Abstract
Context
Although Tongguan capsule (TGC) is used in the treatment of coronary atherosclerotic disease, the exact mechanism remains unclear.
Objective
Network pharmacology and experimental validation were applied to examine the mechanism of TGC for treating myocardial ischemia-reperfusion injury (MIRI).
Materials and methods
The components and candidate targets were searched based on various databases such as TCMSP, TCMID, BATMAN-TCM. The binding ability was determined by molecular docking. The ischemia-reperfusion (I/R) model was constructed by ligating the left anterior descending (LAD) coronary artery. APOE-/- mice were divided into three groups (n = 6): Sham group, I/R group, and TGC group (1 g/kg/d). To further verification, HCAEC cells were subjected to hypoxia-reoxygenation (H/R) to establish in vitro model.
Results
The compounds, such as quercetin, luteolin, tanshinone IIA, kaempferol and bifendate, were obtained after screening. The affinity values of the components with GSK-3β, mTOR, Beclin-1, and LC3 were all <-5 kcal/mol. In vivo, TGC improved LVEF and FS, reducing infarct size. In vitro, Hoechst 33258 staining result showed TGC inhibited apoptosis. Compare with the H/R model, TGC treatment increased the levels of GSK-3β, LC3, and Beclin1, while decreasing the expression of mTOR and p62 (p < 0.05).
Discussion and Conclusion
The findings revealed that TGC exerted a cardioprotective effect by up regulating autophagy-related proteins through the mTOR pathway, which may be a therapeutic option for MIRI. However, there are still some limitations in this research. It is necessary to search more databases to obtain information and further demonstrated through randomized controlled trials for generalization.
Introduction
Reperfusion therapy is a milestone achievement in the management of acute myocardial infarction (AMI) (Lee and Hanif Citation2018). Early reperfusion therapy can restore blood flow through the occluded coronary artery immediately upon diagnosis, thus protecting heart function and improving the prognosis of patients with AMI (Vogel et al. Citation2019). However, even if the arterial stenosis or occlusion is relieved, some patients experience slow coronary blood flow, suggesting cardiac microvascular disorder (Kumar et al. Citation2019; Wang et al. Citation2020a, Citation2020b). In addition, ischemia-reperfusion (I/R) causes functional damage of endothelial cells, which is the crucial mechanism of microvascular diseases (Zhou and Toan Citation2020; Wang et al. Citation2020a). Therefore, endothelial homeostasis is critical for alleviating myocardial ischemia-reperfusion injury (MIRI) (Davidson et al. Citation2019).
Previous studies have shown that traditional Chinese medicine (TCM) may have its own unique role in the treatment of MIRI (Wang et al. Citation2020c; Xie et al. Citation2021). Tongguan capsule (TGC), a proprietary Chinese medicine, composed of Astragalus membranaceus Moench (Fabaceae), Salvia miltiorrhiza Bunge (Lamiaceae), Borneol, and Leech, play the role on promoting blood circulation, invigorating qi, replenishing energy and improving metabolism in TCM (Shuai et al. Citation2018; Ma et al. Citation2019a). Based on accurate fingerprint analysis with high-performance liquid chromatography, TGC contains substantial active compounds, such as tanshinone IIA and salvianolic acid B (Mao et al. Citation2020). TGC improves cardiac function in patients with coronary atherosclerotic disease, inhibiting postoperative ventricular remodeling and vascular restenosis (Shuai et al. Citation2018; Ma et al. 2019; Zhou et al. Citation2021).
However, the molecular mechanism of TGC is unclear, which needs to be preliminarily explored through network pharmacology. Network pharmacology, based on bioinformation data analysis, is devoted to discover the complex mechanisms of drugs by searching for components and targets (Kibble et al. Citation2015; Muhammad et al. Citation2018; Zhang et al. Citation2019a). Molecular docking is applied to the study of interactions between ligands and receptors by simulating the binding (Pinzi and Rastelli Citation2019). In this study, compounds and targets were screened through network pharmacological analysis, verified by molecular docking and experiment, to elucidate the mechanism of TGC for treating MIRI, providing a reference for clinical medication. The workflow of this study was shown in .
Materials and methods
Active ingredient screening
The TGC active ingredients, with drug-likeness (DL) ≥0.18 and oral bioavailability (OB) ≥30%, were screened from TCMSP (https://tcmspw.com/tcmsp.php), TCMID (http://119.3.41.228:8000/tcmid/) and BATMAN-TCM (http://bionet.ncpsb.org.cn/batman-tcm/). The targets of the compounds were obtained through TCMSP, Swiss TargetPrediction (http://swisstargetprediction.ch/), and STITCH (http://stitch.embl.de/). Simultaneously, the UniProt database (https://www.uniprot.org/) was applied for gene name standardization.
Identification of candidate targets
The targets related to ‘myocardial ischemia-reperfusion injury’ were retrieved through the GeneCards (https://www.genecards.org), Online Mendelian Inheritance in Man (OMIM, https://omim.org), and TTD (http://db.idrblab.net/ttd/). The overlapping genes with MIRI and active components were identified as candidate targets.
PPI network construction
The candidate targets were imported into String (https://string-db.org/), and the condition was set as Homo sapiens with a confidence of 0.95 to obtain the relevant information on protein interactions. PPI network was constructed through Cytoscape (V3.7.1) and CytoNCA plug-in was devoted to calculate the topology index, including betweenness centrality (BC), closeness centrality (CC), degree centrality (DC), eigenvector centrality (EC), local average connectivity-based method (LAC), and network centrality (NC). The genes greater than the median of each topological index were regarded as core targets.
Component-target network
The network between active components and core targets was visualized by Cytoscape, from which the important compounds were obtained according to the degree value.
Enrichment analysis
With p value < 0.05 and κ set to 0.4, under the conditions of Homo sapiens, the ClueGO plug-in of Cytoscape was applied for Gene Ontology (GO) and Kyoto Encyclopedia of Genes and Genomes (KEGG) enrichment analysis of the core targets.
Molecular docking
The 2D structures of important compounds were obtained from PubChem (https://pubchem.ncbi.nlm.nih.gov/), and 3D chemical structures were created by ChemBioDraw software. Protein structures were searched from PDB (https://www.rcsb.org/) according to UniProt ID. Water molecules and ligands of protein were removed by Discovery Studio. AutoDock Vina software was used for molecular docking with the binding site of the protein’s own ligand as the active pocket.
Animal model of I/R
Male ApoE-/- mice weighing 20 ± 2 g were obtained from Guangdong Medical Experimental Animal Center. The animals were housed in an SPF environment with a temperature of 22–25 °C, relative humidity of 60%, and 12 h light/dark cycle, with free access to adequate water and food. All procedures (including the mouse euthanasia) were performed in compliance with the regulations of the Second Affiliated Hospital of Guangzhou University of Chinese Medicine institutional animal care (Ethical number: 2016026) and conducted according to the AAALAC and IACUC guidelines (Ingham et al. Citation2000).
The operations were completed in the Laboratory Animal Center of the Second Affiliated Hospital of Guangzhou University of Traditional Chinese Medicine. Mice were randomly divided into 3 groups (n = 6): Sham group, I/R group, and TGC group (1 g/kg/d). During I/R surgery, the left anterior descending (LAD) coronary artery was ligated with 8–0 nylon suture. The ST segment elevation on the electrocardiogram and the color change of myocardial tissue indicated that myocardial ischemia was successfully established. Sutures were released after 45 min to induce 24 h of reperfusion. Mice in sham group underwent similar surgery without LAD coronary artery occlusion. All mice were given intragastric administration 5 days before surgery. The TGC group was administered TGC (Lot number: 161201, obtained from Guangdong Provincial Hospital of Chinese Medicine), while the sham group and the I/R group were administered the same volume of normal saline.
Echocardiography
The mice were anesthetized with 2% isoflurane (VETEASY, China), and heart function was assessed by transthoracic echocardiography (Vevo 2100, Canada). After measurement, left ventricular ejection fraction (LVEF) and fractional shortening (FS) were recorded.
Evans blue-TTC staining
After echocardiography was completed, the infarct size of the heart was determined by Evans blue-TTC staining. Briefly, the LAD coronary artery was sutured, and Evans blue solution (Meilunbio, China) was injected into heart vessels through the aorta. The heart was quickly removed and snap-frozen at −20 °C for 30 min, and then was cut into 1 mm thick slices and placed into TTC solution (Sigma-Aldrich, USA) at 37 °C for 15 min. The sections were fixed with a 4% paraformaldehyde solution. Image-Pro Plus software was devoted to assess infarct size (IA), area at risk (AAR), and total left ventricular area (LV), from which IA/AAR and IA/LV were calculated.
Cell model of hypoxia/reoxygenation (H/R)
HCAECs from BeNa Culture Collection, China, were cultured in DMEM (Gibco, China) supplemented with 10% FBS (HyClone, USA) and 1% penicillin/streptomycin in a humidified atmosphere containing 5% CO2/95% air at 37 °C. Before the experiment, the cells were regularly subcultured until they reached 80% confluence.
Cells were then divided into a control group, H/R group, and TGC group. Cells in H/R group were cultured in glucose-free medium with hypoxia (94% N2, 5% CO2, 1% O2) for 3 h and then transferred to 10% fetal bovine serum (FBS, HyClone, USA) medium in a normoxic incubator for 1h. Cell in TGC group was treated with 100 μg/mL TGC solution for 7 h before oxygen-glucose deprivation (OGD). Cells in the control group were cultured under normal conditions.
Hoechst 33258 staining and immunofluorescence
After the medium was discarded, the cells were washed with PBS (HyClone USA) 2 times and rapidly immobilized with 4% paraformaldehyde. The Hoechst 33258 solution (Beyotime, China) was then used to stain the cells for 15 min at room temperature. The images were observed under a fluorescence microscope (Nikon, China).
When performing immunofluorescence, the cell washing and immobilization steps were similar to those in Hoechst 33258 staining. Then, the cells were permeabilized in 0.5% Triton X-100 for 5 min and sealed in 5% BSA for 2 h. After being incubated with LC3 primary antibody (CST, USA) at 4 °C overnight, the cells were treated with fluorescently labeled secondary antibody (Invitrogen, USA) and DAPI (BOSTER, China) at room temperature. The images were observed under a fluorescence microscope, and the expression level was analyzed based on the fluorescence intensity.
Western blot
After H/R, the cells were dissolved in RIPA buffer containing protease and phosphoprotein inhibitors. A BCA kit (Thermo Fisher Scientific, USA) was used to detect the protein content. The protein samples were then separated by sodium dodecyl sulfate-polyacrylamide gel electrophoresis and transferred to a polyvinylidene fluoride (PVDF) membrane. After blocking with 5% bovine serum albumin, the PVDF membrane was incubated with primary antibody at 4 °C overnight and with secondary antibody (CST, USA) at room temperature for 1 h. The primary antibodies were GAPDH (CST, USA), GSK-3beta (CST, USA), P-GSK-3β (CST, USA), mTOR (CST, USA), P-mTOR (CST, USA), Beclin1 (CST, USA), and p62 (CST, USA). The band development was performed with an ECL kit (Millipore, USA) and the results were analyzed by Image J software.
Statistical analysis
All data were expressed as the mean ± SD and statistically analyzed by GraphPad Prism 8 software. Statistical significance analysis was performed using one-way ANOVA. The difference was considered statistically significant at p < 0.05.
Results
Identification of active compounds and candidate targets
Based on TCMSP, TCMID, and BATMAN, 91 active compounds met the screening conditions. A total of 1143 targets of the active compounds were searched from TCMSP, Swiss TargetPrediction, and STITCH. 1302 MIRI-related targets were identified according to GeneCards, OMIM, and TTD. A total of 393 overlapping genes both in MIRI and TGC were recognized as candidate targets. By calculating the median of topological indexes, 95 core targets were obtained, including mTOR, GSK-3β, AKT1, PIK3CA, and PIK3R1 ().
Component-target network
In the network (), 90 components in TGC were correlated with the core targets. There are eight active components with degree >20, which are considered as important compounds, including quercetin, luteolin, tanshinone IIA, kaempferol, and bifendate.
Enrichment analysis
GO enrichment analysis () indicated that the targets were mainly enriched in positive regulation of phosphorylation, positive regulation of kinase activity, angiogenesis, positive regulation of protein transport, and negative regulation of apoptotic signaling pathway. The KEGG results () further suggested that TGC in the treatment of MIRI might be related to the PI3K − AKT signaling pathway and mTOR signaling pathway, which were crucial in autophagy and apoptosis.
Molecular docking of compounds with proteins in the mTOR pathway
KEGG analysis revealed that TGC might improve MIRI by regulating autophagy-related proteins in the mTOR pathway. To further verify the interaction of TGC with targets in this pathway (), we performed the docking of important compounds with the following proteins: GSK-3β (PDB: 1H8F), mTOR (PDB: 4JSV), Beclin1 (PDB: 6HOJ), and LC3 (PDB: 7ELG). The value of affinity reflected the strength of docking. As shown in , the affinity values were all <-5 kcal/mol, suggesting that TGC may interact with autophagy-related proteins in the mTOR pathway to improve MIRI.
Figure 5. The interaction of important components with GSK-3β, mTOR, Beclin1, LC3. (A) In the active pocket of GSK-3β, Kaempferol formed hydrogen bonds with GLY A:79 and GLU A:80, and there was an alkyl interaction with VAL A:82. (B) Quercetin interacts with MET B:2345, TRP B:2239, and LEU B:2185 in mTOR to form π-sulfur, π-π stacking, and π-alkyl, respectively. (C) Docking with Beclin 1, Tanshinone IIA forms π-cation, π-σ and π-alkyl interactions with ARG C:65, TYR A:61, and PHE B:62, respectively. (D) In addition to hydrogen bonding with ASP A:52, Luteolin also forms π-cation interaction with ARG A:14 and π -alkyl interaction with VAL A:50 in the binding of LC3.
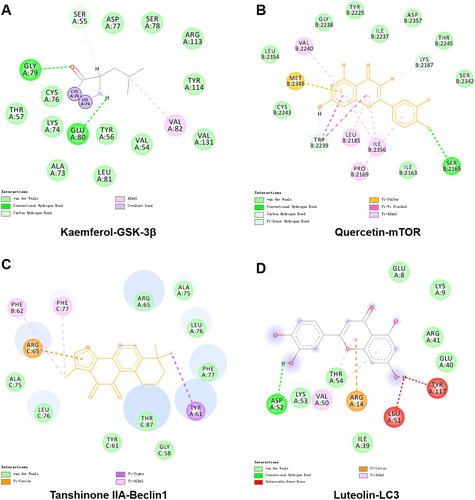
Table 1. Docking score of important compounds with GSK-3β, mTOR, Beclin1, and LC3.
Effect of TGC on MIRI heart
Echocardiography showed abnormal ventricular wall motion in mice after surgery (). Compared with sham group, LVEF and FS in I/R group decreased significantly (p < 0.05). After TGC treatment, these two parameters were significantly increased (p < 0.05), suggesting that TGC was beneficial to improve cardiac function after MIRI ().
Figure 6. Results of each group in echocardiography and Evans blue/TTC staining. (A) Representative images of M-mode echocardiography after MIRI. (B) LVEF and FS measured according to echocardiography (n = 6). (C) Representative images of Evans blue/TTC staining. (D) The area of myocardial infarction was measured according to Evans blue/TTC staining (n = 3). *p < 0.05, **p < 0.01, ***p < 0.001.
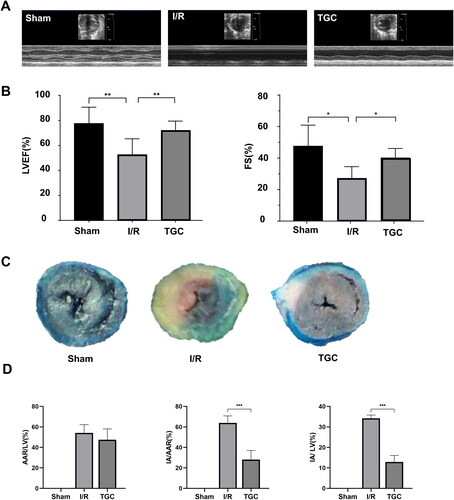
In Evans Blue/TTC staining (), there was no significant difference in AAR/LV between TGC group and I/R group. IA/LV and IA/ARR in the TGC group were significantly lower than those in I/R group (p < 0.05), indicating that TGC could reduce the proportion of myocardial infarction area ().
TGC reduce the apoptosis of H/R cells
In Hoechst 33258 staining, bright blue nuclei represented apoptosis. The ratio of bright blue nuclei in TGC group was significantly lower than that in H/R group, indicating that the apoptosis rate of cells decreased after TGC treatment (). These results suggested that TGC inhibit the apoptosis of coronary endothelial cells induced by H/R.
TGC upregulates LC3 expression in H/R cells
To clarify the role of autophagic proteins, we performed immunofluorescence detection of LC3. As shown in , the level of LC3 (red fluorescence) in TGC group was significantly higher than that in H/R group, manifesting that TGC may inhibit apoptosis by enhancing autophagy.
TGC inhibits apoptosis by regulating autophagy-related proteins in the mTOR pathway
As shown in western blot (), after treatment with TGC, the levels of p-GSK-3 β and Beclin1 increased, while p-mTOR and p62 were decreased compared with those in H/R group (p < 0.05). It was further suggested that TGC inhibited apoptosis of coronary endothelial cells by regulating autophagy-related proteins in the mTOR pathway.
Figure 9. The expression of autophagy-related proteins in the mTOR pathway. (A) TGC decreased the level of p-mTOR and increased p-GSK-3β, which was assessed by Western blot. (B) TGC reduced the level of p62 and increased Beclin1, which was assessed by Western blot. N = 3. *p < 0.05, **p < 0.01, ***p < 0.001.
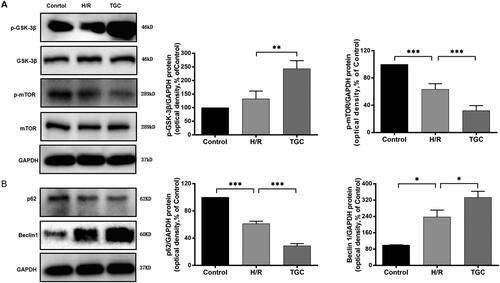
Discussion
Network pharmacology analysis suggested that the important active ingredients in TGC, including quercetin, luteolin, tanshinone IIA, kaempferol and bifendate improved MIRI through mTOR pathway and autophagy. Experiment has further determined that TGC reduced the area of myocardial infarction and increased the left ventricular ejection fraction, by regulating autophagy-related proteins in the mTOR pathway to inhibit cell apoptosis.
Previous studies have shown that the level of autophagy affected the repair of ischemia-reperfusion injury (Zhang et al. Citation2019c). Autophagy enhances the survival of cardiomyocytes after I/R injury by removing damaged mitochondria and other cellular contents (Khuanjing et al. Citation2021). In the case of hypoxia or lack of nutrient substrates, autophagy provides energy by degrading impaired organelles, thereby exerting a cytoprotective effect (Varshavsky Citation2017; Yu et al. Citation2018; Yang et al. Citation2019). Numerous cardiovascular diseases are associated with endothelial dysfunction, and regulating autophagy may be a novel frontier in the treatment of these conditions (Schaaf et al. Citation2019; Hughes et al. Citation2020; Shan et al. Citation2021; Mameli et al. Citation2022).
Autophagy initiation is regulated by multiple signaling pathways (Dikic and Elazar Citation2018; Levine and Kroemer Citation2019). mTOR is a relatively conserved serine/threonine-protein kinase that regulates various biological functions, including cell division, differentiation, apoptosis, and autophagy (Wang and Proud Citation2006; Saxton and Sabatini Citation2017; El Hiani et al. Citation2019). Under oxidative stress, mTOR promotes tissue repair by regulating the process of autophagy-related proteins, such as beclin1, p62, and LC3 (Tang et al. Citation2017; Zhou et al. Citation2018; Al-Bari and Xu Citation2020). During I/R, the mTOR signaling pathway is inhibited, while autophagy is activated, thus increasing the level of Beclin1 (Dai et al. Citation2017). In addition, Beclin1 knockout prevents the production of autophagosomes, promoting apoptosis and tissue damage (Dai et al. Citation2017).
As a conserved serine/threonine kinase, GSK-3β exerts biological effects by controlling mTOR (Urbanska et al. Citation2018). By regulating the expression of GSK-3β and mTOR, autophagy signals are induced to decrease myocardial infarct size and promote cardiac recovery in MIRI mouse models (Li et al. Citation2014).
The important active components of TGC have a role in protecting the heart by regulating autophagy. Quercetin alleviates oxidative stress-induced damage and restores mitochondrial functions by enhancing autophagy (Daw and Law Citation2021). Moreover, luteolin improves cardiac function, by upregulating autophagy and inhibiting inflammation (Hu et al. Citation2016; Wu et al. Citation2020). Additionally, tanshinone IIA induces autophagy by regulating the mTOR signaling pathway to reduce myocardial cell apoptosis (Zhang et al. Citation2019b). Kaempferol upregulates GSK-3β to inhibit the mTOR pathway, increasing LC3-II and Beclin 1, for alleviating ox-LDL-induced apoptosis (Che et al. Citation2017; Hoang et al. Citation2019).
Molecular docking results indicated that the important compound had a high affinity for the target, suggesting that TGC may protect the heart through autophagy proteins associated with the mTOR pathway. Based on autophagy, exploring the mechanism of TGC in the treatment of MIRI could further clarify the drug target, which was conducive to the research and development of novel medicine for coronary artery disease.
Conclusions
Based on network pharmacology and experimental studies, we found that TGC exerted a cardioprotective effect against MIRI, by upregulating autophagy-related proteins through mTOR pathway (). These data suggested that TGC may be a therapeutic option for MIRI. However, the present study had certain limitations. Insufficient databases were utilized in network pharmacology analysis, resulting in omissions in data collection. In addition, randomized controlled trials are needed for further validation for clinical application.
Author contributions
JL and LC jointly designed and conducted this study. HC performed the experimental data analysis and interpretation of the results. HC and HY assisted with experiments such as I/R surgury and echocardiography detection. PL, FL, and LM revised and improved the text of the manuscript. QC and LW provided technical guidance and supervised the study.
Consent for publication
All authors agreed to publish.
Acknowledgement
We are very grateful to Shihui Zhang for her help in this study.
Disclosure statement of interest
All authors declared that there were no conflicts of interest.
Data availability statement
The raw data of this study were available upon request.
Additional information
Funding
References
- Al-Bari MAA, Xu P. 2020. Molecular regulation of autophagy machinery by mTOR-dependent and -independent pathways. Ann NY Acad Sci. 1467(1):3–20.
- Che J, Liang B, Zhang Y, Wang Y, Tang J, Shi G. 2017. Kaempferol alleviates ox-LDL-induced apoptosis by up-regulation of autophagy via inhibiting pi3k/AKT/mTOR pathway in human endothelial cells. Cardiovasc Pathol. 31:57–62.
- Dai S, Xu Q, Liu S, Yu B, Liu J, Tang J. 2017. Role of autophagy and its signaling pathways in ischemia/reperfusion injury. Am J Transl Res. 9(10):4470–4480.
- Davidson SM, Ferdinandy P, Andreadou I, Botker HE, Heusch G, Ibanez B, Ovize M, Schulz R, Yellon DM, Hausenloy DJ, et al. 2019. Multitarget strategies to reduce myocardial ischemia/reperfusion injury: JACC review topic of the week. J Am Coll Cardiol. 73(1):89–99.
- Daw S, Law S. 2021. Quercetin induces autophagy in myelodysplastic bone marrow including hematopoietic stem/progenitor compartment. Environ Toxicol. 36(2):149–167.
- Dikic I, Elazar Z. 2018. Mechanism and medical implications of mammalian autophagy. Nat Rev Mol Cell Biol. 19(6):349–364.
- El Hiani Y, Egom EE, Dong XP. 2019. mTOR signalling: jack-of-all-trades (1). Biochem Cell Biol. 97(1):58–67.
- Hoang MH, Jia Y, Lee JH, Kim Y, Lee SJ. 2019. Kaempferol reduces hepatic triglyceride accumulation by inhibiting AKT. J Food Biochem. 43(11):e13034.
- Hu JQ, Man WR, Shen M, Zhang MM, Lin J, Wang TT, Duan Y, Li CY, Zhang RQ, Gao EH, et al. 2016. Luteolin alleviates post-infarction cardiac dysfunction by up-regulating autophagy through mst1 inhibition. J Cell Mol Med. 20(1):147–156.
- Hughes WE, Beyer AM, Gutterman DD. 2020. Vascular autophagy in health and disease. Basic Res Cardiol. 115(4):41.
- Ingham KM, Goldberg JA, Klein HJ, Johnson RG, Kastello MD. 2000. A novel approach for assessing the quality and effectiveness of IACUC oversight in investigator compliance. Contemp Top Lab Anim Sci. 39(1):28–31.
- Khuanjing T, Palee S, Kerdphoo S, Jaiwongkam T, Anomasiri A, Chattipakorn SC, Chattipakorn N. 2021. Donepezil attenuated cardiac ischemia/reperfusion injury through balancing mitochondrial dynamics, mitophagy, and autophagy. Transl Res. 230:82–97.
- Kibble M, Saarinen N, Tang J, Wennerberg K, Makela S, Aittokallio T. 2015. Network pharmacology applications to map the unexplored target space and therapeutic potential of natural products. Nat Prod Rep. 32(8):1249–1266.
- Kumar J, O'Connor CT, Kumar R, Arnous SK, Kiernan TJ. 2019. Coronary no-reflow in the modern era: a review of advances in diagnostic techniques and contemporary management. Expert Rev Cardiovasc Ther. 17(8):605–623.
- Lee ZV, Hanif B. 2018. Historical perspectives on management of acute myocardial infarction. In: Watson TJ, Ong PJL, Tcheng JE, editors. Primary angioplasty: a practical guide. Singapore: Springer. Chapter 1:1–13.
- Levine B, Kroemer G. 2019. Biological functions of autophagy genes: a disease perspective. Cell. 176(1-2):11–42.
- Li J, Rohailla S, Gelber N, Rutka J, Sabah N, Gladstone RA, Wei C, Hu P, Kharbanda RK, Redington AN. 2014. Microrna-144 is a circulating effector of remote ischemic preconditioning. Basic Res Cardiol. 109(5):415–423.
- Ma S, Ma J, Zhou Y, Guo L, Bai J, Zhang M. 2019. Tongguan capsule derived-herb ameliorates remodeling at infarcted border zone and reduces ventricular arrhythmias in rats after myocardial infarction. Biomed Pharmacother. 120:109514.
- Mameli E, Martello A, Caporali A. 2022. Autophagy at the interface of endothelial cell homeostasis and vascular disease. Febs J. 289(11):2976–2991.
- Mao S, Ouyang W, Zhou Y, Zeng R, Zhao X, Chen Q, Zhang M, Hinek A. 2020. Addition of Chinese herbal remedy, Tongguan capsules, to the standard treatment in patients with myocardial infarction improve the ventricular reperfusion and remodeling: proteomic analysis of possible signaling pathways. J Ethnopharmacol. 257:112794.
- Muhammad J, Khan A, Ali A, Fang L, Yanjing W, Xu Q, Wei DQ. 2018. Network pharmacology: exploring the resources and methodologies. Curr Top Med Chem. 18(12):949–964.
- Pinzi L, Rastelli G. 2019. Molecular docking: shifting paradigms in drug discovery. Int J Mol Sci. 20(18):1–23.
- Saxton RA, Sabatini DM. 2017. mTOR signaling in growth, metabolism, and disease. Cell. 168(6):960–976.
- Schaaf MB, Houbaert D, Mece O, Agostinis P. 2019. Autophagy in endothelial cells and tumor angiogenesis. Cell Death Differ. 26(4):665–679.
- Shan R, Liu N, Yan Y, Liu B. 2021. Apoptosis, autophagy and atherosclerosis: relationships and the role of hsp27. Pharmacol Res. 166:105169.
- Shuai M, Peipei C, Ting L, Liheng G, Minzhou Z. 2018. Tongguan capsule mitigates post-myocardial infarction remodeling by promoting autophagy and inhibiting apoptosis: role of Sirt1. Front Physiol. 9:589.
- Tang Q, Zheng G, Feng Z, Chen Y, Lou Y, Wang C, Zhang X, Zhang Y, Xu H, Shang P, et al. 2017. Trehalose ameliorates oxidative stress-mediated mitochondrial dysfunction and er stress via selective autophagy stimulation and autophagic flux restoration in osteoarthritis development. Cell Death Dis. 8(10):e3081.
- Urbanska M, Gozdz A, Macias M, Cymerman IA, Liszewska E, Kondratiuk I, Devijver H, Lechat B, Van Leuven F, Jaworski J. 2018. Gsk3beta controls mTOR and prosurvival signaling in neurons. Mol Neurobiol. 55(7):6050–6062.
- Varshavsky A. 2017. The ubiquitin system, autophagy, and regulated protein degradation. Annu Rev Biochem. 86:123–128.
- Vogel B, Claessen BE, Arnold SV, Chan D, Cohen DJ, Giannitsis E, Gibson CM, Goto S, Katus HA, Kerneis M, et al. 2019. St-segment elevation myocardial infarction. Nat Rev Dis Primers. 5(1):39.
- Wang J, Toan S, Zhou H. 2020a. Mitochondrial quality control in cardiac microvascular ischemia-reperfusion injury: new insights into the mechanisms and therapeutic potentials. Pharmacol Res. 156:104771.
- Wang J, Toan S, Zhou H. 2020b. New insights into the role of mitochondria in cardiac microvascular ischemia/reperfusion injury. Angiogenesis. 23(3):299–314.
- Wang R, Wang M, Zhou J, Wu D, Ye J, Sun G, Sun X. 2020c. Saponins in Chinese herbal medicine exerts protection in myocardial ischemia-reperfusion injury: possible mechanism and target analysis. Front Pharmacol. 11:570867.
- Wang X, Proud CG. 2006. The mTOR pathway in the control of protein synthesis. Physiology (Bethesda). 21:362–369.
- Wu B, Song H, Fan M, You F, Zhang L, Luo J, Li J, Wang L, Li C, Yuan M. 2020. Luteolin attenuates sepsis induced myocardial injury by enhancing autophagy in mice. Int J Mol Med. 45(5):1477–1487.
- Xie YZ, Ma J, Yao GZ, Zou X. 2021. Relationship between programmed cell death and myocardial ischemia-reperfusion injury and new perspectives of traditional Chinese medicine intervention. Zhongguo Zhong Yao Za Zhi. 46(6):1345–1356.
- Yang J, Zhou R, Ma Z. 2019. Autophagy and energy metabolism. Adv Exp Med Biol. 1206:329–357.
- Yu L, Chen Y, Tooze SA. 2018. Autophagy pathway: cellular and molecular mechanisms. Autophagy. 14(2):207–215.
- Zhang R, Zhu X, Bai H, Ning K. 2019a. Network pharmacology databases for traditional Chinese medicine: review and assessment. Front Pharmacol. 10:123.
- Zhang X, Wang Q, Wang X, Chen X, Shao M, Zhang Q, Guo D, Wu Y, Li C, Wang W, et al. 2019b. Tanshinone IIa protects against heart failure post-myocardial infarction via ampks/mTOR-dependent autophagy pathway. Biomed Pharmacother. 112:108599.
- Zhang Y, Liu D, Hu H, Zhang P, Xie R, Cui W. 2019c. Hif-1alpha/bnip3 signaling pathway-induced-autophagy plays protective role during myocardial ischemia-reperfusion injury. Biomed Pharmacother. 120:109464.
- Zhou H, Toan S. 2020. Pathological roles of mitochondrial oxidative stress and mitochondrial dynamics in cardiac microvascular ischemia/reperfusion injury. Biomolecules. 10(1):21–85.
- Zhou M, Xu W, Wang J, Yan J, Shi Y, Zhang C, Ge W, Wu J, Du P, Chen Y. 2018. Boosting mTOR-dependent autophagy via upstream tlr4-myd88-mapk signalling and downstream NF-kappaB pathway quenches intestinal inflammation and oxidative stress injury. EBioMedicine. 35:345–360.
- Zhou YS, Mao S, Guo LH, Gao XY, Zou X, Zhang MZ. 2021. Effect of tongguan capsules (通冠胶囊) on restenosis after coronary stent implantation: study protocol for a randomized controlled trial. Chin J Integr Med. 27(1):16–23.