Abstract
Context
Gamma conglutin (Cγ) from lupine species represents a potential complementary treatment for type 2 diabetes mellitus (T2DM) because of its hypoglycaemic effect. However, its underlying mechanism of action is not fully known.
Objective
To evaluate whether Cγ from Lupinus rotundiflorus M. E. Jones (Fabaceae) modulates c-Jun N-terminal kinase 1 (JNK1) expression and activation in a T2DM rat model.
Materials and methods
Gamma conglutin isolated from L. rotundiflorus seeds was characterized by SDS-PAGE. Fifteen Wistar rats with streptozotocin-induced T2DM (HG) were randomized into three groups (n = 5): vehicle administration (HG-Ctrl), oral treatment with Cγ (120 mg/kg/day) (HG-Lr) for one week, and treatment with metformin (300 mg/kg/day) (HG-Met); a healthy group (Ctrl, n = 5) was included as control. The levels of glucose and biomarkers of renal and hepatic function were measured pre- and post-treatment. Hepatic Jnk1 expression and phosphorylation of JNK1 were evaluated by qRT-PCR and western blot, respectively.
Results
Oral treatment with either Cγ or metformin reduced serum glucose level to 86.30 and 74.80 mg/dL, respectively (p ˂ 0.05), from the basal levels. Jnk1 expression was 0.65- and 0.54-fold lower (p ˂ 0.05) in the HG-Lr and HG-Met groups, respectively, than in HG-Ctrl. Treatment with Cγ decreased JNK1 phosphorylation. However, Cγ did not change the levels of kidney and liver biomarkers.
Discussion and conclusions
Treatment with Cγ from L. rotundiflorus inhibited Jnk1 expression, in vivo, suggesting JNK1 as a potential therapeutic target in diabetes and revealing one mechanism underlying the hypoglycaemic effect of lupine Cγ. Nevertheless, further studies are required.
Introduction
Type 2 diabetes mellitus (T2DM) is a chronic degenerative disease in which the pancreas cannot produce enough insulin and/or the insulin produced is inefficient, causing hyperglycaemia. This metabolic disorder is multifactorial, with some of the main risk factors for its development being age, genetic background, environment, non-nutritious diet, physical inactivity, and obesity (Chisholm et al. Citation1997; American Diabetes Association (ADA) Citation2019; International Diabetes Federation (IDF) Citation2019). Some of the characteristic symptoms of T2DM are polydipsia, polyuria, fatigue, numbness and tingling in distal extremities, blurred vision, and recurrent fungal infections (IDF Citation2019).
Type 2 diabetes mellitus is a major health problem, being one of the top 10 causes of mortality worldwide. According to the IDF (Citation2019), 9.3% of the world’s adult population (20–79 years), corresponding to 463 million people have diabetes; if the trend continues, this number will rise to 700 million people by 2045, which is equivalent to 10.90% of the adult population worldwide.
Over the years, the molecular biology of T2DM has been studied in different tissues to understand the pathophysiological mechanisms driving this complex metabolic disease. The liver is one of the key organs that controls glucose and lipid metabolism, and its function is altered in those with T2DM mostly involved with insulin resistance (Loria et al. Citation2013). While several pathways are altered in this pathology, inhibition of the insulin signalling pathway is one of the most important because this hormone is crucial for glucose homeostasis. In this regard, c-Jun N-terminal kinases (JNKs) are some of the proteins involved in insulin resistance and diabetes that have been most extensively studied (Kaneto Citation2005; Solinas and Karin Citation2010; Solinas and Becattini Citation2017). JNKs constitute a group of serine-threonine kinases belonging to the family of mitogen-activated protein kinases (MAPKs) (Chang and Karin Citation2001). They are present in different cell types and respond to diverse stressors such as saturated fatty acids, oxidative stress, inflammatory cytokines, and endoplasmic reticulum stress, theses stressors are involved in diabetes and obesity (Hibi et al. Citation1993; Kyriakis et al. Citation1994; Kaneto 2005; Solinas and Karin Citation2010; Holzer et al. Citation2011; Kaneto and Matsuoka Citation2012). Moreover, these stressors activate the MAPK signalling pathway. To activate the JNK pathway, mitogen-activated protein kinase kinase 4 (MKK4) and mitogen-activated protein kinase kinase 7 (MKK7) phosphorylate JNK on Tyr-185 and Thr-183 respectively (Yung and Giacca Citation2020).
In particular, the isozyme c-Jun N-terminal kinase 1 (JNK1) is critically involved in T2DM. In the liver, JNK1 activation contributes to insulin resistance, hepatocyte apoptosis, and hepatic inflammation. Its inhibition in the liver is related to decreases in glycaemia and improvements in insulin sensitivity (Hirosumi et al. Citation2002; Bennett et al. Citation2003; Nakatani et al. Citation2004; Kaneto Citation2005; Tarantino and Caputi Citation2011). Thus, JNK1 can be considered as a target molecule in the liver to treat T2DM.
Diabetic people need to incorporate adequate nutrition in their treatment, including a diet that helps control their glycaemia. Legumes are an excellent option for these patients as they are highly nutritious: they are rich in proteins (ranging from 20 to 40%), soluble fibre, polyunsaturated fatty acids, some vitamins, and minerals; furthermore, they are known for having a low glycemic index. In addition, some legumes contain bioactive compounds that can influence glucose metabolism (Gętek et al. Citation2014). A large number of these bioactive molecules are being investigated for their mechanism of action and some compounds were found to inhibit JNK gene and protein expression (Bhuvaneswari et al. Citation2014; Jiang et al. Citation2015; Li et al. Citation2015; El-Beih et al. Citation2019; Zhang et al. Citation2019). Further research is needed to understand the inhibition of these molecules by natural compounds. In this sense, a leguminous whose compounds could modify JNK is the lupine. This leguminous has drawn attention for its potential health benefits, especially for treating T2DM. The genus Lupinus (Fabaceae) includes a wide variety of plant species distributed worldwide, which can be found in the Mediterranean region, North Africa, Australia, and America (McVaugh Citation1987). Lupine species can be classified as domesticated and wild. In this respect, Mexico has nearly 100 wild species; and Lupinus rotundiflorus M. E. Jones is one of the most abundant (McVaugh Citation1987; Dunn Citation2001; Ruiz-López et al. Citation2019).
In recent years, although several biological effects have been attributed to lupine proteins, including the modulation of glucose and lipid metabolism, further investigation is required to understand the mechanism of action of these proteins. Among them, the most studied is gamma conglutin (Cγ). The hypoglycaemic effect of Cγ is outstanding, as demonstrated by an oral glucose tolerance test performed in healthy rats, in which Cγ induced a dose-dependent reduction in glycaemia (Magni et al. Citation2004). In addition, treatment of hyperglycaemic rats with Cγ from L. albus L. reduced blood glucose and insulin resistance (Lovati et al. Citation2012); also, an improvement in blood insulin concentration along with an increase in insulin-1 gene expression and pancreatic insulin content have been reported in neonatal rats with streptozotocin (STZ)-induced diabetes that were treated with Cγ from L. albus (Vargas-Guerrero et al. Citation2014). Notably, Cγ has been reported to lower hepatic glucose production in two different murine models, mainly by downregulating the gene expression of glucose-6-phosphatase (González-Santiago et al. Citation2017). In mouse myoblasts, Cγ can activate insulin receptor substrate 1 (IRS-1)/phosphatidylinositol 3-kinase (PI3K) and other kinases involved in the insulin signalling cascade, suggesting that this lupine protein may act similarly to insulin (Terruzzi et al. Citation2011).
Notably, the beneficial properties of Cγ are not only present in L. albus but also in other lupine species. The Cγ isolated from L. angustifolius L. has been reported to upregulate the expression of enzymes involved in insulin signalling and reduce oxidative stress in vitro (Lima-Cabello et al. Citation2019) besides, an hydrolysed Cγ fraction from L. mutabilis Sweet has been demonstrated to enhance glucose uptake and inhibit hepatic gluconeogenesis (Muñoz et al. Citation2018). Furthermore, a high sequence identity was found among Cγ from diverse species and varieties of lupines (Foley et al. Citation2015). Therefore, it is likely that Cγ proteins from different lupine species maintain their beneficial health effects. In that sense, it would be interesting to study in more depth the antidiabetic activity of other Cγ isoforms.
This study evaluates the effects of oral treatment with Cγ from L. rotundiflorus on hepatic gene expression and protein activation of JNK1 in a rat model of STZ-induced T2DM. Considering the potential benefits of Cγ in diabetes treatment, this study could offer additional novel insights into the antidiabetic mechanism of this protein.
Materials and methods
Plant material
Seeds of L. rotundiflorus were collected at Chiquilistlán, Jalisco, Mexico, in April 2015. A specimen has been authenticated by the taxonomist Dr. Daniel Sánchez Carbajal of Laboratorio Nacional de Identificación y Caracterización Vegetal (LaniVeg) and deposited at the Herbarium Luz María Villarreal de Puga of Institute of Botany, University of Guadalajara (voucher specimen number 210430). The authenticated seeds were hulled, powdered by a grain mill (ALMET, Tlaquepaque, Jalisco, Mexico), and sifted.
Isolation and characterization of Cγ
L. rotundiflorus flour was defatted with hexane in a Soxhlet extractor. Cγ solution was obtained using a previously described method (Martínez-Ayala and Paredes-López Citation2001; Vargas-Guerrero et al. Citation2014) that includes precipitation procedures, centrifugation and dialysis. Afterwards, the Cγ solution was lyophilized in a FreeZone® 4.5 freeze dryer (Labconco, Kansas, MO, USA) at −50 °C and 0.04 mbar, for ∼6 h. Lyophilized Cγ was identified by SDS-PAGE at 12% following the next steps: Cγ samples (2 μg) were mixed in Laemmli Sample Buffer (Bio-Rad, Segrate, Milan, Italy) and assayed under reducing and nonreducing conditions (with and without 1% β-mercaptoethanol). The protein was incubated for 2 min at 90 °C and then centrifuged for 10 min at 10,640 ×g at 4 °C. The Cγ samples were characterized by SDS-PAGE using the mini gel electrophoresis system mini-PROTEAN® Tetra Cell (Bio-Rad, Segrate, Milan, Italy). The Coomassie Brilliant Blue G-250 dye (Bio-Rad, Milan, Italy) was used to stain the proteins in the SDS-PAGE gels.
Experimental animals
Twenty male Wistar rats weighing between 200 and 250 g were obtained from Bioterium of the University of Guadalajara. Animals were maintained in a 12 h light/dark cycle under standard laboratory conditions of temperature (24 ± 2 °C) and humidity (55 ± 5%), and provided with standard rodent diet (LabDiet 5001, LabDiet, St. Louis, MO, USA) and water ad libitum. All animal procedures were approved by the institutional ethics committee (CI/101/2017) and conducted in accordance with the guidelines for production, care and use of laboratory animals established in the Mexican Official Standard (NOM-062-ZOO-1999).
T2DM induction and Cγ treatment
The experimental animals were randomly divided into two groups: 1) a control group (Ctrl, n = 5) consisting of healthy animals that received saline as a vehicle (0.90% w/v NaCl); 2) a diabetic group (n = 15) in which type 2 diabetes was induced by a single dose of STZ (65 mg/kg body weight) (Sigma, St. Louis, MO, USA) administrated intraperitoneally. After 72 h, rats with fasting glucose levels >200 mg/dL were considered diabetic and divided into three subgroups as follows: 2a) HG-Ctrl group (n = 5) comprising, diabetic rats administered with vehicle; 2 b) HG-Lr (n = 5) comprising, diabetic rats treated with Cγ of L. rotundiflorus (120 mg/kg/day); and 2c) HG-Met group (n = 5) consisting of diabetic rats administered with metformin (300 mg/kg/day). All the treatments above mentioned (groups 2a–2c) were administered orally for one week (seven days).
Biochemical analysis
Before (pre-) and after (post-) treatment, overnight fasted rats were anaesthetized with tiletamine-zolazepam (80 mg/kg, Zoletil®50, Virbac, Carros, France) and blood was obtained from the retro-orbital plexus. Blood was centrifuged for 10 min at 1,300 ×g, at 4 °C; serum was then separated and stored at −70 °C until use. The serum concentrations of glucose, alanine aminotransferase (ALT), aspartate aminotransferase (AST), creatinine, and urea, were quantified using biochemical reagents from BioSystems (Barcelona, Spain) and the semi-automatic analyser BTS-350 (BioSystems, Barcelona, Spain).
Quantification of mRNA expression by qRT-PCR
After completing the one-week treatment, all rats were anaesthetized as previously mentioned and sacrificed to collect samples of liver tissue. Total RNA from liver homogenates was extracted using the RNeasy® Protect Mini Kit (QIAGEN, Hilden, Germany); afterwards, total RNA (2 μg) was retrotranscribed into cDNA using the Transcriptor First Strand cDNA Synthesis Kit (Roche, Mannheim, Germany). Gene expression of Jnk1 and Rps18 (reference gene) was quantified by qRT-PCR using probes from the Universal Probe Library (UPL, Roche, Mannheim, Germany) and LightCycler® TaqMan® Master Mix (Roche, Mannheim, Germany). The primer sequences, UPL probes, and annealing temperatures used are shown in . The general reaction conditions were as follows: initial denaturation step at 95 °C for 10 min; 40 cycles of denaturation at 95 °C for 10 s; annealing for 10 s; and elongation at 72 °C (see for annealing temperatures and elongation times). All reactions were performed in triplicate employing a LightCycler 1.5 Instrument (Roche, Mannheim, Germany). Relative gene expression was quantified using the 2–ΔΔCt method (Pfaffl Citation2001).
Table 1. Primer sequences and PCR conditions used in qRT-PCR.
Western blotting
Liver tissue was frozen in dry ice and stored at −70 °C until use. To extract total protein, a fragment of frozen tissue was homogenized with a rotor-stator homogenizer in ice-cold Tissue Protein Extraction Reagent (T-PER, Thermo Fisher Scientific, Waltham, MA, USA) containing a protease/phosphatase inhibitor cocktail (Cell Signalling Technology, Danvers, MA, USA). Protein concentration was measured using the PierceTM BCA Protein Assay Kit (Thermo Fisher Scientific, Waltham, MA, USA), according to the manufacturer’s instructions. An equivalent amount of proteins (10 μg) from each sample were separated on a 10% SDS-PAGE gel and transferred to a polyvinylidene difluoride (PVDF) membrane (Bio-Rad, Segrate, Milan, Italy) using a semi-dry membrane blotting system (Bio-Rad, Segrate, Milan, Italy). The PVDF membrane was blocked with 5% non-fat milk in Tris-buffered saline containing 0.1% Tween-20 (TBST) and incubated overnight at 4 °C in the same buffer with the following primary antibodies (dilution 1:1000): mouse monoclonal anti-JNK1 (catalog 3708), mouse monoclonal anti-phosphorylated JNK1 (catalog 9255), and mouse monoclonal anti-β-actin (catalog 3700) (Cell Signalling Technology, Danvers, MA, USA). Membranes were incubated for 1 h with anti-mouse horseradish peroxidase (HRP)-conjugated secondary antibody (dilution 1:6000, catalog ab6789, Abcam, Cambridge, UK). After washing the membranes, antibody binding was detected by chemiluminescence using the Amersham ECL Prime Western Blotting Detection Kit (GE Healthcare, Freiburg, Germany). Membranes were imaged with the MicroChemi 4.2 system (DNR Bio Imaging Systems, Ltd., Neve Yamin, Israel) and bands intensities were measured by densiometric quantification using the Image J 1.4 software (Rasband WS. 1997–2015).
Statistical analysis
All data were expressed as mean ± standard error of the mean (SEM). Intragroup comparisons for the levels of biochemical parameters were analysed by a paired t-test. Comparisons between groups were conducted by a one-way analysis of variance (ANOVA) followed by a post hoc test. A p value < 0.05 was considered as statistically significant. Statistical analysis was performed using the PASW statistical software, version 18.0 (Chicago, IL, USA).
Results
Cγ characterization
After carrying out the Cγ extraction from L. rotundiflorus seeds we characterized the protein fraction obtained by SDS-PAGE to validate the isolation of Cγ. Under native (nonreducing) conditions a major band of ∼49 kDa was observed, while under denaturing conditions, two bands of about 17 and 29 kDa were evident (). These results indicated that the protein isolated from L. rotundiflorus corresponded to Cγ.
Treatment with L. rotundiflorus Cγ reduces serum glucose levels in diabetic rats
To evaluate the effect of L. rotundiflorus Cγ in T2DM, we first established a rat model of T2DM induced by STZ. As expected, a STZ-induced diabetic rats became hyperglycaemic (glucose > 200 mg/dL), whereas the serum glucose levels in the control group, without diabetes induction and without treatment (Ctrl) remained normal (62.00 ± 3.20 mg/dL) (). After treatments (post-treatment values), serum glucose was reduced by 26% and 24% in HG-Lr and HG-Met rats, respectively, when compared with the corresponding pre-treatment levels (245.00 ± 53.30 and 229.20 ± 28.30 vs. 331.30 ± 27.60 and 304.00 ± 23.30; p < 0.05). That results indicated that the oral administration of Cγ from L. rotundiflorus significantly reduced serum glucose levels and the reduction was similar to that resulting from treatment with metformin.
Table 2. Pre- and post-treatment levels of serum biochemical parameters.
Effect of Cγ treatment in kidney and liver biomarkers
The levels of kidney and liver biomarkers were measured to verify the absence of damage in these organs after Cγ and metformin administration (). At the end of the one-week treatment, no significant changes in serum levels of urea and creatinine were found in any of the groups, relative to their pre-treatment levels. In addition, the hepatic biomarker ALT remained unchanged in all groups during the experimental period. However, a significant increase in serum AST levels was noted in the group treated with metformin (p ˂ 0.05).
Cγ treatment downregulates Jnk1 expression in liver
We assessed the effect of Cγ treatment on Jnk1 expression in the liver by qRT-PCR, as a possible mechanism of this protein hypoglycaemic properties. The control diabetic group (HG-Ctrl), showed significantly higher mRNA levels of Jnk1, compared with the nondiabetic group (Ctrl) (p ˂ 0.05) (). Conversely, both Cγ and metformin treatments significantly downregulated Jnk1, expression compared to that in the HG-Ctrl group, with this reduction being slightly more pronounced in the group treated with Cγ (0.65- and 0.54-fold in HG-Lr and HG-Met, respectively; p ˂ 0.05) ().
Figure 2. Relative mRNA expression of Jnk1 in the liver of treated and untreated rats with experimental type 2 diabetes. Data are expressed as mean ± SEM of five rats per group. *p ˂ 0.05, **p ˂ 0.01 when compared with HG-Ctrl group; δp ˂ 0.05 when compared with Ctrl group. Ctrl, control group, nondiabetic rats without treatment; HG-Ctrl, diabetic rats administered with vehicle (saline); HG-Lr, diabetic rats treated with Cγ from L. rotundiflorus; HG-Met, diabetic rats treated with metformin.
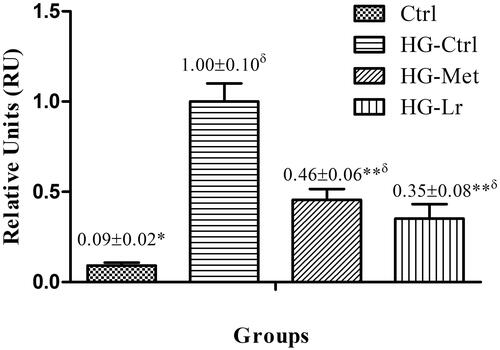
L. rotundiflorus Cγ lowers JNK1 protein activation in the liver
The effect L. rotundiflorus Cγ on the activation of JNK1 in hepatic tissue was assayed by western blotting (). Interestingly, we observed that protein phosphorylation followed a similar trend to Jnk1 expression measured by qRT-PCR. While JNK1 phosphorylation slightly increased in the liver of HG-Ctrl group, it tended to reduce upon treatment with Cγ, in the HG-Lr group. A similar but less pronounced trend was observed in the HG-Met group ().
Figure 3. Western blot analysis of phosphorylated JNK1 in the liver of treated and untreated rats with experimental type 2 diabetes. (A) Representative blots are shown for each group. (B) Relative quantification of phosphorylated JNK1 expressed as phosphorylated JNK1/total JNK1 ratio, using β-actin as a loading control. Values represent mean ± SEM of five rats per group. Ctrl, control group, nondiabetic rats without treatment; HG-Ctrl, diabetic rats administered with vehicle (saline); HG-Lr, diabetic rats treated with Cγ from L. rotundiflorus; HG-Met, diabetic rats treated with metformin; pJNK1, phosphorylated JNK1.
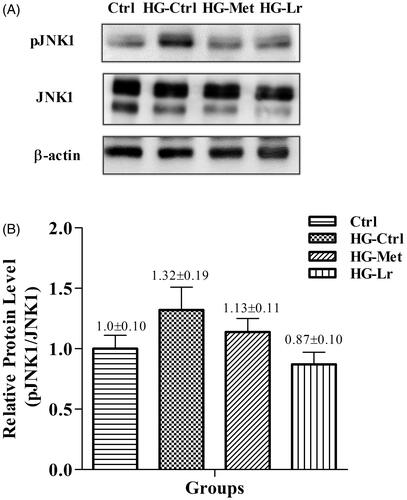
These results suggest that the reduced gene expression and protein activation of JNK1 after Cγ treatment could be involved in the hypoglycaemic effect of this protein.
Discussion
Lupines have gained increasing importance because of the beneficial health effects of their components (Mane et al. Citation2018). The Cγ protein has obtained special interest due its hypoglycaemic activity, which has been already demonstrated in vitro, in vivo, and in human participants in clinical trials (Magni et al. Citation2004; Bertoglio et al. Citation2011; Lovati et al. Citation2012; Vargas-Guerrero et al. Citation2014; Mane et al. Citation2018). Although, the effects of lupine Cγ on several molecules involved in the glucose metabolism have been evaluated (Vargas-Guerrero et al. Citation2014; González-Santiago et al. Citation2017; Muñoz et al. Citation2018; Sandoval-Muñíz et al. Citation2018), its full mechanism of action remains unknown. Besides, most studies have focussed on domesticated lupine species, the knowledge available on Cγ from wild lupines is still limited. Therefore, in this study we evaluated the effect of Cγ from L. rotundiflorus, a wild lupine species, on gene expression and protein activation of JNK1.
Here, Cγ was isolated from L. rotundiflorus and characterized by SDS-PAGE. A protein band of ∼49 kDa was obtained under native conditions while two bands were observed under reducing conditions (17 and 29 kDa), which corresponded to the previously reported subunits of Cγ (Duranti et al. Citation2008). These results are in agreement with those reported for Cγ from domesticated lupine species isolated by different extraction methods (Bertoglio et al. Citation2011; Lovati et al. Citation2012; Vargas-Guerrero et al. Citation2014), and indicate that the protein extracted from L. rotundiflorus seeds corresponds to Cγ.
Differences in Cγ lupine species have emphasized the importance of verifying the biological effects of Cγ among species (Foley et al. Citation2015; Mane et al. Citation2018). In previous studies, Cγ extracted from L. albus (domesticated species) was evaluated using the same treatment period (one week) and dose (120 mg/kg/day) employed in the present study, and a reduction of 17–27% in serum glucose levels was reported (Vargas-Guerrero et al. Citation2014; González-Santiago et al. Citation2017; Sandoval-Muñíz et al. Citation2018). In another study using male Sprague-Dawley rats, the administration of 28 mg/kg of Cγ from L. albus for three weeks reduced glucose levels by 22% compared with those without treatment (Lovati et al. Citation2012). In our study, the percent reduction in serum glucose levels following the administration of Cγ from L. rotundiflorus (wild species) was similar to those reported in the aforementioned studies that used a domesticated species of lupines. This suggests that the hypoglycaemic properties of Cγ, as well as its other effects, are similar across wild and domesticated lupines. Moreover, the glucose-lowering effect observed in the HG-Lr group was comparable to that in metformin-treated rats (HG-Met), consistent with previous described reports (Magni et al. Citation2004).
At the same time, it is important to verify the absence of kidney and liver toxicity after administering any treatment. Here, no significant changes in serum urea and creatinine levels were observed in the group treated with Cγ from L. rotundiflorus. Similarly, the serum ALT and AST levels remained unchanged in this group when compared with baseline levels. Thus, the administration of Cγ from L. rotundiflorus at the dose and time tested in this study does not seem to cause any renal or liver damage. This is consistent with prior results obtained for the Cγ of the domesticated species L. albus (González-Santiago et al. Citation2017).
In T2DM, several pathways are dysregulated in the liver, including the mRNA expression and activation of JNK1, both of which are abnormally high in this pathology (Nakatani et al. Citation2004; Kaneto Citation2005; Tarantino and Caputi Citation2011). In agreement, in the present study, the mRNA expression of Jnk1 was upregulated in diabetic rats compared with the nondiabetic control group. However, oral administration of metformin or Cγ significantly lowered Jnk1 expression in the liver. Interestingly, this reduction was more pronounced in the group treated with Cγ. Since JNK1 downregulation diminishes the expression of key gluconeogenic enzymes, our findings could be related with the reported effect of Cγ in reducing glucose production in the liver. In agreement with this hypothesis, in vivo administration of Cγ from L. albus reduced the expression of glucose-6-phosphatase; moreover, the Cγ of L. mutabilis was reported to inhibit hepatic gluconeogenesis in vitro (Nakatani et al. Citation2004; Loria et al. Citation2013; González-Santiago et al. Citation2017; Muñoz et al. Citation2018). Therefore, the downregulation of Jnk1 observed in this study could represent one of the mechanisms by which Cγ exerts its effects on gluconeogenic enzymes, supporting the idea that JNK1 is a potential therapeutic target in T2DM.
The effects of plant compounds on JNK have also been evaluated at the protein level by western blotting. In a previous study, a protein extract of Momordica charantia administrated in different doses for eight weeks in diabetic rats, reduced the mRNA and protein expression of JNK and SOCS-3 (Ma et al. Citation2017). In our study, after one week of treatment, a trend towards lower JNK1 phosphorylation was observed in rats administered with Cγ from L. rotundiflorus, as compared with diabetic rats receiving vehicle. Hyperglycaemia has been reported to increases reactive oxygen species (ROS), thus activating the JNK pathway (Kaneto et al. Citation2010). In this regard, the decreased JNK1 activation could be attributed to the reduced ROS levels, caused by the reduction of hyperglycaemia after Cγ treatment or the decrease in the levels of inflammatory cytokines such as tumour necrosis factor α (TNFα). Nevertheless, further studies are required to corroborate it.
Treatment with Cγ tended to repress JNK1 more than metformin and even seemed to inhibit protein phosphorylation to levels slightly below those of healthy, nondiabetic rats. Metformin is one of the main drugs used for T2DM treatment. It reduces hyperglycaemia, gluconeogenesis, and hyperinsulinemia, and improves insulin sensitivity and glucose transporter type 4 (GLUT4) translocation, which is key for insulin-induced glucose uptake, among other effects. Besides, it has been reported that metformin inhibits hepatic JNK1 activation in vitro (Conde de la Rosa et al. Citation2015; Garabadu and Krishnamurthy Citation2017; Wang et al. Citation2017; Lu et al. Citation2019). Interestingly, the effects of Cγ treatment observed in this study were similar to those of metformin not only in terms of lowering glucose levels, but also in terms JNK1 gene expression and protein activation. This provides additional evidence in support of the potential use of Cγ as a complement of the drugs used in the guidelines for T2DM treatment. Therefore, it is necessary to evaluate the effect of combined Cγ administration with oral hypoglycaemic agents, especially with metformin, which is the first-line drug for treat T2DM.
A limitation of our study was that we did not evaluate molecules that stimulates.
JNK pathway activation, such as inflammatory cytokines and ROS, or are involved in the insulin pathway. Therefore, further studies are required to assess the effects of Cγ from L. rotundiflorus on these molecules.
Conclusions
Oral administration of Cγ from L. rotundiflorus inhibited both gene expression and protein activation of JNK1 in an animal model of T2DM. This suggests that Cγ exerts its hypoglycaemic effects, at least partially, by modulating the quantity and activation of JNK1 in the liver and supports the hypothesis that this protein is a potential drug target for T2DM treatment. In the future, it will be interesting to explore other possible therapeutic targets involved in the JNK pathway and investigate if Cγ proteins from other lupine species also act through a similar mechanism.
Acknowledgments
The authors thank Adrián Ramírez de Arellano and Lucía De la Cruz Color for their valuable technical assistance.
Disclosure statement
No potential conflict of interest was reported by the author(s).
Additional information
Funding
References
- American Diabetes Association (ADA). 2019. Standards of medical care in diabetes. Diabetes Care. 42:S13–S18.
- Bennett BL, Satoh Y, Lewis AJ. 2003. JNK: a new therapeutic target for diabetes. Curr Opin Pharmacol. 3(4):420–425.
- Bertoglio JC, Calvo MA, Hancke JL, Burgos RA, Riva A, Morazzoni P, Ponzone C, Magni C, Duranti M. 2011. Hypoglycemic effect of lupin seed γ-conglutin in experimental animals and healthy human subjects. Fitoterapia. 82(7):933–938.
- Bhuvaneswari S, Yogalakshmi B, Sreeja S, Anuradha CV. 2014. Astaxanthin reduces hepatic endoplasmic reticulum stress and nuclear factor-κB-mediated inflammation in high fructose and high fat diet-fed mice. Cell Stress Chaperones. 19(2):183–191.
- Chang L, Karin M. 2001. Mammalian MAP kinase signalling cascades. Nature. 410(6824):37–40.
- Chisholm DJ, Campbell LV, Kraegen EW. 1997. Pathogenesis of the insulin resistance syndrome (syndrome X). Clin Exp Pharmacol Physiol. 24(9–10):782–784.
- Conde de la Rosa L, Vrenken TE, Buist-Homan M, Faber KN, Moshage H. 2015. Metformin protects primary rat hepatocytes against oxidative stress-induced apoptosis. Pharmacol Res Perspect. 3(2):e00125.
- Dunn DB. 2001. Flora fanerogámica del Valle de México [Phanerogamic Flora of the Valley of Mexico]. 2nd ed. Calderón G, Rzedowski J, editors. Pátzcuaro: Instituto de Ecología. p. 290–299. Spanish.
- Duranti M, Consonni A, Magni C, Sessa F, Scarafoni A. 2008. The major proteins of lupin seed: characterisation and molecular properties for use as functional and nutraceutical ingredients. Trends Food Sci Technol. 19(12):624–633.
- El-Beih NM, Ramadan G, El-Husseiny EA, Hussein AM. 2019. Effects of pomegranate aril juice and its punicalagin on some key regulators of insulin resistance and oxidative liver injury in streptozotocin-nicotinamide type 2 diabetic rats. Mol Biol Rep. 46(4):3701–3711.
- Foley RC, Jimenez-Lopez JC, Kamphuis LG, Hane JK, Melser S, Singh KB. 2015. Analysis of conglutin seed storage proteins across lupin species using transcriptomic, protein and comparative genomic approaches. BMC Plant Biol. 15:106.
- Garabadu D, Krishnamurthy S. 2017. Metformin attenuates hepatic insulin resistance in type-2 diabetic rats through PI3K/Akt/GLUT-4 signalling independent to bicuculline-sensitive GABAA receptor stimulation. Pharm Biol. 55(1):722–728.
- Gętek M, Czech N, Muc-Wierzgoń M, Grochowska-Niedworok E, Kokot T, Nowakowska-Zajdel E. 2014. The active role of leguminous plant components in type 2 diabetes. Evid Based Complement Alternat Med. 2014:293961.
- González-Santiago AE, Vargas-Guerrero B, García-López PM, Martínez-Ayala AL, Domínguez-Rosales JA, Gurrola-Díaz CM. 2017. Lupinus albus conglutin gamma modifies the gene expressions of enzymes involved in glucose hepatic production in vivo. Plant Foods Hum Nutr. 72(2):134–140.
- Hibi M, Lin A, Smeal T, Minden A, Karin M. 1993. Identification of an oncoprotein- and UV-responsive protein kinase that binds and potentiates the c-Jun activation domain. Genes Dev. 7(11):2135–2148.
- Hirosumi J, Tuncman G, Chang L, Görgün C, Uysal K, Maeda K, Karin M, Hotamisligil G. 2002. A central role for JNK in obesity and insulin resistance. Nature. 420(6913):333–336.
- Holzer RG, Park E-J, Li N, Tran H, Chen M, Choi C, Solinas G, Karin M. 2011. Saturated fatty acids induce c-Src clustering within membrane subdomains, leading to JNK activation. Cell. 147(1):173–184.
- International Diabetes Federation (IDF). 2019. IDF Diabetes Atlas. 9th ed.:4–15.
- Jiang S, Wang Y, Ren D, Li J, Yuan G, An L, Du P, Ma J. 2015. Antidiabetic mechanism of Coptis chinensis polysaccharide through its antioxidant property involving the JNK pathway. Pharm Biol. 53(7):1022–1029.
- Kaneto H, Katakami N, Matsuhisa M, Matsuoka TA. 2010. Role of reactive oxygen species in the progression of type 2 diabetes and atherosclerosis. Mediators Inflamm. 2010:453892.
- Kaneto H, Matsuoka T. 2012. Involvement of oxidative stress in suppression of insulin biosynthesis under diabetic conditions. Int J Mol Sci. 13(10):13680–13690.
- Kaneto H. 2005. The JNK pathway as a therapeutic target for diabetes. Expert Opin Ther Targets. 9(3):581–592.
- Kyriakis JM, Banerjee P, Nikolakaki E, Dai T, Rubie EA, Ahmad MF, Avruch J, Woodgett JR. 1994. The stress-activated protein kinase subfamily of c-Jun kinases. Nature. 369(6476):156–160.
- Li S, Jiang W, Hu S, Song W, Ji L, Wang Y, Cai L. 2015. Fucosylated chondroitin sulphate from Cusumaria frondosa mitigates hepatic endoplasmic reticulum stress and inflammation in insulin resistant mice. Food Funct. 6(5):1547–1556.
- Lima-Cabello E, Alché JD, Morales-Santana S, Clemente A, Jimenez-Lopez JC. 2019. Narrow-leafed lupin (Lupinus angustifolius L.) seeds gamma-conglutin is an anti-inflammatory protein promoting insulin resistance improvement and oxidative stress amelioration in PANC-1 pancreatic cell-line. Antioxidants. 9(1):12.
- Loria P, Lonardo A, Anania F. 2013. Liver and diabetes. A vicious circle. Hepatol Res. 43(1):51–64.
- Lovati MR, Manzoni C, Castiglioni S, Parolari A, Magni C, Duranti M. 2012. Lupin seed γ-conglutin lowers blood glucose in hyperglycaemic rats and increases glucose consumption of HepG2 cells. Br J Nutr. 107(1):67–73.
- Lu CC, Chiang JH, Tsai FJ, Hsu YM, Juan YN, Yang JS, Chiu HY. 2019. Metformin triggers the intrinsic apoptotic response in human AGS gastric adenocarcinoma cells by activating AMPK and suppressing mTOR/AKT signaling. Int J Oncol. 54(4):1271–1281.
- Ma C, Yu H, Xiao Y, Wang H. 2017. Momordica charantia extracts ameliorate insulin resistance by regulating the expression of SOCS-3 and JNK in type 2 diabetes mellitus rats. Pharm Biol. 55(1):2170–2177.
- Magni C, Sessa F, Accardo E, Vanoni M, Morazzoni P, Scarafoni A, Duranti M. 2004. Conglutin gamma, a lupin seed protein, binds insulin in vitro and reduces plasma glucose levels of hyperglycemic rats. J Nutr Biochem. 15(11):646–650.
- Mane SP, Johnson SK, Duranti M, Pareek VK, Utikar RP. 2018. Lupin seed γ-conglutin: extraction and purification methods – a review. Trends Food Sci Technol. 73:1–11.
- Martínez-Ayala AL, Paredes-López O. 2001. Molecular characterization of the β-conglutin of lupin seeds. J Food Biochemistry. 25(1):15–31.
- McVaugh R. 1987. Lupinus. In: McVaugh R, Anderson WR, editors. Flora Novo-Galiciana: a descriptive account of the vascular plants of Western Mexico. Ann Arbor (MI): The University of Michigan Press; p. 580–599.
- Muñoz EB, Luna-Vital DA, Fornasini M, Baldeón ME, Gonzalez de Mejia E. 2018. Gamma-conglutin peptides from Andean lupin legume (Lupinus mutabilis Sweet) enhanced glucose uptake and reduced gluconeogenesis in vitro. J Funct Foods. 45:339–347.
- Nakatani Y, Kaneto H, Kawamori D, Hatazaki M, Miyatsuka T, Matsuoka T, Kajimoto Y, Matsuhisa M, Yamasaki Y, Hori M. 2004. Modulation of the JNK pathway in liver affects insulin resistance status. J Biol Chem. 279(44):45803–45809.
- Pfaffl MW. 2001. A new mathematical model for relative quantification in real-time RT–PCR. Nucleic Acids Res. 29:2003–2007.
- Ruiz-López MA, Barrientos-Ramírez L, García-López PM, Valdés-Miramontes EH, Zamora-Natera JF, Rodríguez-Macias R, Salcedo-Pérez E, Bañuelos-Pineda J, Vargas-Radillo JJ. 2019. Nutritional and bioactive compounds in Mexican lupin beans species: a mini-review. Nutrients. 11(8):1785.
- Sandoval-Muñíz R de J, Vargas-Guerrero B, Guzmán TJ, García-López PM, Martínez-Ayala AL, Domínguez-Rosales JA, Gurrola-Díaz CM. 2018. Lupin gamma conglutin protein: effect on Slc2a2, Gck and Pdx-1 gene expression and GLUT2 levels in diabetic rats. Rev Bras Farmacogn. 28(6):716–723.
- Solinas G, Becattini B. 2017. JNK at the crossroad of obesity, insulin resistance, and cell stress response. Mol Metab. 6(2):174–184.
- Solinas G, Karin M. 2010. JNK1 and IKKbeta: molecular links between obesity and metabolic dysfunction. Faseb J. 24(8):2596–2611.
- Tarantino G, Caputi A. 2011. JNKs, insulin resistance and inflammation: a possible link between NAFLD and coronary artery disease. World J Gastroenterol. 17(33):3785–3794.
- Terruzzi I, Senesi P, Magni C, Montesano A, Scarafoni A, Luzi L, Duranti M. 2011. Insulin-mimetic action of conglutin-γ, a lupin seed protein, in mouse myoblasts. Nutr Metab Cardiovasc Dis. 21(3):197–205.
- Vargas-Guerrero B, García-López PM, Martínez-Ayala AL, Domínguez-Rosales JA, Gurrola-Díaz CM. 2014. Administration of Lupinus albus gamma conglutin (Cγ) to n5 STZ rats augmented Ins-1 gene expression and pancreatic insulin content. Plant Foods Hum Nutr. 69(3):241–247.
- Wang YW, He SJ, Feng X, Cheng J, Luo YT, Tian L, Huang Q. 2017. Metformin: a review of its potential indications. Drug Des Devel Ther. 11:2421–2429.
- Yung JHM, Giacca A. 2020. Role of c-Jun N-terminal Kinase (JNK) in obesity and type 2 diabetes. Cells. 9(3):706.
- Zhang Y, Yang S, Zhang M, Wang Z, He X, Hou Y, Bai G. 2019. Glycyrrhetinic acid improves insulin-response pathway by regulating the balance between the Ras/MAPK and PI3K/Akt pathways. Nutrients. 11(3):604.