the chiasmic patterns in various fishes have been somewhat neglected, and here we query whether the laterality of the optic chiasm is predictable in fish with symmetric bodies, as it is in flatfish. Using a morphological approach we investigated the laterality of the optic chiasm in a number of freshwater and seawater fish, in order to discover an appropriate model animal for the study of left-right asymmetry in the optic chiasm.
METHOD AND MATERIALS
Patterns of optic chiasms of the adults were investigated in 25 species of 11 orders of freshwater and seawater fish ( & ). Dissections of the fish were performed in petri dishes filled with saline. After decapitation, aided by a stereoscopic microscope, the lower jaw and some additional tissue were removed to expose the optic chiasm. The optic chiasm was digitally photographed from the ventral side, and each was classified into one of the three types shown in b.
Figure 1. Photographs of the fish used in studies of the decussation pattern of the optic chiasm. Bars, 1 cm or 10 cm.
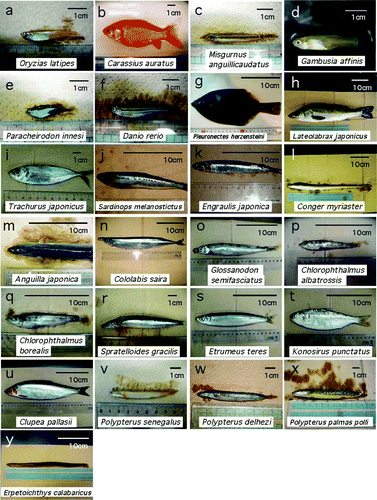
Figure 2. Taxonomic classification of the fishes used and definitions of the chiasmic patterns identified in this study. (a) List of the species examined in this report. Optic chiasms were investigated in 25 species of 11 orders of freshwater and seawater fishes. (b) A type 1 chiasm is defined as a complete decussation in which the entire left optic nerve runs dorsally to the entire right optic nerve. A type 2 chiasm is that in which the entire right optic nerve runs dorsally to the entire left optic nerve (complementary to type 1 in its laterality). A type 3 chiasm is defined as that in which the optic nerves from both sides cross in a complex manner consisting of intercalated bundles. All the chiasms shown are viewed from the ventral side in – except for .
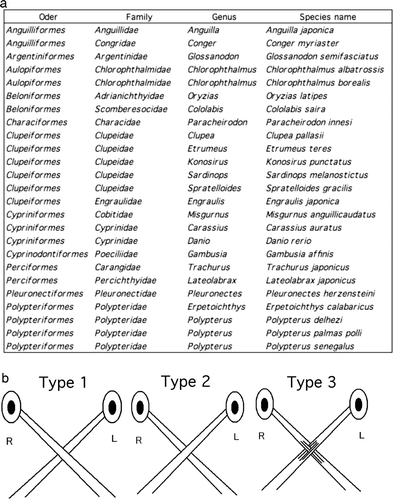
Statistical analysis using a 2×2 contingency table (Fisher's direct probability test) was performed to examine whether there was a bias in the incidence of the type 1 chiasm compared to that of type 2.
RESULTS
The optic nerves crossed to form an optic chiasm in all individuals examined (n=988 adults), across 25 species. Decussation was complete in all species except the Polypteriformes. In each individual of the species showing total decussation, the pattern of decussation was classified into one of three types: type 1, in which the entire left optic nerve passed dorsally to the right optic nerve; type 2, in which the entire right optic nerve passed dorsally to the left; or type 3, in which each optic nerve branched into several bundles and intercalated at the optic chiasm.
Among the 25 species examined, 11 species (Oryzias latipes, Carassius auratus, Misgurnus anguillicaudatus, Gambusia affinis, Lateolabrax japonicus, Trachurus japonicus, Sardinops melanostictus, Anguilla japonica, Conger myriaster, Cololabis saira, and Konosirus punctatus) showed a type 1 or type 2 chiasmic pattern, with an apparently random, 40–60% occurrence of each pattern within each species ( & ; ). Type 3 decussation was not observed in any of the species showing type 1 or 2 decussation. Statistical analyses using 2×2 contingency tables proved that, in these fishes, the choice between type 1 and type 2 chiasms had no significant bias at the 5% significance level (all the χ-values were below 3.24; p>.05).
Figure 3. Fish showing both type 1 and type 2 chiasmic patterns in equal frequencies within one species. Scientific names of the fishes are indicated in the photographs. Of the 25 species examined, 11 species showed a random preference for a type 1 or type 2 chiasm within individuals (see also ). Left column, type 1; right column, type 2.
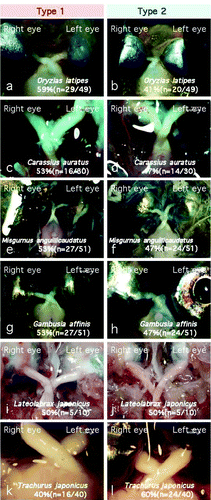
Figure 4. These species also displayed a random occurrence of a type 1 or type 2 chiasm (see also ).
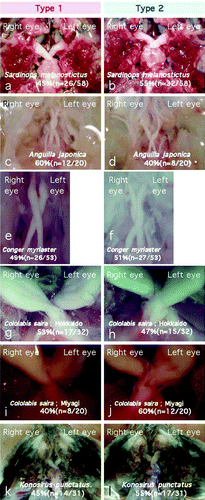
TABLE 1. Patterns of the optic chiasm of the fish investigated in this report
Seven species (Danio rerio, Paracheirodon innesi, Engraulis japonica, Glossanodon semifasciatus, Chlorophthalmus albatrossis, Chlorophthalmus borealis, and Clupea pallasii) showed a type 3 decussation pattern. All of these species except D. rerio (zebrafish) showed type 3 decussation only, and no individual showed type 1 or 2 decussation (, see ). However, in zebrafish 12% of the specimens showed a type 1 or 2 decussation pattern, and the remaining 88% showed a type 3 pattern ().
Figure 5. Of the 25 species examined, 7 species showed a type 3 chiasm. In these species, almost all individuals showed no laterality within the optic chiasm and the optic nerve fibres crossed in a complex manner.
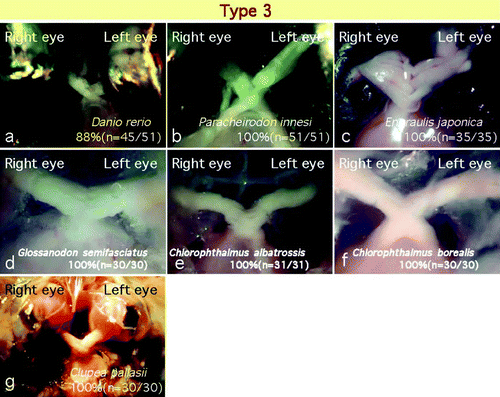
Spratelloides gracilis from the family Clupeidae, order Clupeiformes, showed only a type 1 decussation pattern; in almost all specimens the left optic nerve ran dorsally to the right optic nerve (n=200/202, a, see ). Regardless of the fishing ground (Kochi or Kagoshima, Japan), an absolute preference for type 1 decussation was conserved in S. gracilis. In contrast, Etrumeus teres, belonging to the same family of the same order as S. gracilis, showed an absolute preference for type 2 decussation (n=59/59, b, see ). As with S. gracilis, there was no apparent effect of the different fishing grounds (Kagoshima or Shimane, Japan) on the pattern of decussation in E. teres.
Figure 6. The optic chiasm of Spratelloides gracilis showed opposite laterality to that of Etrumeus teres, although these species belong to the same order Clupeiformes and the same family Clupeidae. (a) Optic chiasm of S. gracilis; left optic nerve invariably ran dorsally to the right optic nerve at the decussation. (b) Optic chiasm of E. teres; right optic nerve always ran dorsally to the left optic nerve.
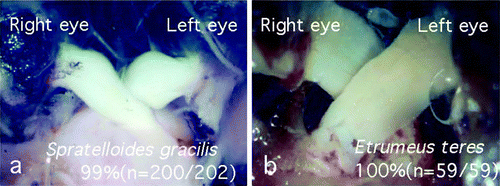
The chiasmic pattern of decussation was highly varied across the order Clupeiformes. While C. pallasii and E. japonica showed type 3 chiasms (see ), S. melanostictus and K. punctatus showed both type 1 and type 2 chiasms at an equal frequency (see ). Although these species belong to the same family of the same order as S. gracilis and E. teres, the patterns of the optic chiasms varied among the species. While all of the species within this order have a symmetric body, only S. gracilis and E. teres (both of the subfamily Dussumieriinae) showed a particular laterality of the optic chiasm. These results suggest that a unique phenomenon occurred during the evolution of these fish, before or after the speciation of S. gracilis and E. teres.
The decussation pattern of the optic chiasm in flatfish, which have an asymmetric external morphology, has been studied using Heterosomata over a lengthy period (Parker, Citation1903; Policansky, Citation1982). Examination of the optic chiasm of Pleuronectes herzensteini (Japanese flounder), which has a remarkably asymmetric external morphology, revealed that the optic nerve from the left eye always runs dorsally to the right optic nerve (Tamura, Citation2005 ,n=10/10; ).
Figure 7. Decussation pattern within the optic chiasm of Pleuronectes herzensteini (Japanese flounder), which has a remarkably asymmetrical body. At the chiasm, the left optic nerve always ran dorsally to the right; all specimens showed definite laterality of the chiasmic pattern (n=10/10). This figure is viewed from the dorsal side.
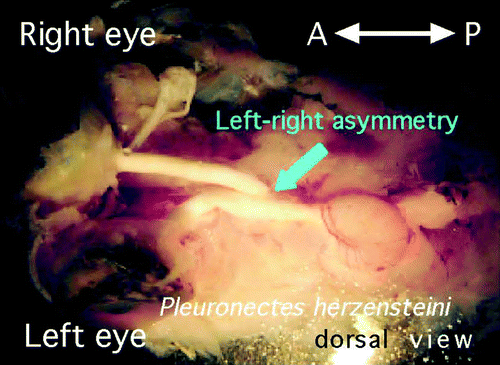
Polypterus senegalus, of the order Polypteriformes, family Polypteridae, is an ancient fish belonging to Brachiopterygii. The optic chiasm of P. senegalus resembled a type 3 decussation pattern, but the optic nerves from both eyes branched into numerous bundles at the chiasmic point, and showed a fused decussation pattern (n=17/17; , see ). Polypterus delhezi (n=3/3) and Polypterus palmas polli (n=5/5) in the same family of the same order also showed a “fused” chiasmic pattern. Erpetoichthys calabaricus, belonging to the order Polypteriformes, family Polypteridae, also showed a “fused” chiasm (n=7/7). All of the ancient fishes investigated showed a similar chiasmic pattern.
Figure 8. Ancestral fishes of subclass Branchiopterygii showed a “fused” type chiasm, in which no fasciculations of the optic nerves were observed at the chiasmic point.
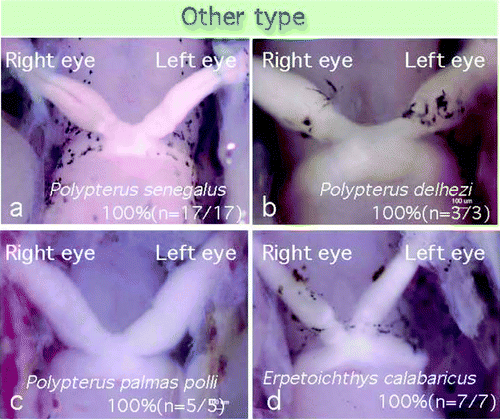
Scores for all of the fish species examined in the present study can be found in . The chiasmic patterns of the fishes are labelled in the phylogenetic tree in , in which the pattern of the optic chiasm in each species is indicated by a superscript. The evolutionarily ancient fishes showed a tendency towards a “fused” chiasmic pattern, and five species of higher orders belonging to superorder Acanthopterygii (Gambusia affinis, Oryzias latipes, Cololabis saira, Lateolabrax japonicus, and Trachurus japonicus) but not the Pleuronectiformes (flatfishes), showed both type 1 and type 2 patterns equally in each species (, see ).
Figure 9. Phylogenetic tree of the fish investigated in this report, showing the chiasmic pattern for each species. The decussation pattern in the optic chiasm is indicated by a superscript (1, 2, and 3 indicate types 1, 2, and 3 chiasm respectively; 1 & 2 indicates a mixture of types 1 and 2, occurring in equal frequency).
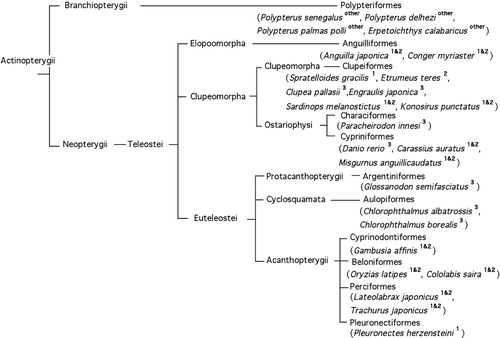
DISCUSSION
Why might the optic chiasm in most fish show complete decussation? The answer to this question may relate partly to predator avoidance: lateral positioning of the eyes provides a wider visual field. Complete decussation facilitates the propagation of optical information to the contralateral side of the body, allowing efficient activation of motor neurons controlling the body wall muscle and aiding rapid escape from predators (Sarnat & Netsky,Citation1981). In the adult specimens of the 25 species investigated here, three patterns of decussation at the optic chiasm were observed (see ,,, and ). Both types 1 and 2 consisted of a “simple chiasm” in that all of the optic nerves from one side wrapped over all of the counterpart. In contrast, type 3 consisted of a “meshed chiasm” in that the optic nerves from both eyes crossed in a more complicated manner. As such, these species appeared to have some as yet undetermined mechanism regulating the formation of the chiasmic pattern during optic nerve projection to the tectum. Our investigations of S. gracilis and E. teres lead us to expect that the left–right patterning of the optic chiasm could constitute a useful indicator of the mechanism of neural development in teleosts.
If the timings of both the crossing and fasciculation of the optic nerves were determined randomly during embryogenesis, then all three chiasmic patterns (type 1, type 2, and type 3) should appear at equal frequencies in one species, or alternatively, only type 3 should appear in every specimen. However, our results revealed that in fish species showing type 1 and/or type 2 chiasmic patterns, type 3 specimens did not occur, while in fish with a type 3 chiasm, neither type 1 nor type 2 chiasmic patterns appeared (with the exception of zebrafish). These results lead us to hypothesise that an exclusive choice between types 1/2 vs type 3 chiasms could be exercised by an unknown mechanism during optic chiasm formation. To investigate this hypothesis further, it would be useful to perform genetic screens of a medaka (Oryzias latipes, of which all of the wild type specimens showed a type 1 or 2 chiasm) mutant frequently showing a type 3 chiasm, and to identify the causative gene(s). Alternatively, it would be useful to identify a zebrafish (Danio rerio, of which most showed a type 3 chiasm) mutant showing only type 1 and 2 chiasms substituted for a type 3 chiasm.
P. senegalus, P. delhezi, P. palmas polli, and E. calabaricus, of the order Polypteriformes, family Polypteridae, are ancient fishes, and showed a type 3-like “fused” chiasm (see and ). Considering that fishes of Polypteridae are ancestral ones, “fused” decussation with no fasciculate bundles at the chiasmic point may constitute the chiasmic prototype. To make type 1 and type 2 chiasms, an additional mechanism controlling the timing and/or dorsal/ventral positioning of the optic nerve projection might be required. Polypteridae fishes do not appear to possess a mechanism for allowing fasciculation of the optic fibres into thick bundle(s) at the chiasmic point.
S. gracilis and E. teres, of the order Clupeiformes, family Clupeidae, showed a strong laterality in the optic chiasm (see and ). It is particularly interesting that S. gracilis and E. teres showed complementary laterality in the optic chiasm, even though the two species are the most closely related within the order Clupeiformes (Asanuma & Matsuoka, Citation2001; Lavoué, Miya, Saitoh, Ishiguro, & Nishida, Citation2007). While C. pallasii and E. japonica in the same family of the same order showed a type 3 chiasmic pattern (see ), S. melanostictus and K. punctatus showed type 1 and type 2 patterns (see ). Five extant species of Spratelloides are known, and three of them, S. gracilis (Temminck & Schlegel, Citation1846), S. delicatulus (Bennett,Citation1831), and S. atrofasciatus (Schultz, Citation1943), live in the warm Japanese ocean. S. gracilis specimens are widely distributed in tropical seas including coastal areas of West Japan and Australia, and E. teres are widely distributed in the warm oceans of West Japan and South China (Watanabe, Shirahuji, & Chimura, Citation2001).
The spawning seasons of S. gracilis and E. teres partially overlap. While S. gracilis spawns in the spring and early summer, when water temperatures are in the range of 23–27°C, E. teres spawns in autumn and spring, when water temperatures are in the range of 15–25°C (Watanabe et al., Citation2001). Since these two species partly share a spawning season, and both require warm water temperatures for embryogenesis, we suggest that complementary chiasmic patterns of these species are unlikely to be caused by differences in seasonal factors.
The opposite laterality of the optic chiasms of S. gracilis and E. teres will be useful for studying the establishment of the species-specific laterality of the central nervous system (CNS) in fishes of symmetric shapes. The brain lateralisation of human beings is thought to have originated from the functional asymmetries in the behaviour of lower vertebrates at both the individual and population level (for review see Bisazza, Rogers, & Vallortigara, Citation1998; Vallortigara & Rogers Citation2005). Lateral asymmetry in the direction of turning during escape behaviour in a species of poeciliid fish, Girardinus falcatus, has been reported (Cantalupo, Bisazza, & Vallortigara, Citation1995). This asymmetry appears to be due to a heritable preferential use of the right eye (Bisazza, Dadda, & Cantalupo, Citation2005; Bisazza, Facchin, & Vallortigara, Citation2000; Facchin, Bisazza, & Vallortigara, Citation1999). In contrast, zebrafish fry use the left eye when they see their own reflection in a mirror (Sovrano & Andrew, Citation2006). At the neuroanatomical level, asymmetries of the parapineal complex in lampreys, teleosts, frogs, newts, and lizards have been reported (Concha & Wilson, Citation2001; Hendricks & Jesuthasan, Citation2007). Thus, studies on the laterality of the CNS might provide a deep insight into the evolution and speciation of the teleosts. Our findings show that laterality of the optic chiasm could be one new model system for studying teleost brain lateralisation.
Perissodus microlepis lives in Lake Tanganyika and is known as the “scale eater”. These fish have asymmetric bodies, and thus selectively eat scales only on one side of their prey (Hori, Citation1993; Nakajima, Matsuda, & Hori, Citation2004; Takahashi & Hori, Citation2008). The “left-handed” scale eater has its mouth open towards the right side and attacks the left side of its prey, whereas “right-handed” individuals have their mouth open towards their left side and attack the right side of their prey. We hypothesise that a similar survival-based bias governs the laterality of the decussation pattern in S. gracilis and E. teres. Ancestral species of both S. gracilis and E. teres might have originally had both type 1 and 2 chiasmic patterns, following which one type might have been selected and the other eliminated during evolution. We anticipate that the laterality of the decussation pattern may be characterised by a small number of genes, because the two most closely related species examined (S. gracilis and E. teres) show completely opposite preferences in chiasmic pattern. From a developmental viewpoint, during the embryonic stages a subset of optic neurons might be eliminated by apoptosis to simplify the overlap of optic nerves to form type 1 or type 2 decussation. Unfortunately, until recently it has been extremely difficult to cultivate S. gracilis and E. teres and to achieve their artificial insemination, and no reports on the embryogenesis of these species in the laboratory environment are available. As such, it is expected that an embryological approach to analyse the mechanism of optic chiasm formation in S. gracilis or E. teres will be very difficult. Instead, a genomic approach to analyse chiasmic laterality might be suitable; detailed analyses of the homologues of optic chiasm-related genes and the corresponding cis-elements that are/will be identified in model fish such as zebrafish or medaka are needed. Oth erwise, techniques for the artificial breeding and fertilisation of S. gracilis and E. teres should be developed.
In zebrafish it has been reported that cyclops (cyc), chameleon (con), you-too (yot), iguana (igu), and detour (dtr) show no optic nerve decussation or show abnormal optic decussation patterning (Brand et al., Citation1996). The zebrafish belladonna (bel) mutant is defective in the axonal guidance factors, and neither the optic chiasm nor the forebrain commissural fibres are formed (Seth et al., Citation2006). Weak alleles of these genes are expected to provide useful information on the laterality of the chiasmic pattern.
Using zebrafish, the laterality of the pineal complex and habenula in the dorsal diencephalon has been studied in detail, in the context of investigations into the left–right asymmetry of the brain (for review see Guglielmotti & Cristino, 2006; Halpern et al., Citation2003). The left nucleus of the habenulae (nuclei habenulae), the paired nuclei of the dorsal diencephalon neighbouring the pineal complex, is larger than the right nucleus and has more dense neuropil (Concha, Burdine, Russell, Schier, & Wilson, Citation2000; Halpern et al., Citation2003). Neural circuits connecting the pineal complex and the habenulae show left–right asymmetry in zebrafish. Projections from the dorsal habenulae to the interpeduncular nucleus (Fasciculus retroflexus [Meynert]) also show left–right differences (Aizawa et al., Citation2005; Gamse et al., Citation2005). These asymmetries are regulated by Nodal signalling (Aizawa et al., Citation2005; Concha et al., Citation2000, Citation2003; Long, Ahmad, & Rebagliati, Citation2003), Notch signalling (Aizawa, Goto, Sato, & Okamoto, Citation2007), Wnt signalling (Carl et al., Citation2007), and by neuropilin (a receptor for class III semaphorins) (Kuan, Yu, Moens, & Halpern, Citation2007).
Cyclops, one of the zebrafish nodal-related genes, is involved in left–right specification and is temporally expressed in the left side of the dorsal diencephalon and left-lateral plate mesoderm (Bisgrove, Essner, & Yost, Citation2000; Boggetti et al., Citation2000; Hashimoto et al., Citation2004). Zebrafish southpaw, which is highly homologous to cyclops, is also expressed in the left lateral plate mesoderm but not in the dorsal diencephalon (Long et al., Citation2003). Although southpaw is not expressed in the diencephalon, it has a significant effect on the left–right asymmetry of the brain (Long et al., Citation2003). It would be interesting to investigate the relationships among visceral left–right asymmetry, cerebral laterality, and the decussation pattern in nodal-related mutants. Such an approach will likely contribute to the identification of the genes governing the laterality of the chiasmic pattern in S. gracilis and E. teres.
Acknowledgements
We were greatly helped and encouraged by the advice of Prof. Akiya Hino, Prof. Tsuyoshi Ogasawara, and Prof. Kazuhito Inoue. We thank also Prof. Yoshihiro Suzuki for providing us with the opportunity to use digital microscope.
References
- Aizawa , H. , Bianco , I. H. , Hamaoka , T. , Miyashita , T. , Uemura , O. Concha , M. L. 2005 . Laterotopic representation of left–right information onto the dorso-ventral axis of a zebrafish midbrain target nucleus . Current Biology , 15 : 238 – 243 .
- Aizawa , H. , Goto , M. , Sato , T. and Okamoto , H. 2007 . Temporally regulated asymmetric neurogenesis causes left–right difference in the zebrafish habenular structures . Developmental Cell , 12 : 87 – 98 .
- Asanuma , T. and Matsuoka , N. 2001 . Phylogenetic relationships among seven fish species of the order Clupeiformes inferred from allozyme variation. Bulletin of the Faculty of Agriculture and Life Science . Hirosaki University , 4 : 1 – 15 .
- Baker , G. E. and Jeffery , G. 1989 . Distribution of uncrossed axons along the course of the optic nerve and chiasm of rodents . Journal of Comparative Neurology , 289 : 455 – 461 .
- Bennett , E. T. 1831 . Observations on a collection of fishes from the Mauritius, with characters of new genera and species . Proceedings of the Zoological Society of London , 1 : 126 – 128 .
- Bisazza , A. , Dadda , M. and Cantalupo , C. 2005 . Further evidence for mirror-reversed laterality in lines of fish selected for leftward or rightward turning when facing a predator model . Behavioural Brain Research , 156 : 165 – 171 .
- Bisazza , A. , Facchin , L. and Vallortigara , G. 2000 . Heritability of lateralization in fish: Concordance of right–left asymmetry between parents and offspring . Neuropsychologia , 38 : 907 – 912 .
- Bisazza , A. , Rogers , L. J. and Vallortigara , G. 1998 . The origins of cerebral asymmetry: A review of evidence of behavioural and brain lateralization in fishes, reptiles and amphibians . Neuroscience Biobehavioral Reviews , 22 : 411 – 426 .
- Bisgrove , B. W. , Essner , J. J. and Yost , H. J. 2000 . Multiple pathways in the midline regulate concordant brain, heart and gut left–right asymmetry . Development , 127 : 3567 – 3579 .
- Boggetti , B. , Argenton , F. , Haffter , P. , Bianchi , M. E. , Cotelli , F. and Beltrame , M. 2000 . Cloning and expression pattern of a zebrafish homolog of forkhead activin signal transducer (FAST), a transcription factor mediating Nodal-related signals . Mechanisms of Development , 99 : 187 – 190 .
- Brand , M. , Heisenberg , C. P. , Warga , R. M. , Pelegri , F. , Karlstrom , R. O. Beuchle , D. 1996 . Mutations affecting development of the midline and general body shape during zebrafish embryogenesis . Development , 123 : 129 – 142 .
- Cantalupo , C. , Bisazza , A. and Vallortigara , G. 1995 . Lateralization of predator-evasion response in a teleost fish (Girardinus falcatus) . Neuropsychologia , 33 : 1637 – 1646 .
- Carl , M. , Bianco , I. H. , Bajoghli , B. , Aghaallaei , N. , Czerny , T. and Wilson , S. W. 2007 . Wnt/Axin1/beta-catenin signaling regulates asymmetric nodal activation, elaboration, and concordance of CNS asymmetries . Neuron , 55 : 393 – 405 .
- Colello , R. J. and Guillery , R. W. 1990 . The early development of retinal ganglion cells with uncrossed axons in the mouse: Retinal position and axonal course . Development , 108 : 515 – 523 .
- Concha , M. L. , Burdine , R. D. , Russell , C. , Schier , A. F. and Wilson , S. W. 2000 . A nodal signaling pathway regulates the laterality of neuroanatomical asymmetries in the zebrafish forebrain . Neuron , 28 : 399 – 409 .
- Concha , M. L. , Russell , C. , Regan , J. C. , Tawk , M. , Sidi , S. Gilmour , D. T. 2003 . Local tissue interactions across the dorsal midline of the forebrain establish CNS laterality . Neuron , 39 : 423 – 438 .
- Concha , M. L. and Wilson , S. W. 2001 . Asymmetry in the epithalamus of vertebrates . Journal of Anatomy , 199 : 63 – 84 .
- Cooper , M. L. and Pettigrew , J. D. 1979 . The decussation of the retinothalamic pathway in the cat, with a note on the major meridians of the cat's eye . Journal of Comparative Neurology , 187 : 285 – 311 .
- Drenhaus , U. and Rager , G. 1992 . Organization of the optic chiasm in the hatched chick . Anatomical Record , 234 : 605 – 617 .
- Facchin , L. , Bisazza , A. and Vallortigara , G. 1999 . What causes lateralization of detour behavior in fish? Evidence for asymmetries in eye use . Behavioural Brain Research , 103 : 229 – 234 .
- Fernald , R. D. 1982 . Retinal projections in the African cichlid fish, Haplochromis burtoni . Journal of Comparative Neurology , 206 : 379 – 389 .
- Fisher , N. F. 1986 . The optic chiasm and the corpus callosum: Their relationship to binocular vision in humans . Journal of Pediatric Ophthalmology & Strabismus , 23 : 126 – 131 .
- Fukuda , Y. , Sawai , H. , Watanabe , M. , Wakakuwa , K. and Morigiwa , K. 1989 . Nasotemporal overlap of crossed and uncrossed retinal ganglion cell projections in the Japanese monkey (Macaca fuscata) . Journal of Neuroscience , 9 : 2353 – 2373 .
- Gamse , J. T. , Kuan , Y. S. , Macurak , M. , Brösamle , C. , Thisse , B. Thisse , C. 2005 . Directional asymmetry of the zebrafish epithalamus guides dorsoventral innervation of the midbrain target . Development , 132 : 4869 – 4881 .
- Gibb , A. 1996 . The kinematics of prey capture in Xystreurys liolepis: Do all flatfish feed asymmetrically? . Journal of Experimental Biology , 199 ( 10 ) : 2269 – 2283 .
- Gray , H. 1918 . Anatomy of the human body , Philadelphia : Lea and Febiger .
- Guglielmotti , V. and Cristino , L. 2006 . The interplay between the pineal complex and the habenular nuclei in lower vertebrates in the context of the evolution of cerebral asymmetry . Brain Research Bulletin , 69 : 475 – 488 .
- Halpern , M. E. , Liang , J. O. and Gamse , J. T. 2003 . Leaning to the left: Laterality in the zebrafish forebrain . Trends in Neuroscience , 26 : 308 – 313 .
- Hashimoto , H. , Mizuta , A. , Okada , N. , Suzuki , T. , Tagawa , M. Tabata , K. 2002 . Isolation and characterization of a Japanese flounder clonal line, reversed, which exhibits reversal of metamorphic left–right asymmetry . Mechanisms of Development , 111 : 17 – 24 .
- Hashimoto , H. , Rebagliati , M. , Ahmad , N. , Muraoka , O. , Kurokawa , T. Hibi , M. 2004 . The Cerberus/Dan-family protein Charon is a negative regulator of Nodal signaling during left–right patterning in zebrafish . Development , 131 : 1741 – 1753 .
- Hendricks , M. and Jesuthasan , S. 2007 . Asymmetric innervation of the habenula in zebrafish . Journal of Comparative Neurology , 502 : 611 – 619 .
- Hori , M. 1993 . Frequency-dependent natural selection in the handedness of scale-eating cichlid fish . Science , 260 : 216 – 219 .
- Hoskins , S. G. 1986 . Control of the development of the ipsilateral retinothalamic projection in Xenopus laevis by thyroxine: Results and speculation . Journal of Neurobiology , 17 : 203 – 229 .
- Hoskins , S. G. and Grobstein , P. 1984 . Induction of the ipsilateral retinothalamic projection in Xenopus laevis by thyroxine . Nature , 307 : 730 – 733 .
- Inatani , M. , Irie , F. , Plump , A. S. , Tessier-Lavigne , M. and Yamaguchi , Y. 2003 . Mammalian brain morphogenesis and midline axon guidance require heparan sulfate . Science , 302 : 1044 – 1046 .
- Jeffery , G. 2001 . Architecture of the optic chiasm and the mechanisms that sculpt its development . Physiological Reviews , 81 : 1393 – 1414 .
- Kennedy , M. C. and Rubinson , K. 1977 . Retinal projections in larval, transforming and adult sea lamprey, Petromyzon marinus . Journal of Comparative Neurology , 171 : 465 – 479 .
- Kosareva , A. A. 1980 . Retinal projections in lamprey (Lampetra fluviatilis) . Journal für Hirnforschung , 21 : 243 – 256 .
- Kuan , Y. S. , Yu , H. H. , Moens , C. B. and Halpern , M. E. 2007 . Neuropilin asymmetry mediates a left–right difference in habenular connectivity . Development , 134 : 857 – 865 .
- Lavoué , S. , Miya , M. , Saitoh , K. , Ishiguro , N. B. and Nishida , M. 2007 . Phylogenetic relationships among anchovies, sardines, herrings and their relatives (Clupeiformes), inferred from whole mitogenome sequences . Molecular Phylogenetics and Evolution , 43 : 1096 – 1105 .
- Long , S. , Ahmad , N. and Rebagliati , M. 2003 . The zebrafish nodal-related gene southpaw is required for visceral and diencephalic left–right asymmetry . Development , 130 : 2303 – 2316 .
- Nakagawa , S. , Brennan , C. , Johnson , K. G. , Shewan , D. , Harris , W. A. and Holt , C. E. 2000 . Ephrin-B regulates the ipsilateral routing of retinal axons at the optic chiasm . Neuron , 25 : 599 – 610 .
- Nakajima , M. , Matsuda , H. and Hori , M. 2004 . Persistence and fluctuation of lateral dimorphism in fishes . The American Naturalist , 163 : 692 – 698 .
- Nakayama , T. and Furuya , S. 1989 . Monensin induces distortion of optic nerve crossing at the chick embryo chiasm . Experimental Brain Research , 76 : 559 – 562 .
- O'Leary , D. M. , Gerfen , C. R. and Cowan , W. M. 1983 . The development and restriction of the ipsilateral retinofugal projection in the chick . Brain Research , 312 : 93 – 109 .
- Oppenheimer , J. M. 1950 . Anomalous optic chiasma in Fundulus embryos . Anatomical Record , 108 : 477 – 483 .
- Parker , G. H. 1903 . The optic chiasma in teleosts and its bearing on the asymmetry of the heterosomata (flatfishes) . Bulletin of the Museum of Comparative Zoology , 40 : 221 – 242 .
- Policansky , D. 1982 . Flatfishes and the inheritance of asymmetries . Behavioral and Brain Sciences , 5 : 262 – 266 .
- Provis , J. M. and Watson , C. R. 1981 . The distribution of ipsilaterally and contralaterally projecting ganglion cells in the retina of the pigmented rabbit . Experimental Brain Research , 44 : 82 – 92 .
- Roth , R. L. 1979 . Decussation geometrics in the goldfish nervous system: Correlation with probability of survival . Proceedings of the National Academy of Sciences of the USA , 76 : 4131 – 4135 .
- Sarnat , H. B. and Netsky , G. 1981 . Evolution of the nervous system , Oxford, , UK : Oxford University Press .
- Sas , E. and Maler , L. 1986 . Retinofugal projections in a weakly electric gymnotid fish (Apteronotus leptorhynchus) . Neuroscience , 18 : 247 – 259 .
- Schreiber , A. M. 2006 . Asymmetric craniofacial remodeling and lateralized behavior in larval flatfish . Journal of Experimental Biology , 209 : 610 – 621 .
- Schultz , L. P. 1943 . Fishes of the Phoenix and Samoan islands collected in 1939 during the expedition of the U. S. S. “Bushnell” . Bulletin of the United States National Museum , 180 : 1 – 316 .
- Seth , A. , Culverwell , J. , Walkowicz , M. , Toro , S. , Rick , J. M. Neuhauss , S. C. 2006 . belladonna/(Ihx2) is required for neural patterning and midline axon guidance in the zebrafish forebrain . Development , 133 : 725 – 735 .
- Sovrano , V. A. and Andrew , R. J. 2006 . Eye use during viewing a reflection: Behavioural lateralisation in zebrafish larvae . Behavioural Brain Research , 167 : 226 – 231 .
- Sweeney , P. J. 1984 . Isaac Newton and the optic chiasm . Neurology , 34 : 309
- Takahashi , T. & Hori , M. 2008 . Evidence of disassortative mating in a Tanganyikan cichlid fish and its role in the maintenance of intrapopulation dimorphism . Biology Letters 2008 Jun 24. [Epub ahead of print] (DOI 10.1098/rsbl.2008.0244) .
- Tamura , K. 2005 . Ocular and blind sides in flatfishes -Mechanism of asymmetrical differentiation and its anomalies in Heterosomata- Study on the formation of asymmetrical optic chiasma during early development from the view point of developmental biology [mini-symposium in Japanese] . Nippon Suisan Gakkaishi , 71 : 1004 – 1005 .
- Taylor , J. 1750 . Mechanismus oder neue abhandl. v. d. kunstl. Zusammensetz. des menschlichen Auges . Frankfurt .
- Temminck , C. J. and Schlegel , H. 1846 . Pisces in Siebold's Fauna Japonica. Pisces . Fauna Japonica Parts , 10–14 : 173 – 269 .
- Vallortigara , G. , & Rogers , L. J. 2005 . Survival with an asymmetrical brain: Advantages and disadvantages of cerebral lateralization . Behavioral and Brain Sciences , 28 , 575 589 ; discussion 589–633 .
- Watanabe , Y. , Shirahuji , N. , & Chimura , M. 2001 . Latitudinal difference in recruitment dynamics of clupeid fishes – variable to the north, stable to the south . In F. Funk , J. Blackburn , D. Hay , A. J. Paul , R. Stephenson , R. Toresen , et al. Herring: Expectations for a new millennium 521 533 . University of Alaska Sea Grant college program , AK-SG-01-04, Fairbanks, AK, , USA .